- 1College of Forest Resources and Environmental Science, Michigan Technological University, Houghton, MI, United States
- 2USDA Forest Service, Northern Research Station, Houghton, MI, United States
- 3Department of Chemical Engineering, Michigan Technological University, Houghton, MI, United States
- 4Department of Civil and Environmental, Geospatial Engineering, Michigan Technological University, Houghton, MI, United States
- 5USDA Forest Service, Northern Research Station, Rhinelander, WI, United States
Enhanced weathering of terrestrial rock material is a promising method for the removal of anthropogenic CO2 emissions from the atmosphere. Herein, we demonstrate that an ameliorated mining waste product can be effectively weathered in the soil environment when used as a soil amendment in conjunction with the cultivation of fast-growing willows (Salix matsudana Koidz. ⨯ S. alba L. “Austree”) in a pot study environment. Utilizing this locally sourced amendment minimizes emissions associated with grinding and transportation of enhanced weathering materials. Results showed that the willows were able to tolerate the relatively high metal concentrations of this amendment and sequester inorganic carbon (C) through the production of bicarbonate in soil solution. During the period of peak plant growth (10 weeks after planting), alkalinity measurements of soil solution from pots with willows and the addition of 25% by mass mine waste product indicated an additional 10 mg of inorganic C sequestration per liter of leached soil solution compared to unamended soils with willows. This represents 4.5 times the inorganic C sequestration rate of unamended soils. The addition of ameliorated mining waste also increased the pH of the soil solution by up to two units (pH of 6 in control vs. pH of 8 with the addition of 25% by mass mineral amendment). In addition to inorganic C sequestration, weathering of the ameliorated mining waste product may also provide base cations (such as calcium and magnesium) which could improve soil fertility. These results are encouraging for future investigation of ameliorated mine waste rock to sequester carbon and enhance the production of willows grown for ecosystem services and phytotechnologies.
1 Introduction
A large amount of atmospheric CO2 is sequestered annually by natural weathering of silicate minerals (Mackenzie and Lerman, 2006; Hartmann and Kempe, 2008). This sequestration occurs when bicarbonate and carbonates are formed through the reaction of CO2 with base cations from silicates found in many rock formations (Seifritz, 1990; Lackner et al., 1995; Beerling et al., 2020). This natural weathering process can be sped up by finely grinding silicate rocks to increase surface area, thereby increasing the rate of the weathering reaction. This process is referred to as “enhanced weathering” and the dissolved bicarbonates and carbonate minerals formed through this process can sequester C on a long time scale (Lackner et al., 1995; Schuiling and Krijgsman, 2006; Hartmann et al., 2013; Renforth et al., 2015; Renforth et al., 2015; Strefler et al., 2018; Haque et al., 2019; Haque et al., 2020b).
Dissolved bicarbonate derived from enhanced weathering can leach into groundwater and subsequently surrounding water bodies. These bicarbonates can help to balance the acidification caused by CO2 absorption (Zeebe, 2012; Renforth et al., 2015; Taylor et al., 2015; Kanzaki et al., 2023). Acidification associated with rising atmospheric CO2 levels is an emerging issue in the Laurentian Great Lakes of eastern North America (Great Lakes) (Rowe et al., 2020), and therefore employment of enhanced weathering in management plans in the Great Lakes regions may have the potential to combat lake acidification. This represents a unique region-specific co-benefit of enhanced weathering activities.
The Upper Peninsula (UP) of Michigan, United States, a geographic region bordering Lakes Superior, Huron and Michigan, is burdened with a large amount of mining waste, often referred to as mine tailings, leftover from the extraction of copper (Cu) and iron (Fe) ores. These tailings are typically alkaline with high base cation concentrations and contain silicate minerals similar to the minerals and lithologies used in previous enhanced weathering experiments (e.g., Beerling et al., 2018). Their local proximity could partially avoid emissions associated with transportation (Moosdorf et al., 2014). Much of Michigan’s UP consists of infertile acidic soils (Bockheim, 2021), and amending these soils with the alkaline mine tailings may raise soil pH while simultaneously introducing base cations essential for tree growth (Goll et al., 2021; Vicca et al., 2022).
Employing enhanced weathering in the soils of Michigan’s UP may offer an organic C sequestration benefit in addition to the inorganic C sequestration associated with dissolved bicarbonate production (Goll et al., 2021; Vicca et al., 2022). Short rotation woody crops (SRWCs) such as willows (Salix species and their hybrids) are grown in the UP as feedstocks for bioenergy, biofuels, and bioproducts, as well as phytoremediation and associated phytotechnologies (Keoleian and Volk, 2005; Wang and MacFarlane, 2012; Ali et al., 2013; Miller, 2018; Haque et al., 2020a; Suhrhoff, 2022; Vinhal et al., 2022). Willows are ideal for these applications due to their rapid growth and tolerance of widely ranging soil conditions (Kockic et al., 2007; Volk et al., 2016), and have been suggested as useful species in the context of combining enhanced weathering, phytoremediation and bioenergy carbon capture and storage (Suhrhoff, 2022). Soil amendment with silicate-rich mine tailings could be a promising way to increase willow biomass production while simultaneously sequestering CO2 through enhanced weathering. The presence of plants also substantially increases mineral weathering rates (Lucas, 2001).
Willows are good candidates for growth in soils amended with mine tailings because they have a high tolerance for iron (Fe), nickel (Ni), and other heavy metals and could potentially serve to remediate the tailings (Korzeniowska and Stanislawska-Glubiak, 2018; Pilipović et al., 2019; Suhrhoff, 2022). Many of the mafic and ultramafic rocks used for enhanced weathering contain high concentrations of various heavy metals (Haque et al., 2020a). Preventing heavy metal contamination from enhanced weathering is essential to the feasibility of this technology, and the use of SRWCs can help minimize heavy metal accumulation in soils (Suhrhoff, 2022). Nickel is an environmental contaminant toxic to many plants, but there is a potential for dispersion of mine tailings onto willow biomass production systems to allow for phytomining of heavy metals (Mohsin et al., 2022). Phytomining involves a process whereby certain plants that hyperaccumulate heavy metals (such as Ni) are grown in mining waste or other substrates containing these toxic metals. These plants are then burned, and the leftover ash is leached to reclaim the metals accumulated during their growth (van der Ent et al., 2015). Metals such as Ni can accumulate at high rates in willow tissues (Jama-Rodzeńska and Nowak, 2012; Salam et al., 2016) and can be useful in efforts to remediate toxic mining sites.
In addition to phytoremediation potential (Mirck et al., 2005; Vinhal et al., 2022), willows are also burned to produce bioenergy (Volk et al., 2016; Montes et al., 2021), which may have the potential to offset some emissions from fossil fuel use during comminution and transport of mine tailings. Willows are grown for an establishment year, coppiced (i.e., aboveground biomass is removed from the original root system during the dormant season), and then coppiced again on 3-years intervals for seven rotations (i.e., the final root system is 22 years old) (Sleight and Volk, 2016). Although willows are able to grow quickly in the poor-quality soils of this region, soil amendments may create more productive soils and improve growth rates, thus raising the amount of carbon that is sequestered.
To address the need for such sustainable biomass production systems, this work evaluated the use of ameliorated mine waste product, a potentially toxic mining byproduct prevalent in the region, as a soil amendment to support bioenergy crop production and sequester carbon through enhanced weathering (Bullock et al., 2021; Khalidy and Santos, 2021). Our primary means of assessing the suitability of the mineral amendment were examining changes in soil pH and bicarbonate production following addition of the mineral material. Plant biomass production and Ni content were also examined to provide baseline information on the effect of mineral additions on plant health and to gain insight into whether phytomining of mining wastes might be a possibility in this region.
2 Materials and methods
2.1 Materials
Soil was collected to 20 cm of depth (Ap horizon) at the Michigan State University Upper Peninsula Forestry Innovation Center (45°45′26.9″N 87°11′00.8″W), just after plowing and application of glyphosate in preparation for planting of willow in June 2022. The soils are classified as Inceptic Hapludalfs, which are representative of the relatively nutrient poor soils employed for SRWCs. Soils were dried at 60°C and sieved through 2 mm mesh.
Mineral amendments used in the experiment were composed of locally sourced mining waste material. Figure 1 shows the elemental and mineralogical compositions of the mineral amendment, which were obtained and processed from local mine waste products. The waste products contain a high percentage of sulfur-bearing minerals, which had to be lowered prior to amending soils (hence, “ameliorated”). The mine materials were beneficiated using the froth flotation method (Xing et al., 2017; Mesa and Brito-Parada, 2019) to produce non-sulfide mineral feed materials. Soil and mineral amendments were characterized according to standard methods.
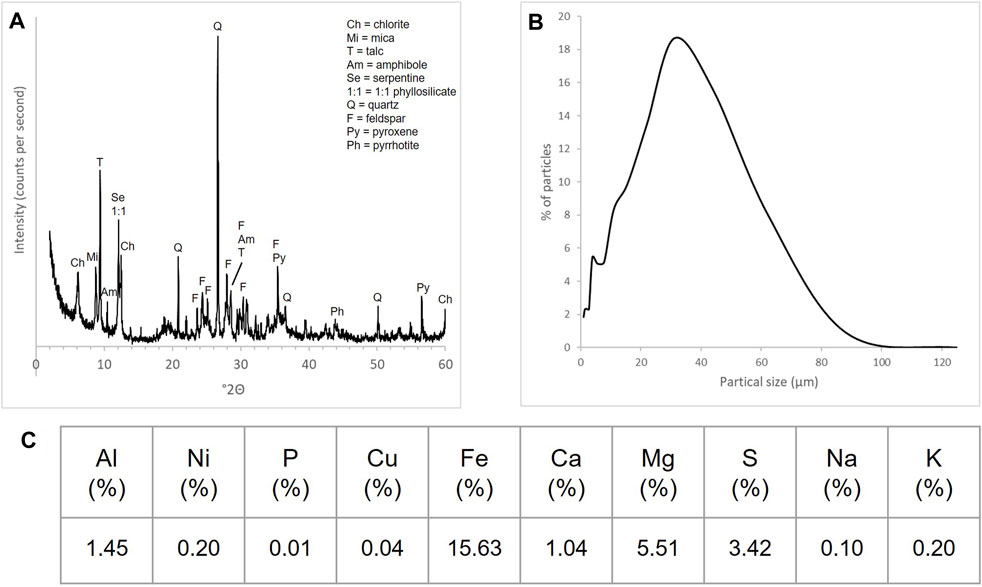
Figure 1. Characterization of mineral amendment derived from mine waste: (A) X-ray diffraction (XRD) spectra of the silicate-rich ameliorated mine tailings, (B) particle size distribution (PSD), and (C) elemental composition (% by mass) of the material as determined by ICP-OES.
The soil and mineral amendment were characterized following standard protocols for pH, water holding capacity (field capacity) and cation exchange capacity. The pH was measured using a 1:1 mixture of soil or mineral amendment to DI water using a pH meter (Model 220, Denver Instruments, Arvada, Colorado, United States) after the mixture had been allowed to sit for 15 min (Soil Survey Staff, 2014). Particle size was measured using a hydrometer and sedimentation columns (Gee and Bauder, 1986). Field water holding capacity of the soil and the mineral amendment was measured using a gravimetric water content test. A 25 g sample of soil was placed in a funnel over a graduated cylinder with wetted filter paper and 25 mL of DI water was added. These were allowed to drain until no more water was dripping from them. The percent of water that did not drain from the soil was used to calculate the field capacity in ml g−1 soil. Cation exchange capacity was measured by ammonium displacement by 1 M KCl (Shuman et al., 2008; Soil Survey Staff, 2014). The elemental composition of the mineral amendment was determined using inductively coupled plasma - optical emission spectroscopy (ICP-OES, model Optima 7000DV, PerkinElmer, Shelton, CT, United States). ICP measurements were conducted at the Lab for Ecological Analyses in Forests (LEAF) housed in the College of Forest Resources and Environmental Science at Michigan Technological University. The elements measured included calcium (Ca), iron (Fe), potassium (K), magnesium (Mg), sodium (Na) (Perkin Elmer Instrument Calibration Standard 3), and aluminum (Al), nickel (Ni) and copper (Cu) (calibration standard by Inorganic ventures CCS-6). Single element standards were used for sulfur (S) and phosphorus (P) (Spex CertiPrep). Quality control standards were within 10% of the known value. Prior to analysis, the mineral material was first digested in a two-acid digestion method (a mixture of hydrochloric acid and nitric acid) using a standard microwave digestion method (EPA method 3052).
The willow clone tested in this experiment was S. matsudana Koidz. ⨯ S. alba L. “Austree” (family: Salicaceae). Dormant, unrooted cuttings of −20 cm length were purchased from a commercial nursery (CZ Grain Store, Iowa, United States). This variety is fast growing and hardy, able to grow 1.83–3.05 m yr−1, and can be coppiced annually.
All treatments were irrigated with a mixture designed to simulate rainwater. The composition of the mixture was as follows for 1 L of concentrated solution: 88 mg NaCl, 282.5 mg Ca(NO3−)2, 223.1 mg Mg(SO4)*(H2O)7, 62.8 mg KNO3, 845.8 mg (NH4)2SO4, 16 mg NH4Cl, 177 mg (NH4)2CO3, and 0.59 mL HNO3. Each of these chemicals was added into a 1 L container of deionized water and mixed thoroughly. The pH of this solution was adjusted to 4.8–5.5 by adding additional nitric acid and refrigerated at 4°C. A 1 mL aliquot of this concentrated solution was diluted in 1 L of deionized water to form the artificial rainwater used for irrigation. Concentrations of analytes in final artificial rainwater solutions were as follows: 0.03 ppm Na, 0.07 ppm Ca, 0.02 ppm Mg, 0.02 ppm K, 0.70 SO4, 0.30 ppm NH4, 0.21 ppm NO3, 0.06 ppm Cl.
2.2 Experimental setup
The mineral amendment material utilized in the current study was intended to have an acid neutralizing capacity, thereby acting both as a C sequestration tool and a liming substitute. Liming rate for the soil was determined according to Warncke et al. (2010), with the mass of mineral amendment used adjusted according to base cation content of the tailings material (Beerling et al., 2018). The mass of mineral amendment used for the 1% by mass addition treatment was roughly three times that of the equivalent amount of pure calcium carbonate needed to achieve the targeted base cation addition. Larger additions of mineral amendment (4% and 25%) were included to investigate possible toxicity effects of Ni on willows and to examine how mineral weathering might vary based on concentration in the soil matrix.
The experimental design consisted of four levels of mineral amendment addition (0%, 1%, 4%, 25% by mass) and the presence or absence of trees. There were seven treatments total with twelve replicates each for a total of 84 experimental units. The treatments were as follows: 1) a control containing unamended soil (0%) (approx. 1 kg soil), 2) a mixture of soil and a low concentration of mineral amendment (1%) (approx 1 kg soil, 12 g amendment), 3) a mixture of soil, a low concentration of mineral amendment (1%), and willows (approx 1 kg soil, 12 g amendment), 4) a mixture of soil and a high concentration of mineral amendment (4%) (approx 1 kg soil, 40 g amendment), 5) a mixture of soil, a high concentration of mineral amendment (4%), and willows (approx 1 kg soil, 40 g amendment), 6) a mixture of soil and an approximated 20-year field amendment treatment (25%) (approx. 765 g soil, 235 g amendment), and 7) a mixture of soil, the 20-years field amendment (25%), and willows (approx. 765 g soil, 235 g amendment) (Supplementary Table S1). Materials were weighed into 1 L plastic pots (6.9 ⨯ 35.6 cm Lightweight Deepot Cell, Stuewe & Sons, Inc., Tangent, Oregon, United States) with vented bottoms. The bottoms of the pots were covered with fine mesh netting to prevent loss of particulate material while allowing excess moisture to drain through. Willow cuttings were soaked in tap water for 48 h to promote root nodule development and then planted in prepared pots approximately 10.16 cm deep. All pots (with and without trees) were transferred to a growth chamber (Model CMP5090, Conviron, Winnipeg, Manitoba, Canada) for the duration of the experiment. Samples were incubated at 50% water-holding capacity (240 mL of water was added to the dry soil to achieve this, or the equivalent of −6.24 cm of precipitation) for a period of 108 days under controlled conditions, rotating the pot locations within the growth chamber. Growth conditions were as follows: 60% relative humidity, 21°C, 13-h days and 9-h nights with 1-h transitions between light and dark to simulate dusk and dawn. Soil moisture was maintained through daily application of artificial water, and water contents were checked by mass and adjusted weekly to ensure consistent moisture conditions among pots and treatments over time. For context, the potential field site where soils were harvested received 64.8 cm of precipitation in 2022 (Michigan Automated Weather Network, 2022), the equivalent of −5 times that of the soil water holding capacity.
2.3 Leachate chemistry
At weeks 5, 10, and 15 of the experiment the pots were irrigated with water in excess of the soil’s field capacity to produce 120 mL of fluid (hereafter referred to as leachate) from the bottom of the pot. A −60 mL subsample of the leachate was measured for pH using a pH probe (Model 220, Denver Instruments, Arvada, Colorado, United States), then filtered through nylon membrane filters with pore size of 0.45 μm (Product #7404-004, Whatman, Little Chalfont, Buckinghamshire, United Kingdom) and stored at 4°C for further analysis. A second −50 mL subsample of the leachate was weighed and measured for alkalinity within an hour of leachate collection (Hach Method 8221, Hach Company, Loveland, Colorado, United States).
Leachate chemistry was further evaluated through the measurement of dissolved compounds of interest. Both Iron and sulfur (S) were measured to evaluate the dissolution of residual iron sulfide from mineral amendment material. Nitrate (NO3−), sulfate (SO4−2), and chloride (Cl−) were measured to assess nutrient dynamics, and Cl− concentration was used to assess ion accumulation from the artificial rainwater mixture (ICS-2000 ion chromatograph with an IonPac AS11 separator column (Dionex Corporation, Bannockburn, IL, United States)). Instrument calibration was performed using a proprietary seven-anion standard (Dionex CO.) at four levels (0.1, 0.25, 1.00, and 5.00 mg L−1). As such, our accuracy is within 0.1 mg L−1 and our purported precision is 2%. Dissolved Fe was measured using the phenanthroline spectrophotometric method (Hach Method 8146, Hach Company, Loveland, Colorado, United States), which was scaled to microplates following Veverica et al. (2016).
Elemental composition of leachate solutions was determined using inductively coupled plasma - optical emission spectroscopy (ICP-OES, model Optima 7000DV, PerkinElmer, Shelton, CT, United States). Only two of the twelve replicates from each treatment were measured for leachate elemental composition by ICP-OES (see methods above for instrumental details).
2.4 Post-incubation harvest and characterization
At 15 weeks after planting the trees were removed from the soil matrix and weighed to assess biomass accumulation. The roots and shoots (stems + leaves) were separated from the original cuttings, and all biomass portions were dried separately at 60°C until a constant mass was achieved. Soil and mineral amendment mixtures were dried at 60°C. Plant shoots were combusted at 500°C for 8 h to produce ash that was subsequently measured by ICP-OES for elemental composition following methods outlined above.
Organic matter content of the incubated soils and mixtures was measured through loss on ignition. A 5 g subsample was taken from each replicate and placed into a muffle furnace in ceramic crucibles at 500°C for 8 h to burn off organic matter. These samples were weighed before and after to determine the mass loss and thereby estimate percent organic matter content for each sample (n = 12 for each treatment). Four samples were taken randomly from each treatment for elemental analysis (n = 4 for each treatment) (4010 Elemental Analyzer, Costech, Valencia, California, United States).
2.5 Statistical analysis
Tests for significance were performed using JMP®, Version 17 (SAS Institute Inc., 2023). ANOVA tests followed by post hoc Tukey’s HSD were performed on leachate and biomass data to test differences across all treatment and week combinations. Letter groupings in figures and tables were determined using Tukey’s HSD. Figures were created using the package ggplot2 (Wickham, 2016) in R statistical software (R core team, 2021).
2.6 Carbon dioxide removal estimates
Carbon sequestration determination from alkalinity measurements followed the recommendation of Hach Co., wherein alkalinity is converted to equivalent mg of CaCO3 based on molecular mass. Carbon sequestration potential on a per hectare scale was estimated by applying the alkalinity concentration data to the average water holding capacity of the soil (53 cm profile depth) based on physical data typical for this Series (United States Department of Agriculture, Natural Resources Conservation Service, 1977). Per hectare estimates for C sequestration potential (Table 2) assume one volumetric replacement of the soil’s total water holding capacity (i.e., a “flushing event”).
Total CO2 removal potential of the mineral amendment was calculated based on the assumption that all base cations (Mg, Ca, K, Na) are charged balanced by bicarbonate. The total amount of mineral amendment dissolved over the course of the experiment was estimated through calculating the total bicarbonate produced based on alkalinity measurements, then subtracting the alkalinity value of the soil only treatment. These bicarbonate estimates were converted to mg of C sequestered per g of tailings in each treatment. This C sequestration number was then compared to the total CO2 removal potential of the mineral amendment to estimate the total amount of mineral amendment that was dissolved during the course of the experiment.
3 Results
3.1 Soil and mineral amendment characterization
The soil was a loamy sand (79% sand, 16% silt, 5% clay) composed almost wholly of quartz and feldspar, with small amounts of kaolinite and a 2:1 phyllosilicate (Supplementary Figure S1). Soil pH in water was 5.11 ± 0.01 (SE, n = 9). Water holding capacity (field capacity) was 0.48 ± 0.01 mL g−1 (SE, n = 9).
The mineral amendment had an Dv80 particle size of 70 μm. Dominant crystalline mineral phases in the mineral amendment included plagioclase feldspar, talc, a 1:1 phyllosilicate phase, serpentine-chlorite, quartz, pyroxene, iron-bearing phases (magnetite, ferrihydrite), a sulfur-iron phase (pyrrhotite; (Fe1-xSx)), kaolinite, mica, amphibole, and small amounts of carbonaceous minerals (calcite, dolomite, ankerite, siderite) (Figure 1). Note that only qualitative XRD analysis was performed, so quantitative measures of mineral phases were not possible. Base cations potentially contributing to enhanced weathering included (% by mass): Mg (5.50%) and Ca (1.05%). Potential toxic elements included Ni (0.20%), Cu (0.04%), and S (3.42%) (Figure 1). The pH in water was 6.29 ± 0.00 (SE, n = 2) and field water holding capacity was 0.48 mL g-1 (n = 1). The potential total CO2 removal of the mineral amendment based on base cation concentrations was 13.2 g of C per kg of tailings, or 48.3 g of CO2 per kg of tailings.
3.2 Leachate chemistry
In general, the addition of ameliorated mine tailings to the samples produced alkalinity and increased soil pH in the sampled leachate, though the lowest addition rate (1%) was almost never significantly different from the control treatment (0%). When averaged across time, treatments containing 4% and 25% additions of mineral amendments produced significantly more alkalinity than treatments containing 0% and 1% additions (p < 0.001). Similarly, 4% and 25% additions of mineral amendment also had elevated pH values in comparison to 0% and 1% additions (p < 0.0001). Differences among treatments were similar at each of the leachate sampling times, though there was some variation across time (Table 1). The presence of trees influenced both alkalinity and pH, though the directionality of the change differed among treatments and over time. When averaged across time, paired t-tests indicated that alkalinity was higher when trees were present in the 1% treatments (p = 0.0385), but not different in the 4% treatment (p = 0.4679), and lower in the 25% treatment (p < 0.0001). Trees decreased soil pH in the 4% (p < 0.0001) and 25% (p < 0.0001) treatments. The presence of trees substantially increased the variability in leachate chemistry (Table 1; Figures 2, 3).
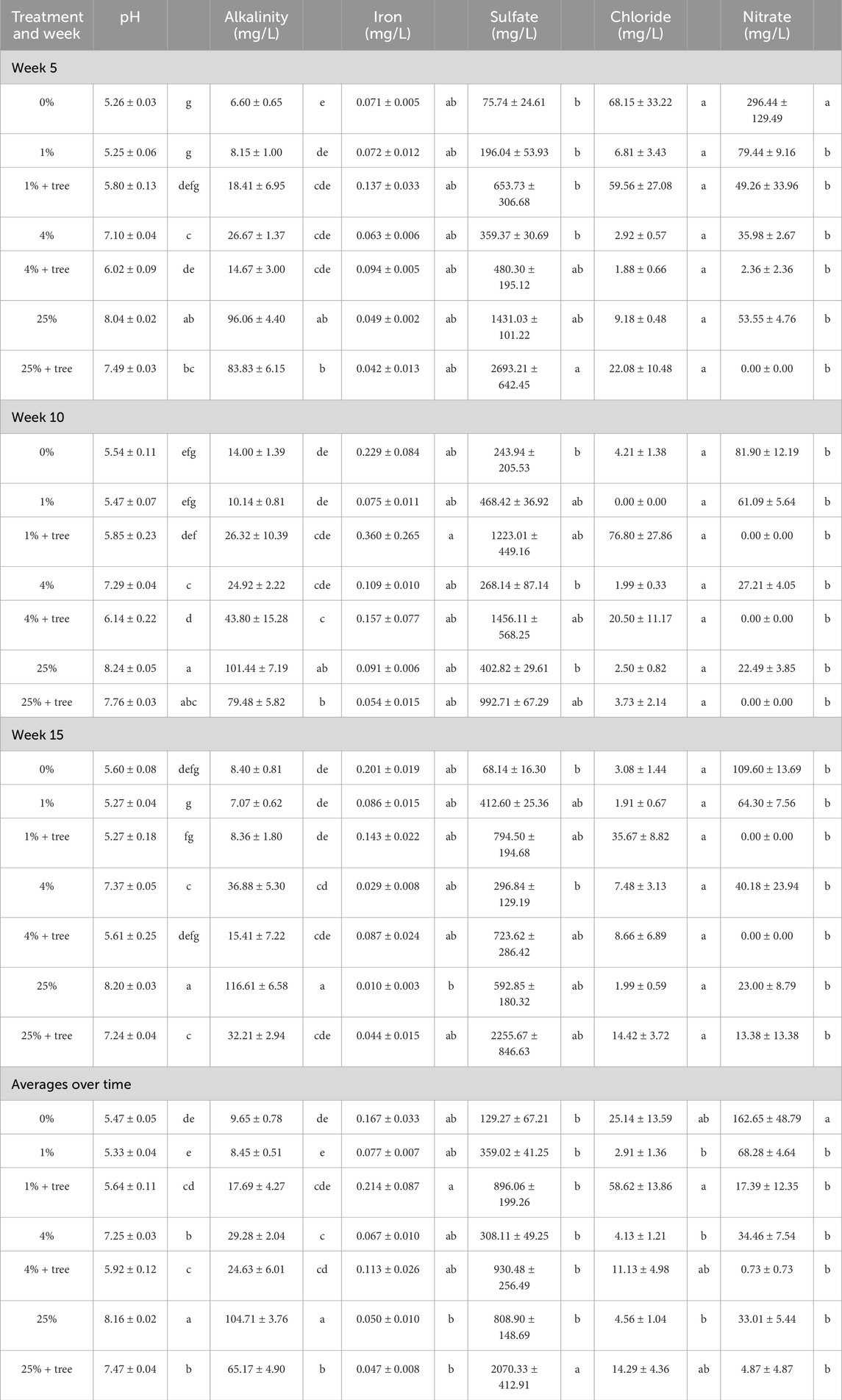
Table 1. Leachate sample chemistry. Values are the averages and standard errors of 12 replicates per treatment for pH and alkalinity, and 4 replicates per treatment for iron, sulfate, chloride and nitrate.Letters represent significance grouping across treatments according to Tukey’s post hoc HSD test at α = 0.05.
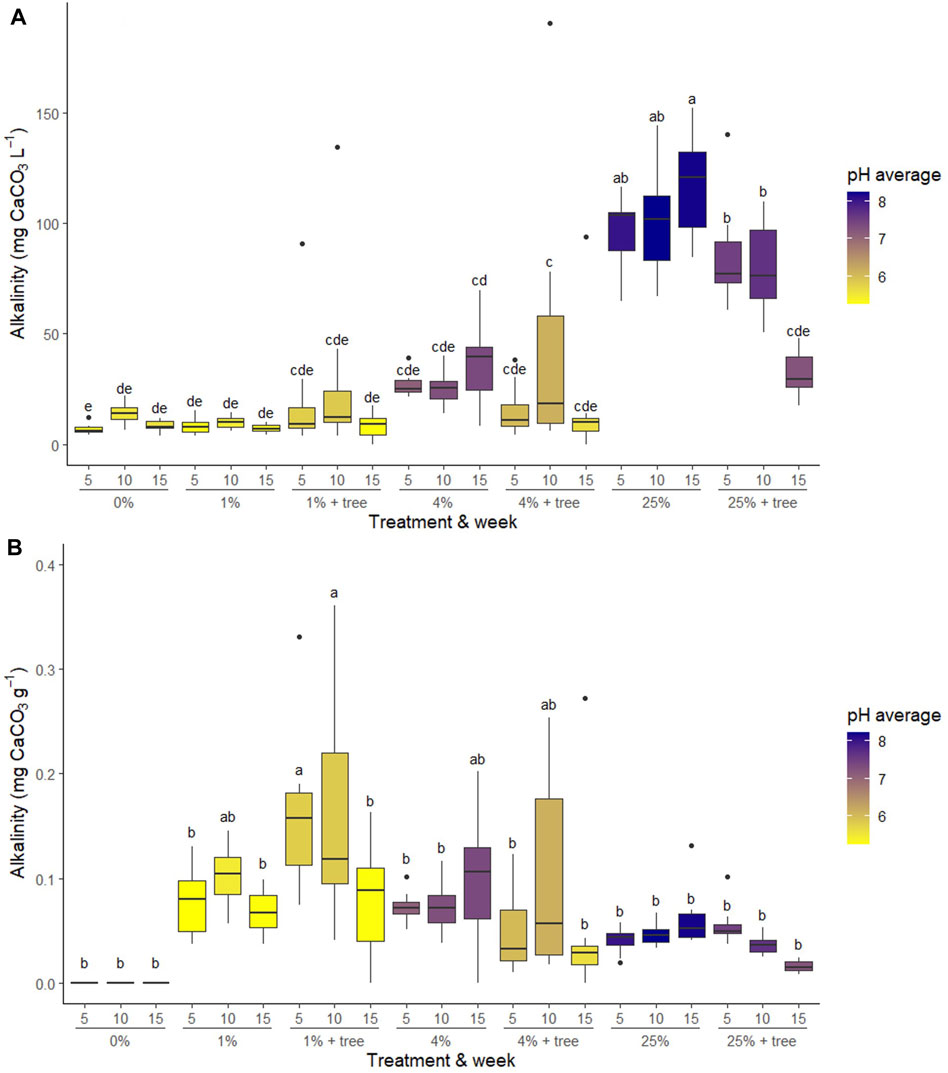
Figure 2. Alkalinity of treatments normalized to (A) Liters of leachate and, and (B) grams of mineral amendment. Values closest to the x-axis represent the week of leachate harvest (5 weeks, 10 weeks, 15 weeks after planting). Grouping values on the x-axis represent the amount of mineral amendment added to the soil as a mass percent, and the presence or absence of willow trees. Letters represent significance grouping according to Tukey’s post hoc HSD test at α = 0.05.
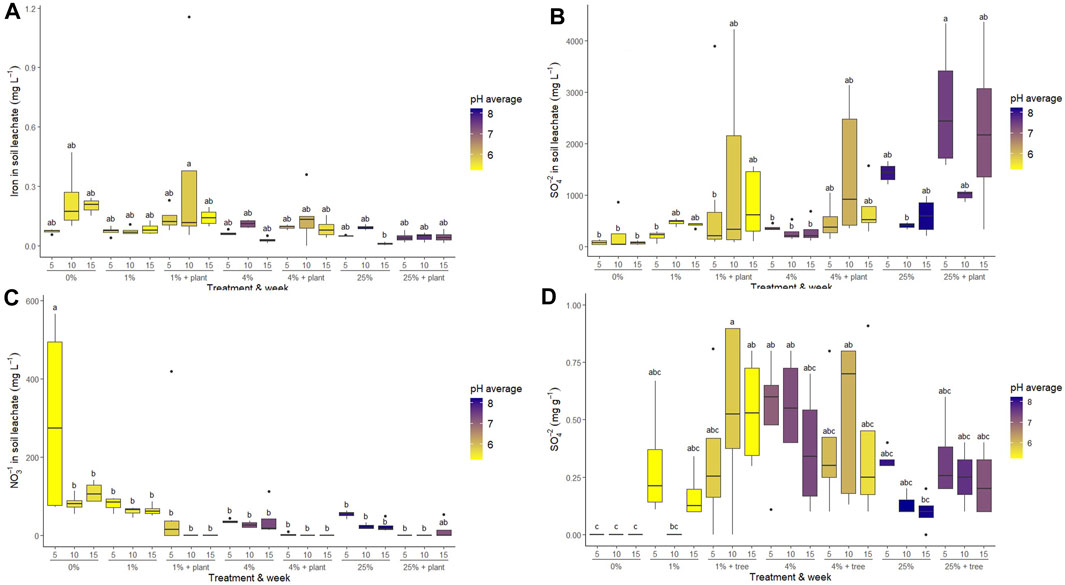
Figure 3. Concentrations of dissolved (A) Fe (mg L−1 leachate), (B) sulfate (mg L−1 leachate), (C) nitrate (mg L−1 leachate), and (D) sulfate (mg g−1 added mineral amendment) in leachate samples. Values closest to the x-axis represent the week of leachate harvest (5 weeks, 10 weeks, 15 weeks following planting). Grouping values on the x-axis represent the amount of mineral amendment added to the soil as a mass percent and the presence or absence of a willow trees. Letters represent significance grouping according to Tukey’s post hoc HSD test at α = 0.05. For comparison, concentrations of analytes in final artificial rainwater solutions used for watering the samples were as follows: 0.03 mg L−1 Na, 0.07 mg L−1 Ca, 0.02 mg L−1 Mg, 0.02 mg L−1 K, 0.70 SO4, 0.30 mg L−1 NH4, 0.21 mg L−1 NO3, 0.06 mg L−1 Cl.
Alkalinity in mg L−1 as CaCO3 was converted to equivalent masses of C and CO2 by dividing by molecular mass. Potential CO2 sequestration is expressed on a per liter and per hectare basis (Table 2). During the period of peak plant growth (week 10), alkalinity measurements of soil solution from the amended soils with willows indicated an additional 10 mg of inorganic C sequestration per liter of leachate in comparison to unamended soils. This is 4.5 times the inorganic C sequestration rate of unamended soils. The amount of mineral amendment dissolved over the course of the experiment was small, ranging from 0.6% in the 25% + tree treatment to 1.9% in the 1% + tree treatment.
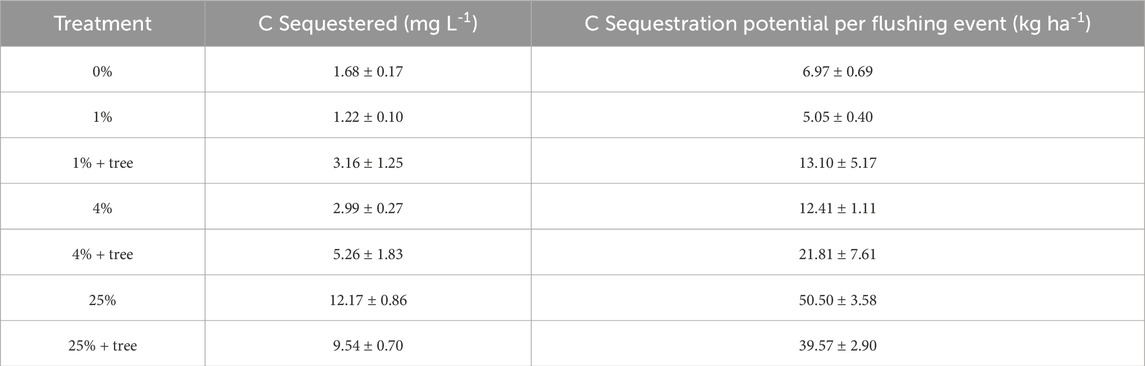
Table 2. Inorganic C sequestration potential of ameliorated tailings additions based on leachate measurements at 10 weeks of growth. Per hectare estimates are for a one-time flushing event where all soil pore water is replaced.
Leachate inorganic chemistry was variable over time and among treatments, but showed few significant treatment effects (Figure 3; Table 1). Dissolved Fe and sulfate (SO4−2) concentrations were measured as a potential metric of pyrrhotite dissolution. Very little Fe was present in leachate samples, with means of Fe concentration ranging from 0.010 to 0.360 mg L−1. There is a significant difference in Fe concentrations between the 1% + tree treatment and the 25% and 25% + tree treatments overall; however, the broad variance in the 1% + tree treatment is mainly due to a single measurement that was much higher than the average. Sulfate was significantly higher in the 25% + tree treatment than in the other treatments overall (p < 0.05). Treatments with trees had higher variability in their sulfate contents compared to treatments without trees. Nitrate tended to be higher in treatments without trees in weeks 5, 10, and 15; however overall, the only treatment with significantly higher nitrate content was the 0% treatment (p < 0.01).
Supplementary Table S2 shows the elemental composition of leachate solutions of individual metal elements from the ameliorated mine waste materials. Only two leachate samples from each treatment were measured for elemental concentrations by ICP. Concentrations of Fe, Al, and phosphate (PO43-) leached in the filtrate solutions after 5 weeks, 10 weeks and 15 weeks of root growth was minimal, while copper (Cu) concentrations were below detection level. The minimal amounts of PO43- found in the leachate solutions were attributed to the trace amount of these ions in the ameliorated mine waste materials. On the other hand, the amounts of Ca, Mg, and S in the leached solutions were found to be significant, and the amount of these ions increased with increasing percentage of mine waste product in the soil matrix. At 4% and 25% additions of mineral amendments, the concentrations of Ca and Mg in the leachate solutions reached up to −300 mg L−1 and −180 mg L−1, respectively. The S concentration in the leachate solutions were also found to be higher, in the range of 200–500 mg L−1. It is evident that both Ca and Mg from the serpentine, feldspar, pyroxene, amphibole and carbonate mineral phases were present in the leachate solution at neutral pH. S leaching can likely be attributed to the oxidation of pyrrhotite minerals from the amendment. Furthermore, low concentrations of Ni and manganese (Mn) in the range of 1–5 mg L−1 were also found in the leachate solutions.
3.3 Organic C accumulation in biomass and soil
There were no significant differences among treatments for total biomass, root biomass, shoot biomass, or root:shoot biomass ratio on a dry weight basis (Table 3; Figure 4). Willows grew an average of 4.33 ± 0.55 g of shoot biomass (n = 36) and 6.19 ± 1.41 g of root biomass (n = 36) for a total average of 10.51 ± 1.70 g of biomass (n = 36) during the 15-week time period. The ratio of roots to shoots were not significantly different among any of the treatments, with an average root:shoot ratio of 1.57 ± 0.31 (± standard error, n = 36).
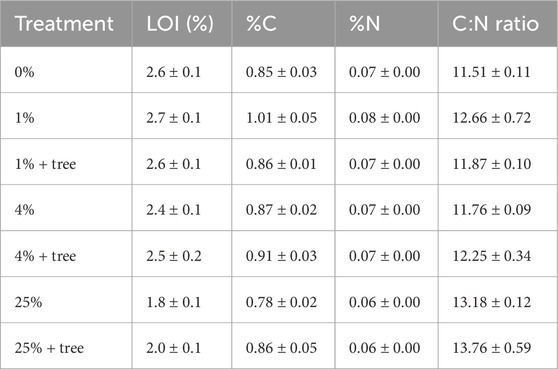
Table 3. Loss on ignition (LOI, n = 12), carbon (%C, n = 4), nitrogen (%N, n = 4), and C:N (n = 4) values of soil mixtures following 15 weeks of growth.
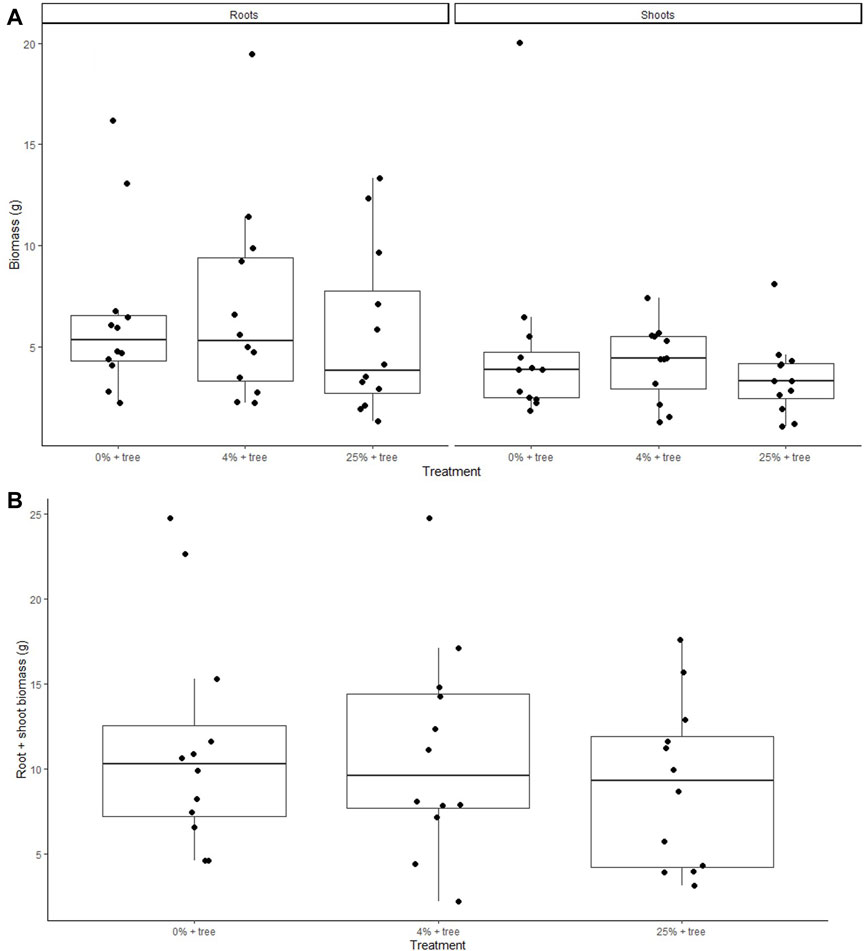
Figure 4. (A) Mass of willow roots and shoots grown during the 15-week incubation period. (B) Root:shoot ratio of willow trees following 15 weeks of growth. There were no significant differences between treatments.
Percent C, N and organic matter (as estimated through loss on ignition (LOI)) in the soil matrix clearly show a dilution effect associated with the addition of mine tailings. Because the mineral amendment had very low C and N concentrations in comparison to the soil itself, addition of mineral material decreased the overall concentrations of C, N, and organic matter. This dilution effect would not take place in the field, as the soil would not be volumetrically replaced in the field application as it was in the laboratory experiment. Because the motivation for these measurements was to assess whether the presence of plants would be associated with an organic C sequestration benefit, we compared treatments with versus without plants at each level of mineral amendment addition. We noted no differences in %C, %N or C:N associated with the presence of plants at any level of mineral amendment addition. However, loss on ignition data suggested OM accumulation associated with plant presence at the 25% addition level, with % organic matter values (% LOI) significantly higher in the treatment with trees than without (p = 0.0113). The other levels of mineral amendment treatment did not show evidence of increased organic matter associated with tree presence.
4 Discussion
4.1 Is there a carbon sequestration benefit associated with adding ameliorated mine tailings to fast-growing willows?
Our study provided evidence of inorganic C sequestration associated with the addition of ameliorated mine tailings to soils. A higher amount of inorganic C was sequestered in treatments with higher tailings content, and treatments with trees generally showed higher variability in alkalinity measurements. Soil pH also increased with the addition of higher amounts of tailings, a result consistent with previous work (Haque et al., 2019; Swoboda et al., 2022). Though the CO2 sequestration potential of the mineral amendments may seem small (Table 2), these values represent only a short time of weathering (15 weeks), and are similar to values associated with leaching of pure anorthite (Tepas et al., 2023).
The total bicarbonate produced by a particular feedstock material varies widely and is dependent not only on its base cation content, but also the weatherability of its mineral phases, particle size, application rate, and the climate of the region where it is deployed. This complicates intercomparisons of the absolute value of one feedstock over another. The ameliorated mining waste rock used in the current study has a base cation content on the lower end of materials currently being evaluated in the literature, −6.8% by weight in comparison to wollastonite often used for agricultural liming purposes (−25%, Haque et al., 2020b), pure olivine (−29%, Renforth et al., 2015), or ultramafic basalt (>10%, Beerling et al., 2020). However, its convenience and proven C sequestration potential may make its value sufficient for regional use.
It is additionally difficult to extrapolate mineral weathering rates observed in the laboratory to the field scale. In the area under evaluation for field deployment, Michigan’s Upper Peninsula, significant weathering of mineral amendments would likely only occur during the active growing season, and translocation of dissolved bicarbonates to depth would only be possible during rain events significant enough to replace soil pore water in the rooting zone. These values of C sequestration estimated by one such rainfall event (or with spring freshet) are small in comparison to the overall C sequestration capacity of the mineral feedstock (Table 2). However, given the ∼20-year life cycle of a willow planting (−7 coppicing events over a −20 years period) and the assumption of a uniform weathering rate over time, estimates indicate that 40% or more of the applied mineral material could be dissolved during that time period at an application rate of 1% mineral amendment by mass. Weathering rates may decline over time, however (Calabrese et al., 2022), so 40% is most likely an overestimate.
We measured bicarbonate production at multiple time points during the plant growth cycle and employed a high number of experimental replicates (n = 12) with the goal of gaining insight into the inherent variability of weathering rates, and by extension, C sequestration, associated with the deployment of enhanced weathering. We noted substantial variability both within treatments and over time, but the driving mechanism of the variability remains unclear. Variability in alkalinity production over time was most likely associated with variance in plant growth, root respiration and exudate production (Lucas, 2001). However, treatments demonstrated different patterns over time, and individual time points were often not different from one another due to the high within-treatment variance (Figure 2). The variability in pH and alkalinity within treatments with willows (Figure 2) may have been due to the presence of beneficial microbes (mycorrhizae) in some pots but not others, as the soil was not sterilized or pasteurized prior to use in this study. The influence of mycorrhizal associations on enhanced weathering rates deserves further study (Koele et al., 2014).
We hypothesized an additional C sequestration benefit of ameliorated tailings use associated with greater accrual of organic C in soils and in plant biomass (Haque et al., 2019). We observed no direct evidence of greater biomass production or organic C accumulation in soils (Table 3) associated with tailings additions (Figure 4A). While there was no obvious benefit to tree growth, there was also no evidence of harm to the trees from the tailings additions, even at concentrations of 25% by mass. The short timescale (15 weeks) and small pot size also likely limited the growth of the willows in this study, possibly contributing to the lack of treatment effects. Treatment impacts on tree growth and health may be present when grown in the field for one or more coppice rotations; such field testing may be warranted. In the current study, tree growth was likely limited by the availability of N, as shown by the lack of NO3− in the treatments containing willows (Figure 3C; Table 1). It is likely that the trees became root bound in the pots over the course of the experiment and were unable to grow past a certain point, which may be why a lack of significant differences in biomass among treatments was observed. If these willows were to be grown for a shorter period of time in the current pots and harvested before becoming root bound, or grown in larger pots for a longer period of time and harvested before becoming root bound, we would anticipate greater differences among treatments.
When calculating the C sequestration benefit of enhanced weathering, a full life cycle assessment must be conducted which includes monetary and C costs associated with feedstock modification (purification and comminution), transport and field application (Strefler et al., 2018). In this context, there are both benefits and drawbacks to using mining waste rock as feedstock. Carbon dioxide emissions and costs associated with mining and transport are strongly reduced in comparison to the use of ultramafic rock. However, the costs of purifying the mining waste may equal or surpass the savings associated with its proximity and previous extraction and comminution. An accurate life cycle assessment for the material used in the current study is not currently possible, but the froth flotation process used for removal of iron sulfide phases is commonly used in the mining industry (Mesa and Brito-Parada, 2019), and therefore costs can be assessed if field trials are deemed a desirable next step.
4.2 Is there a danger of environmental toxicity associated with ameliorated tailings additions to soils?
Mine tailings and mine waste are most often associated with negative environmental effects such as acid mine drainage, toxic aerosols, and contributions of heavy metals to surrounding areas (Akcil and Koldas, 2006; Sun et al., 2018; Vandeginste et al., 2024). Acid mine drainage is produced through reduction of iron sulfide minerals and subsequent production of sulfuric acid. We observed substantial dissolution of iron sulfide minerals in the current study. However, while concentrations of S were high in leachate from the treatments with tailings amendment in the current study, solutions maintained strongly alkaline pH values, and S was found in the form of sulfate, most likely charge balanced by Mg cations. From a public health perspective, sulfate concentrations in leachate far exceeded the EPA recommended upper limit of 250 mg L−1 for drinking water (Safe Drinking Water Act Amendments of 1996, 1996), and the fate of dissolved sulfate is likely transformation to hydrogen sulfide, either biotically or abiotically (Maier et al., 2009) which has deleterious effects on plant and animal life (Rubright et al., 2017). These results indicate that further removal of sulfur-bearing mineral phases necessarily precludes the use of this feedstock for enhanced weathering. The high S concentrations however did not appear to have a negative effect on tree survival nor health. There was a 0% mortality rate of willows in this study, demonstrating these willows were able to tolerate high concentrations of S, which was consistent with previous findings from McBride et al. (2016). While there was no statistically significant increase in biomass in the treatments with mine tailings, the willows’ ability to tolerate these S concentrations was encouraging for the possibility of a field trial following additional modification of mineral feedstock. Though pyrrhotite weathering should produce both dissolved Fe as well as dissolved S, we did not observe concentrations of Fe equivalent to those of S due to the low solubility of Fe at high pH (Figure 3A; Table 1). Fe was retained in the mineral matrix likely through a combination of sorption to organic and crystalline mineral surfaces and reprecipitation in less crystalline forms. It should be noted that S content in the waste rock can be further decreased by optimizing both grinding and froth flotation processes for sulfur removal (Mesa and Brito-Parada, 2019), mitigating the risks of environmental S toxicity associated with this amendment. This increased processing of the amendment does come with increased carbon costs which need to be taken into account when scaling up this process.
Nickel is another environmental toxin of concern associated with mining operations in Michigan’s UP. Ni is a human, animal, and environmental health concern when released from mining operations; it is toxic to animals and most plants at high enough concentrations (Das et al., 2018). Average Ni concentrations in the mineral amendment were 2,000 ppm, well above the U.S. Environmental Protection Agency’s maximum allowable concentration of 75 ppm for sludges broadcasted as field amendments (U.S. EPA, 1993), but nearly equivalent to ultramafic rocks such as dunite which have also been proposed for use in enhanced weathering (Beerling et al., 2018; Suhrhoff, 2022). Species that accumulate Ni, such as willow species and their hybrids, can be effective in removing Ni from contaminated sites, and have previously been suggested as a useful species in combining enhanced weathering and phytoremediation (Pilipović et al., 2019; Suhrhoff, 2022). Ni toxicity in trees often presents as inhibition of growth, chlorosis, wilting, and death (Drzewiecka et al., 2012; Bhalerao et al., 2015). The willows in our study did not display these symptoms, which was consistent with the knowledge that willows are tolerant to high concentrations of Ni (Marmiroli et al., 2011; Drzewiecka et al., 2012).
4.3 Can willows be used for phytomining of Ni in ameliorated mine tailings?
Based on our analysis of Ni content in plant shoot ash (0.02% Ni for 1% addition treatment and 0.05% Ni for 25% addition treatment, Supplementary Figure S2), it does not appear that the willows were accumulating high levels of Ni. However, this is likely due to the short duration of the experiment, and longer-term studies are needed to examine the Ni sequestration potential. Overall, based on biomass production and Ni concentrations in shoots, the willows removed approximately 5% of the Ni from the mineral amendment in the 1% addition treatment, but only 0.6% of the Ni applied in the 25% addition treatment. This data may suggest that willows could effectively remove much of the applied heavy metals over the −20 years lifespan of the crop if application rates were on the lower end. Willows do not meet the threshold for nickel accumulation (0.1% dry biomass) to be considered hyperaccumulators (Baker and Brooks, 1989; Brooks et al., 1998), however they produce higher biomass than many hyperaccumulator species and thus can be useful in phytoremediation (Borišev et al., 2009). According to Nkrumah et al. (2016) and Rascio and Navari-Izzo (2011), a concentration of >1% Ni in dry biomass is needed for a plant to be potentially suitable for phytomining. The 0.05% concentration seen in the willows of our study is much too low for phytomining, however given a longer growing season, a more specialized variety of willow, and a higher concentration of Ni in the tailings amendment, the willows may still be useful for “phytoprevention” of heavy metal contamination in soils amended with mine tailings for enhanced weathering (Suhrhoff, 2022). This “phytoprevention” of Ni accumulation in soils could help mitigate the negative environmental effects associated with Ni in our tailings amendment. Preventing Ni accumulation in soils would also help prevent Ni concentrations from surpassing regulatory limits (Dupla et al., 2023), possibly allowing for sustained application of mineral amendments derived from tailings materials. More specialized willow species may contain as much as 0.1% Ni on a dry biomass basis (Brooks et al., 1998). Assuming average biomass production rates of 12 tonnes dry weight per hectare each year (Cuniff et al., 2015), willows could extract more than a tonne of Ni from the applied mineral amendment annually. If the mineral amendment used in the current study was used at application rates suggested for basalts (30 t ha−1; Beerling et al., 2018), this would equal an addition of 6 tonnes of Ni to the soil, an amount hypothetically extractable given favorable conditions.
4.4 The potential for providing ecosystem services
The use of mining waste amendments for enhanced weathering has the potential to provide several ecosystem services, possibly with a lower than average monetary cost due to the local availability of the feedstock. However, all costs and benefits must be evaluated in light of a full life cycle assessment. Production of alkalinity has the potential to improve soil health, including the availability of nutrients such as phosphorus (Adams, 1984), and may offer beneficial knock-on effects if dissolved bicarbonates eventually travel through the watershed and into the Great Lakes (Rowe et al., 2020). These benefits are in addition to the most basic goal of providing a direct mechanism for drawdown of atmospheric CO2 (Seifritz, 1990; Schuiling and Krijgsman, 2006; Hartmann and Kempe, 2008; Köhler et al., 2010; ten Berge et al., 2012; Hartmann et al., 2013; Beerling et al., 2018; Beerling et al., 2020; Strefler et al., 2018; Kelland et al., 2020). In addition to increasing soil pH, weathering of the added soil amendment provides dissolved cations to the soil solution which may improve or maintain the suitability of local poor quality soils for bioenergy crop production (Kelland et al., 2020; Hartmann et al., 2013; Swoboda et al., 2022). Bioenergy crop production offers another potential climate change mitigation opportunity in itself (Volk et al., 2016; Montes et al., 2021). Though the use of local mining waste as a soil amendment has many potential benefits, these must be considered in balance with the potential for the addition of toxic levels of heavy metals, Ni being of primary concern with this specific feedstock material. Willows have shown promise in the context of phytoprevention (Suhrhoff, 2022), and thereby the combination of local mineral feedstock and bioenergy crop production may be a viable local climate change mitigation approach. However, further refinement of feedstock, selection of specialized willow varieties and a full life cycle assessment are necessary to fully evaluate the appropriateness of these activities.
We found significant production of alkalinity and increase in soil pH following addition of ameliorated mine waste rock to the sandy soils of Michigan’s Upper Peninsula. Though the mineral amendment contained significant concentrations of iron sulfide and Ni, willow trees grown in the amended soil showed no signs of suffering from toxicity. Willow biomass production did not increase with treatment, but this is likely due to other resource limitations such as nitrogen or pot size. Dissolution of mineral amendments over the course of the experiment yielded Ni and S concentrations in effluent far in excess of acceptable levels, indicating additional processing of these and similar feedstocks is necessary if they are to be utilized for large-scale enhanced weathering installations. Nickel uptake by willows was far less than the level necessary for phytomining but may have been sufficient for phytoprevention over the course of a typical willow planting lifecycle. Specialized willow varieties better adapted for heavy metal uptake would likely improve the possibility of phytomining with materials similar to those tested in the current study. In summary, we found that ameliorated mine waste rock can be used to produce bicarbonate when applied to soils and thereby has some C sequestration potential, and in combination with the cultivation of bioenergy crops such as willow may have potential for use as a feedstock in field trials. A full life cycle assessment is necessary to assess the potential benefits of using ameliorated mine waste rock for enhanced weathering purposes.
Data availability statement
The raw data supporting the conclusions of this article will be made available by the authors, without undue reservation. Raw data is additionally available in the Supplementary Materials.
Author contributions
MR: Data curation, Formal Analysis, Investigation, Methodology, Visualization, Writing–original draft, Writing–review and editing. KH: Conceptualization, Data curation, Formal Analysis, Funding acquisition, Investigation, Methodology, Project administration, Resources, Supervision, Visualization, Writing–original draft, Writing–review and editing. LP: Conceptualization, Data curation, Investigation, Methodology, Project administration, Resources, Writing–original draft, Writing–review and editing. XY: Investigation, Writing–review and editing. RZ: Project administration, Resources, Writing–original draft, Writing–review and editing. EK: Conceptualization, Data curation, Funding acquisition, Investigation, Methodology, Resources, Writing–original draft, Writing–review and editing.
Funding
The author(s) declare that financial support was received for the research, authorship, and/or publication of this article. This project was jointly supported by Michigan Technological University (Summer Undergraduate Research Fellowship) and the USDA Forest Service, Northern Research Station.
Acknowledgments
We acknowledge the support of the College of Forestry Resources and Environmental Science’s analytical services laboratory. We thank Jennifer Eikenberry for laboratory analyses and consultation.
Conflict of interest
The authors declare that the research was conducted in the absence of any commercial or financial relationships that could be construed as a potential conflict of interest.
The author(s) declared that they were an editorial board member of Frontiers, at the time of submission. This had no impact on the peer review process and the final decision.
Publisher’s note
All claims expressed in this article are solely those of the authors and do not necessarily represent those of their affiliated organizations, or those of the publisher, the editors and the reviewers. Any product that may be evaluated in this article, or claim that may be made by its manufacturer, is not guaranteed or endorsed by the publisher.
Supplementary material
The Supplementary Material for this article can be found online at: https://www.frontiersin.org/articles/10.3389/feart.2024.1414437/full#supplementary-material
References
Akcil, A., and Koldas, S. (2006). Acid Mine Drainage (AMD): causes, treatment and case studies. J. Clean. Prod. 14, 1139–1145. doi:10.1016/j.jclepro.2004.09.006
Ali, H., Khan, E., and Sajad, M. A. (2013). Phytoremediation of heavy metals--concepts and applications. Chemosphere 91, 869–881. doi:10.1016/j.chemosphere.2013.01.075
Baker, A. J. M., and Brooks, R. R. (1989). Terrestrial higher plants which hyperaccumulate metallic elements A review of their distribution, ecology and phytochemistry. Biorecovery 1, 81–126.
Beerling, D. J., Kantzas, E. P., Lomas, M. R., Wade, P., Eufrasio, R. M., Renforth, P., et al. (2020). Potential for large-scale CO2 removal via enhanced rock weathering with croplands. Nature 583, 242–248. doi:10.1038/s41586-020-2448-9
Beerling, D. J., Leake, J. R., Long, S. P., Scholes, J. D., Ton, J., Nelson, P. N., et al. (2018). Farming with crops and rocks to address global climate, food and soil security. Nat. Plants 4, 138–147. doi:10.1038/s41477-018-0108-y
Bhalerao, S. A., Sharma, A. S., and Poojari, A. C. (2015). Toxicity of nickel in plants. Int. J. Pure Appl. Biosci. 3, 345–355.
Borišev, M., Pajević, S., Nikolić, N., Pilipović, A., Krstić, B., and Orlović, S. (2009). Phytoextraction of Cd, Ni, and Pb using four willow clones (Salix spp.). Pol. J. Environ. Stud. 18, 553–561.
Brooks, R. R., Chambers, M. F., Nicks, L. J., and Robinson, B. H. (1998). Phytomining. Trends Plant Sci. 3, 359–362. doi:10.1016/s1360-1385(98)01283-7
Bullock, L. A., James, R. H., Matter, J., Renforth, P., and Teagle, D. A. H. (2021). Global carbon dioxide removal potential of waste materials from metal and diamond mining. Front. Clim. 3. doi:10.3389/fclim.2021.694175
Calabrese, S., Wild, B., Bertagni, M. B., Bourg, I. C., White, C., Aburto, F., et al. (2022). Nano-to global-scale uncertainties in terrestrial enhanced weathering. Environ. Sci. Technol. 56 (22), 15261–15272. doi:10.1021/acs.est.2c03163
Cunniff, J., Purdy, S. J., Barraclough, T. J., Castle, M., Maddison, A. L., Jones, L. E., et al. (2015). High yielding biomass genotypes of willow (Salix spp.) show differences in below ground biomass allocation. biomass bioenergy 80, 114–127. doi:10.1016/j.biombioe.2015.04.020
Das, K. K., Reddy, R. C., Bagoji, I. B., Das, S., Bagali, S., Mullur, L., et al. (2018). Primary concept of nickel toxicity - an overview. J. Basic Clin. Physiology Pharmacol. 30, 141–152. doi:10.1515/jbcpp-2017-0171
Drzewiecka, K., Mleczek, M., Gasecka, M., Magdziak, Z., and Golinski, P. (2012). Changes in Salix viminalis L. cv. 'Cannabina' morphology and physiology in response to nickel ions--hydroponic investigations. J. Hazard Mater 217-218, 429–438. doi:10.1016/j.jhazmat.2012.03.056
Dupla, X., Möller, B., Baveye, P. C., and Grand, S. (2023). Potential accumulation of toxic trace elements in soils during enhanced rock weathering. Eur. J. Soil Sci. 74. doi:10.1111/ejss.13343
Gee, G. W., and Bauder, J. W. (1986). Particle-size analysis. Methods of soil analysis: Part 1 Physical and mineralogical methods. America: American Society of Agronomy/Soil Science Society of America.
Goll, D. S., Ciais, P., Amann, T., Buermann, W., Chang, J., Eker, S., et al. (2021). Potential CO2 removal from enhanced weathering by ecosystem responses to powdered rock. Nat. Geosci. 14, 545–549. doi:10.1038/s41561-021-00798-x
Haque, F., Chiang, Y. W., and Santos, R. M. (2020a). Risk assessment of Ni, Cr, and Si release from alkaline minerals during enhanced weathering. Open Agric. 5, 166–175. doi:10.1515/opag-2020-0016
Haque, F., Chiang, Y., and Santos, R. (2019). Alkaline mineral soil amendment: a climate change stabilization wedge. Energies 12, 2299. doi:10.3390/en12122299
Haque, F., Santos, R. M., and Chiang, Y. W. (2020b). CO2 sequestration by wollastonite-amended agricultural soils – an Ontario field study. Int. J. Greenh. Gas Control 97, 103017. doi:10.1016/j.ijggc.2020.103017
Hartmann, J., and Kempe, S. (2008). What is the maximum potential for CO2 sequestration by ‘stimulated’ weathering on the global scale? Naturwissenschaften 95, 1159–1164. doi:10.1007/s00114-008-0434-4
Hartmann, J., West, A. J., Renforth, P., Köhler, P., de la Rocha, C. L., Wolf-Gladrow, D. A., et al. (2013). Enhanced chemical weathering as a geoengineering strategy to reduce atmospheric carbon dioxide, supply nutrients, and mitigate ocean acidification. Rev. Geophys. 51, 113–149. doi:10.1002/rog.20004
Jama-Rodzeńska, A., and Nowak, W. (2012). Nickel and lead uptake by willows (Salix viminalis L.). Pol. J. Agron. 11, 29–33.
Kanzaki, Y., Planavsky, N. J., and Reinhard, C. T. (2023). New estimates of the storage permanence and ocean co-benefits of enhanced rock weathering. PNAS nexus 2 (4), pgad059. doi:10.1093/pnasnexus/pgad059
Kelland, M. E., Wade, P. W., Lewis, A. L., Taylor, L. L., Sarkar, B., Andrews, M. G., et al. (2020). Increased yield and CO2 sequestration potential with the C4 cereal Sorghum bicolor cultivated in basaltic rock dust-amended agricultural soil. Global Change Biol. 26 (6), 3658–3676. doi:10.1111/gcb.15089107
Keoleian, G. A., and Volk, T. A. (2005). Renewable energy from willow biomass crops: life cycle energy, environmental and economic performance. Crit. Rev. Plant Sci. 24, 385–406. doi:10.1080/07352680500316334
Khalidy, R., and Santos, R. M. (2021). The fate of atmospheric carbon sequestrated through weathering in mine tailings. Miner. Eng. 163, 106767. doi:10.1016/j.mineng.2020.106767
Kocik, A., Truchan, M., and Rozen, A. (2007). Application of willows (Salix viminalis) and earthworms (Eisenia fetida) in sewage sludge treatment. Eur. J. Soil Biol. 43, S327–S331. doi:10.1016/j.ejsobi.2007.08.019
Koele, N., Dickie, I. A., Blum, J. D., Gleason, J. D., and de Graaf, L. (2014). Ecological significance of mineral weathering in ectomycorrhizal and arbuscular mycorrhizal ecosystems from a field-based comparison. Soil Biol. Biochem. 69, 63–70. doi:10.1016/j.soilbio.2013.10.041
Köhler, P., Hartmann, J., and Wolf-Gladrow, D. A. (2010). Geoengineering potential of artificially enhanced silicate weathering of olivine. Proc. Natl. Acad. Sci. U. S. A. 107 (47), 20228–20233. doi:10.1073/pnas.1000545
Korzeniowska, J., and Stanislawska-Glubiak, E. (2018). Phytoremediation potential of Phalaris arundinacea, Salix viminalis and Zea mays for nickel-contaminated soils. Int. J. Environ. Sci. Technol. 16, 1999–2008. doi:10.1007/s13762-018-1823-7
Lackner, K. S., Wendt, C. H., Butt, D. P., Joyce, E. L., and Sharp, D. H. (1995). Carbon dioxide disposal in carbonate minerals. Energy 20, 1153–1170. doi:10.1016/0360-5442(95)00071-n
Lucas, Y. (2001). The role of plants in controlling rates and products of weathering: importance of biological pumping. Annu. Rev. Earth Planet. Sci. 29 (1), 135–163. doi:10.1146/annurev.earth.29.1.135
Mackenzie, F. T., and Lerman, A. (2006). “Brief overview of carbon on earth,” in Carbon in the geobiosphere — earth’s outer shell —. Dordrecht: Springer Netherlands.
Maier, R. M., Pepper, I. L., and Gerba, C. P. (2009). Environmental microbiology, 397. USA: Academic Press.
Marmiroli, M., Pietrini, F., Maestri, E., Zacchini, M., Marmiroli, N., and Massacci, A. (2011). Growth, physiological and molecular traits in Salicaceae trees investigated for phytoremediation of heavy metals and organics. Tree Physiol. 31, 1319–1334. doi:10.1093/treephys/tpr090
Mcbride, M. B., Martinez, C. E., and Kim, B. (2016). Zn, Cd, S and trace metal bioaccumulation in willow (Salix spp.) cultivars grown hydroponically. Int. J. Phytoremediation 18, 1178–1186. doi:10.1080/15226514.2016.1189401
Mesa, D., and Brito-Parada, P. R. (2019). Scale-up in froth flotation: a state-of-the-art review. Sep. Purif. Technol. 210, 950–962. doi:10.1016/j.seppur.2018.08.076
Michigan Automated Weather Network (2022). Michigan state university. Available at: https://enviroweather.msu.edu/.
Miller, R. O. (2018). Shrub willow (Salix) biomass crop performance on five sites over two rotations in Michigan, USA and the implications of adequate field testing to commercial producers. BioEnergy Res. 11, 803–815. doi:10.1007/s12155-018-9939-7
Mirck, J., Isebrands, J. G., Verwijst, T., and Ledin, S. (2005). Development of short-rotation willow coppice systems for environmental purposes in Sweden. Biomass Bioenergy 28, 219–228. doi:10.1016/j.biombioe.2004.08.012
Mohsin, M., Salam, M. M. A., Nawrot, N., Kaipiainen, E., Lane, D. J., Wojciechowska, E., et al. (2022). Phytoextraction and recovery of rare earth elements using willow (Salix spp.). Sci. Total Environ. 809, 152209. doi:10.1016/j.scitotenv.2021.152209
Montes, F., Fabio, E. S., Smart, L. B., Richard, T. L., Añó, R. M., and Kemanian, A. R. (2021). A semi-commercial case study of willow biomass production in the northeastern United States. Agron. J. 113, 1287–1302. doi:10.1002/agj2.20603
Moosdorf, N., Renforth, P., and Hartmann, J. (2014). Carbon dioxide efficiency of terrestrial enhanced weathering. Environ. Sci. Technol. 48, 4809–4816. doi:10.1021/es4052022
Nkrumah, P. N., Baker, A. J. M., Chaney, R. L., Erskine, P. D., Echevarria, G., Morel, J. L., et al. (2016). Current status and challenges in developing nickel phytomining: an agronomic perspective. Plant Soil 406, 55–69. doi:10.1007/s11104-016-2859-4
Pilipović, A., Zalesny, R. S., Rončević, S., Nikolić, N., Orlović, S., Beljin, J., et al. (2019). Growth, physiology, and phytoextraction potential of poplar and willow established in soils amended with heavy-metal contaminated, dredged river sediments. J. Environ. Manag. 239, 352–365. doi:10.1016/j.jenvman.2019.03.072
Rascio, N., and Navari-Izzo, F. (2011). Heavy metal hyperaccumulating plants: how and why do they do it? And what makes them so interesting? Plant Sci. 180, 169–181. doi:10.1016/j.plantsci.2010.08.016
R core team (2021). R: a language and environment for statistical computing. Vienna, Austria: R Foundation for Statistical Computing. Available at: https://www.R-project.org/.
Renforth, P., Pogge von Strandmann, P. A. E., and Henderson, G. M. (2015). The dissolution of olivine added to soil: implications for enhanced weathering. Appl. Geochem. 61, 109–118. doi:10.1016/j.apgeochem.2015.05.016
Rowe, M. D., Errera, R. M., Rutherford, E. S., Elgin, A. K., Pilcher, D. J., Day, J., et al. (2020). in NOAA ocean, coastal, and Great lakes acidification research plan. Editor N. O. A. A. ADMINISTRATION
Rubright, S. L. M., Pearce, L. L., and Peterson, J. (2017). Environmental toxicology of hydrogen sulfide. Nitric Oxide 71, 1–13. doi:10.1016/j.niox.2017.09.011
Salam, M. M. A., Kaipiainen, E., Mohsin, M., Villa, A., Kuittinen, S., Pulkkinen, P., et al. (2016). Effects of contaminated soil on the growth performance of young Salix (Salix schwerinii E. L. Wolf) and the potential for phytoremediation of heavy metals. J. Environ. Manage 183, 467–477. doi:10.1016/j.jenvman.2016.08.082
Schuiling, R. D., and Krijgsman, P. (2006). Enhanced weathering: an effective and cheap tool to sequester Co2. Clim. Change 74, 349–354. doi:10.1007/s10584-005-3485-y
Shuman, L. M., and Duncan, R. R. (2008). Soil exchangeable cations and aluminum measured by ammonium chloride, potassium chloride, and ammonium acetate. Commun. Soil Sci. Plant Analysis 21, 1217–1228. 1990 - Issue 13-16. doi:10.1080/00103629009368300
Sleight, N. J., and Volk, T. A. (2016). Recently bred willow (Salix spp.) biomass crops show stable yield trends over three rotations at two sites. BioEnergy Res. 9, 782–797. doi:10.1007/s12155-016-9726-2
SOIL SURVEY STAFF (2014). Kellogg soil Survey laboratory methods manual. USA: U.S. Department of Agriculture, Natural Resources Conservation Service.
Strefler, J., Amann, T., Bauer, N., Kriegler, E., and Hartmann, J. (2018). Potential and costs of carbon dioxide removal by enhanced weathering of rocks. Environ. Res. Lett. 13, 034010. doi:10.1088/1748-9326/aaa9c4
Suhrhoff, T. J. (2022). Phytoprevention of heavy metal contamination from terrestrial enhanced weathering: can plants save the day? Front. Clim. 3. doi:10.3389/fclim.2021.820204
Sun, W., Ji, B., Khoso, S. A., Tang, H., Liu, R., Wang, L., et al. (2018). An extensive review on restoration technologies for mining tailings. Environ. Sci. Pollut. Res. Int. 25, 33911–33925. doi:10.1007/s11356-018-3423-y
Swoboda, P., Döring, T. F., and Hamer, M. (2022). Remineralizing soils? The agricultural usage of silicate rock powders: a review. Sci. Total Environ. 807, 150976. doi:10.1016/j.scitotenv.2021.150976
Taylor, L. L., Quirk, J., Thorley, R. M. S., Kharecha, P. A., Hansen, J., Ridgwell, A., et al. (2015). Enhanced weathering strategies for stabilizing climate and averting ocean acidification. Nat. Clim. Change 6, 402–406. doi:10.1038/nclimate2882
Te Pas, E. E. E. M., Hagens, M., and Comans, R. N. J. (2023). Assessment of the enhanced weathering potential of different silicate minerals to improve soil quality and sequester CO2. Front. Clim. 4. doi:10.3389/fclim.2022.954064
ten Berge, H. F. M., van der Meer, H. G., Steenhuizen, J. W., Goedhart, P. W., Knops, P., Verhagen, J., et al. (2012). Olivine weathering in soil, and its effects on growth and nutrient uptake in ryegrass (Lolium perenne L.): a pot experiment. PLOS ONE 7 (8), 1–8. doi:10.1371/journal.pone.0042098
United States Department of Agriculture, Natural Resources Conservation Service. (1977). Soil Survey of delta county and hiawatha national forest of alger and schoolcraft counties. Washington: Michigan.
Vandeginste, V., Lim, C., and Ji, Y. (2024). Exploratory review on environmental aspects of enhanced weathering as a carbon dioxide removal method. Minerals 14, 75–30. doi:10.3390/min14010075
van der Ent, A., Baker, A. J., Reeves, R. D., Chaney, R. L., Anderson, C. W., Meech, J. A., et al. (2015). Agromining: farming for metals in the future? Environ. Sci. Technol. 49, 4773–4780. doi:10.1021/es506031u
Veverica, T. J., Kane, E. S., Marcarelli, A. M., and Green, S. A. (2016). Ionic liquid extraction unveils previously occluded humic-bound iron in peat soil pore water. Soil Sci. Soc. Am. J. 80, 771–782. doi:10.2136/sssaj2015.10.0377
Vicca, S., Goll, D. S., Hagens, M., Hartmann, J., Janssens, I. A., Neubeck, A., et al. (2022). Is the climate change mitigation effect of enhanced silicate weathering governed by biological processes? Glob. Change Biol. 28, 711–726. doi:10.1111/gcb.15993
Vinhal, R. A., Zalesny, R. S. J., Debauche, B. S., Rogers, E. R., Pilipović, A., Soolanayakanahally, R. Y., et al. (2022). Establishment of willows using the novel DeValix technique: ecological restoration mats designed for phytotechnologies. Int. J. Phytoremediation 24, 730–743. doi:10.1080/15226514.2021.1970102
Volk, T. A., Heavey, J. P., and Eisenbies, M. H. (2016). Advances in shrub-willow crops for bioenergy, renewable products, and environmental benefits. Food Energy Secur. 5, 97–106. doi:10.1002/fes3.82
Wang, Z., and Macfarlane, D. W. (2012). Evaluating the biomass production of coppiced willow and poplar clones in Michigan, USA, over multiple rotations and different growing conditions. Biomass Bioenergy 46, 380–388. doi:10.1016/j.biombioe.2012.08.003
Warncke, D., Bast, L., and Christenson, D. (2010). LIME FOR MICHIGAN SOILS. Available at: https://www.canr.msu.edu/uploads/resources/pdfs/lime-for-mi-soils-e-471.pdf.
Xing, Y., Gui, X., Pan, L., Pinchasik, B. E., Cao, Y., Liu, J., et al. (2017). Recent experimental advances for understanding bubble-particle attachment in flotation. Adv. colloid interface Sci. 246, 105–132. doi:10.1016/j.cis.2017.05.019
Keywords: phytotechnologies, enhanced weathering, carbon sequestration, bioenergy production, soil organic matter
Citation: Russell MD, Heckman KA, Pan L, Ye X, Zalesny RS Jr and Kane ES (2024) Mine waste rock as a soil amendment for enhanced weathering, ecosystem services, and bioenergy production. Front. Earth Sci. 12:1414437. doi: 10.3389/feart.2024.1414437
Received: 08 April 2024; Accepted: 26 July 2024;
Published: 12 August 2024.
Edited by:
Tao Wen, Syracuse University, United StatesReviewed by:
Tim Jesper Suhrhoff, Yale University, United StatesPatricia Fox, Berkeley Lab (DOE), United States
Copyright © 2024 Russell, Heckman, Pan, Ye, Zalesny and Kane. This is an open-access article distributed under the terms of the Creative Commons Attribution License (CC BY). The use, distribution or reproduction in other forums is permitted, provided the original author(s) and the copyright owner(s) are credited and that the original publication in this journal is cited, in accordance with accepted academic practice. No use, distribution or reproduction is permitted which does not comply with these terms.
*Correspondence: Katherine A. Heckman, a2F0aGVyaW5lLmEuaGVja21hbkB1c2RhLmdvdg==