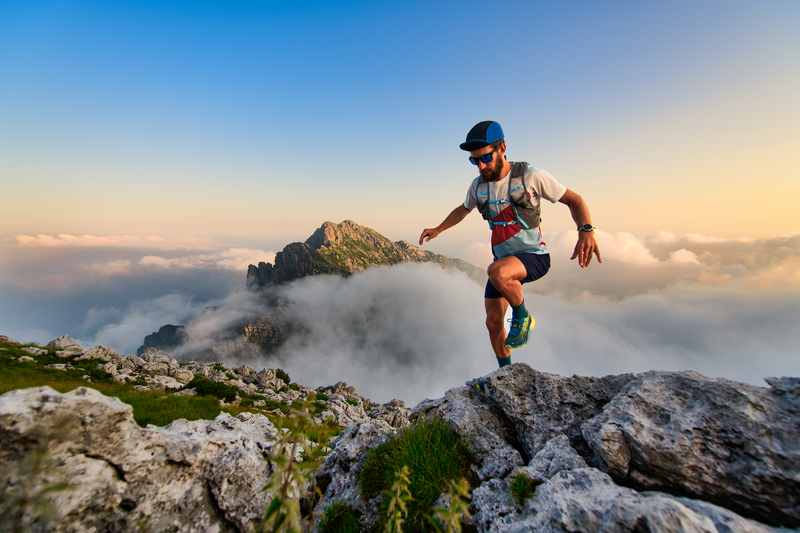
95% of researchers rate our articles as excellent or good
Learn more about the work of our research integrity team to safeguard the quality of each article we publish.
Find out more
ORIGINAL RESEARCH article
Front. Earth Sci. , 31 July 2024
Sec. Economic Geology
Volume 12 - 2024 | https://doi.org/10.3389/feart.2024.1403504
This article is part of the Research Topic Exploration, Development, and Protection of Earth’s Resources and Environment: Methods, Techniques, Applications, Prospects, Insights, and Problems View all 43 articles
Sulfur forms an immiscible liquid upon saturation in magma, and sulfide droplets are commonly found in fresh mid-ocean ridge basalt (MORB). Scanning electron microscopy (SEM) analysis revealed that the fine-grained and weakly phyric MORB samples exhibited hypocrystalline to vitreous textures. Transmission electron microscopy (TEM) of MORB glasses exhibits nanoscale sulfide droplets (10–15 nm) with rounded shapes and smooth edges, showing crystalline and homogeneous composition. Elemental distribution included S, Fe, Cu, and Ni, while Si, Al, and O were lacking. Prior research clarified the immiscibility between sulfide and silicate melts, impacting the size distribution of sulfide droplets. This is the first report on nanoscale sulfide droplets within MORB glasses, and these results suggest that nanoscale sulfide droplets represent the initial phase of sulfide saturation. Such an insight may prove useful in understanding how siderophile and chalcophile elements behaved during sulfide crystallization. In addition, this study determines the immiscibility of sulfides and silicate melts that occur in the early nanometer stage. Therefore, it is speculated that immiscibility phenomena may occur in the nanometer stage during magma evolution.
There are two fundamental differentiation patterns in magma evolution: segregation crystallization and immiscibility. Of these, liquid immiscibility is the more significant (Magloughlin, 2005; Thompson et al., 2007; Veksler et al., 2008; Charlier and Grove, 2012; Kamenetsky et al., 2013). Immiscibility refers to the process by which homogeneous magma decomposes into two melts with significantly different compositions due to changes in temperature, pressure, composition, and other factors. Immiscibility has been described in a broad compositional range of natural magmas such as basalt (Skinner and Peck, 1969; De, 1974), fourchite (Philpotts, 1972), lunar rocks (Roedder and Weiblen, 1970), and various volcanic rocks (Philpotts, 1982). The immiscibility of sulfide and silicate melts is a significant geological phenomenon in magmatic evolution. Specifically, immiscibility initiates during the early stages of magmatic evolution, leading to the initial separation of sulfide from the magma into small droplets, dispersing within the silicate melt (Wallace and Edmonds, 2011; Cox, 2013). Sulfur forms an immiscible liquid upon saturation in magma (e.g., sulfide droplets) (Moore and Calk, 1971; Moore and Schilling, 1973; Patten et al., 2012; Yang et al., 2014). Yeats and Mathez (1976) discovered spherical iron sulfide globules in the walls of vesicles in glassy selvages of tholeiitic basalt. Czamanske and Moore (1977) discovered spherical globules in phenocrysts and glasses of submarine basalt from the Mid-Atlantic Ridge. Sulfide droplets are commonly reported in fresh MORB glasses (Holzheid, 2010). MORB is considered the final product of passive upwelling, decompression, and melting of the mantle material (Brunelli et al., 2014; 2018; Paquet et al., 2016).
In addition, Moore and Calk (1971), Vakhrushev and Prokoptsev (1972), Kanehira et al. (1973), and Mathez and Yeats (1976) described sulfide droplets in some MORB glasses, which are interpreted to represent immiscible sulfide–silicate melts existing at near-liquidus temperatures. Therefore, the well-preserved sulfide droplets in MORB glasses provide an opportunity to investigate the equilibrium between sulfide melt and silicate melt (Yeats and Mathez, 1976; Czamanske and Moore, 1977; Patten et al., 2013). These investigations indicated the sulfide droplets are typically micro-sized and display zoned and fine-grained textures (Czamanske and Moore, 1977; Patten et al., 2012; 2013; Yang et al., 2014). During mantle melting and differentiation of basalt melt, the size of the sulfide droplets formed affects the physical behavior of the separated sulfide phases. However, the separation of sulfide droplets has an insignificant impact on the distribution of siderophile and chalcophile elements (Yeats and Mathez, 1976; Czamanske and Moore, 1977; Hamlyn et al., 1985; Peach et al., 1990; Rehkämper et al., 1999; Bézos et al., 2005; Patten et al., 2013). Existing reports provide limited coverage of the formation of these sulfide droplets and the subsequent scavenging of siderophile and chalcophile elements. A profound understanding of the initial solidification of sulfide–silicate liquids remains a worthwhile endeavor.
Here, material extracted in situ from the surface of polished MORB glass samples is examined by scanning electron microscopy (SEM). Focused ion beam (FIB) techniques coupled with transmission electron microscopy (TEM) and high-angle annular dark-field scanning transmission electron microscopy (HAADF STEM) are employed to 1) investigate micro sulfide droplets in basaltic glass and 2) elucidate the initial stages of sulfide immiscibility in MORB glasses.
The MORB samples were dredged along the axis of the Southwest Indian Ridge (SWIR) during cruises DY105-17, DY115-19, and DY115-20 aboard the R/V DaYangYiHao. The SWIR is an ultraslow-spreading ridge (Dick et al., 2003) extending from the Bouvet Triple Junction (54°50S, 00°40′W) in the South Atlantic Ocean to the Rodrigues Triple Junction (25°30S, 70°00′E) in the Indian Ocean. The MORB samples in this study were selected from the three main ridge segments delimited by the Gallieni (52°20′E) and Melville (60°45′E) fracture zones (Figure 1). SEM analysis revealed that the MORB samples were predominantly fine-grained with weakly porphyritic, hypocrystalline to vitreous textures. Phenocrysts ranged in size from 1 μm to 10 μm and were relatively rich in Si and O (Figures 2B–F).
Figure 1. Topographic map of the Southwest Indian Ridge (SWIR), showing sample locations used in this study (red circles).
Figure 2. SEM image (A) and O, Si, Na, Al, and Fe EDS mappings (B–F) of selected particles of MORB samples.
Normal microscopic analyses were conducted on polished, thin petrographic sections using a Zeiss-Opton light microscope with transmitted light. Rock platelets for SEM observations were polished, and observations were conducted using a Hitachi SU8220 at 5 kV and 10 kV. Elemental distribution was determined by energy-dispersive X-ray spectrometry (EDS).
A TEM (FEI Tecnai G2 F20) equipped with an EDS detector (0.5 to 1 wt% detection limit) was used to determine the structural/chemical information about the nanoscale sulfide droplets. The maximum TEM acceleration voltage is 200 kV. The TEM foil was extracted to attach Cu grids via Pt welding and thinned to thicknesses of 50–70 nm. HAADF-STEM imaging used an ultra-high-resolution and probe-corrected FEI Titan Themis TEM. High-resolution transmission electron microscopy (HRTEM) image processing, including fast Fourier transform (FFT), was accomplished using Gatan’s Digital Micrograph software (version 3.7.4). All analyses, including SEM and TEM, were conducted at the Sinoma Institute of Materials Research (Guangzhou) Co., Ltd.
Figure 3 illustrates the location of the selected FIB-cut of MORB glass, while Figure 4A displays this section, revealing numerous small inclusions with relatively high contrast. These inclusions differed in size and were distributed randomly within the matrix (Figure 4B). Figure 4C also shows a rounded crystalline inclusion (15 nm) based on several diffraction spots recorded in the selected area of the electron diffraction (SAED) pattern. The lattice fringes were clearly recorded in the HRTEM images, except for some lattice defects, with the d-spacing measured as 1.74 Å (2–4–6). The mineral composition of the sulfide droplet was presumed to be pyrite (Figure 4D). Another crystalline round (∼20 nm) inclusion is shown in Figure 4E. The well crystalline structure also contained lattice defects, with the d-spacing measured as 1.94 Å (0 3–8), and the mineral composition of the sulfide droplet is presumed to be pyrrhotite (Figure 4F).
Figure 4. A focused ion beam (FIB)-cut of the MORB glasses (A, B): (A) TEM image. (B) Higher magnification image obtained from the rectangle in (A). (C) TEM image showing a nearly rounded sulfide droplet with its SAED pattern inserted. (D) HRTEM image the droplet in (B). (E) TEM image showing a rounded sulfide droplet with its SAED pattern inserted. (F) HRTEM image of the droplet in (E).
Under HAADF-STEM imaging, the nanoscale inclusion appears bright and consists of metallic elements (Figure 5). Compositionally, the inclusion primarily includes sulfur (Table 1), which indicates these tiny MORB glass inclusions are nanoscale sulfide droplets. According to elemental maps, the sulfide droplet is rich in Fe (20.49%), Cu (24.57%), Ni (2.78%) and S (13.15%) (Figure 6), but lacked Si (7.17%), O (20.49%) and Al (3.02%) (Figure 7).
Figure 6. Elemental maps of a typical sulfide droplet within MORB glasses, the sulfide droplets were relatively rich in Fe, Cu, Ni and S.
Figure 7. Elemental maps of a typical sulfide droplet within MORB glasses, the sulfide droplets were lack in Si, O and Al.
Many studies have reported the presence of sulfide globules in olivine crystals and matrix glasses, which indicated S-saturated fractionation during magmatic evolution (Patten et al., 2012; Yang et al., 2014). On the one hand, well-developed sulfide globules in MORBs are thought to take shape prior to the host magma quenching, like those recorded by Yeats and Mathez (1976) and Patten et al. (2012), which are 5–50 μm in size and round in shape (Yeats and Mathez, 1976; Holzheid, 2010; Patten et al., 2012). The solubility of sulfur in basalt is related to changes in melt composition, temperature, and oxygen and sulfur fugacity (Mavrogenes and O’Neill, 1999). On the other hand, some embayed sulfides similar to those recorded by Yang et al. (2013) are thought to form due to partial sulfide dissolution during decompression. Therefore, the saturation of sulfide droplets may be related to a decrease in pressure or temperature. In this study, the discovery of nanoscale sulfide droplets in natural MORB glasses demonstrates a new method of S-saturated fractionation. The recorded droplets (small in size and rounded in shape) are characterized by smooth edges and crystalline features. Consequently, sulfide saturation may have initially commenced in the form of nanobead droplets.
Although sulfide droplets are common in fresh MORB glasses such as phenocrysts and glass, microcrystalline aggregates of plagioclase and olivine, and vesicle walls (Yeats and Mathez, 1976; Czamanske and Moore, 1977; Patten et al., 2012; 2013; Yang et al., 2014), the micro-sized droplets have different textures ranging from a fine-grained intergrowth of monosulfide solid solution (MSS) and intermediate solid solution (ISS) to coarse-grained intergrowth of MSS and ISS accompanied by oxide and pentlandite (Yeats and Mathez, 1976; Czamanske and Moore, 1977). The textural differences of these droplets might be controlled by component nucleation and diffusion rates (Czamanske and Moore, 1977). For example, Patten et al. (2013) reported that fine-grained and coarse-grained droplets refer to the primitive sulfide liquid, and the zoned sulfide droplets correspond to the stage where MSS crystallizes and co-exists with a residual sulfide liquid (the quenched ISS) (Patten et al., 2013). Compared with those micro-sized sulfide droplets with various textures (Patten et al., 2013; Yang et al., 2013), the nanoscale sulfide droplets recorded in this paper differed significantly in terms of internal textures showing homogeneous compositions without internal fractionation of MSS and ISS phases. Furthermore, the sulfide droplets in this study are crystalline, suggesting the formation of their lattices during this stage (Figures 4D–F).
The sulfide droplet sizes varied at different stages of sulfur saturation, and the droplet sizes also controlled the textural differences of the droplets. Holzheid (2010) demonstrated the polymerization degree of the silicate liquid controlled the sulfide droplet size distribution. Patten et al. (2012) speculated a positive correlation between the droplet diameter increase and the degree of phase segregation. Furthermore, Peach et al. (1990) reported that the size of a globule should reflect its residence time in the melt and the frequency with which it contacts and consumes other globules. Ostwald’s ripening plays an important role in globule size change (Yang et al., 2014). The experimental results indicate that when the composition of silicate melt reaches sulfide saturation immiscible, the phase separation of sulfide nanoscale droplets and silicate nanoscale liquids/melts promptly emerges. Small droplets then disappear, enlarging the larger droplets, and this process is generally accompanied by a reduction in interface free energy (Mazurin and Porai-Koshits, 1984). In this study, the nanoscale sulfide droplets are small (10–15 nm) and have rounded shapes and smooth edges. In summary, sulfides appear to form very shortly before eruption, followed by immediate supercooling.
Sulfide plays an important role in the distribution of chalcophile elements during mantle melting and basalt melt differentiation (Mathez, 1976; Peach et al., 1990; Bézos et al., 2005). As sulfide melt droplets segregate, the droplets scavenge siderophile and chalcophile elements such as Ni, Cu, Pt, and Pd from the magma (Holzheid, 2010). Most micro-sized sulfide grains in MORBs contained MSS, the first solid to crystallize from a sulfide liquid, and ISS, which crystallizes from the remaining liquid (Patten et al., 2013). Therefore, the different chalcophile elements in MSS and ISS typically exhibit distinct behaviors. For instance, platinum-group elements (PGE) are scavenged by immiscible sulfides during the evolution of mafic magmas (Peach et al., 1990; Keays, 1995; Rehkämper et al., 1999; Song et al., 2006), but the iridium-subgroup of PGE (Os, Ir, Ru, and Rh) normally behaves as compatible elements and partition into MSS (Patten et al., 2013; Yang et al., 2014). Co and Re have a slight preference for MSS, whereas Cu, Zn, Au, Ag, Sn, Te, Cd, Bi, and Pb partition into ISS (Patten et al., 2013). The sulfide droplets reported by Yang et al. (2014) contained Ni-Fe-rich MSS and Cu-Fe-rich ISS phases.
However, existing literature does not precisely specify the timing of the chalcophile elements’ incorporation into sulfide droplets. Unlike micro-sized droplets, the nanoscale sulfide droplets in this study contain Fe, Cu, Ni, and S (Figure 6). These elements might have been scavenged during initial sulfide saturation and behaved similarly in the droplets. With the formation of sulfide beads, the elements Si, O, and Al are depleted at the same time. Based on the nanoscale sulfide droplets reported in this paper, it can be inferred that chalcophile elements enter sulfide droplets in the early stage and are uniformly distributed in the range of sulfide droplets. As sulfide droplets in MORB provide unique records of near-liquidus phase relations in basaltic systems, such an insight can help understand how chalcophile elements behave during sulfide crystallization. With longer residence times, sulfide droplets gradually evolve and develop different textures, including MSS and ISS, with each chalcophile element showing distinct preferences.
Immiscibility is an important factor in the process of magmatic evolution and mineralization. During magma immiscibility, a single component melt can decompose into two kinds of melts with completely different compositions. After magma immiscibility, the large-scale separation of conjugated two-phase melts affects the overall evolution of magma. Early immiscibility begins in the magma evolution; the greater the impact on magma evolution, the greater its geochemical and petrological significance (Veksler et al., 2008). The immiscibility between sulfide droplets and silicate melts is similarly pivotal in magma evolution and has been well-documented in previous studies. According to the elemental maps, the sulfide droplets reported here are relatively rich in Fe, Cu, Ni, and S (Figure 6) but lack Si, O, and Al (Figure 7), indicating that the immiscibility between sulfide and silicate melts occurred during the early stage. According to these results, the immiscibility of sulfide and silicate melt occurs at the nanoscale stage, so it can be inferred that the immiscibility in the process of magma evolution may occur at the nanoscale stage. The basic principle of magmatic sulfide deposit origin stems from the sulfur saturation of magma (Mungall and Naldrett, 2008). Sulfide droplets form when sulfur saturation occurs. Magmatic sulfide deposits form when sulfide droplets become sufficiently concentrated in a certain area of the magmatic body (Mungall and Naldrett, 2008). Studies have shown that the formation of magma-type Ni-Cu sulfide deposits is related to S-saturation in mantle-derived basic and ultramafic magmas and the separation and enrichment of immiscible sulfide droplets (Arndt et al., 2005). Moreover, the physicochemical action of mineralization in a magmatic sulfide system is primarily controlled by the dynamics and kinetics of transported sulfide droplets (Robertson et al., 2015). Therefore, clarifying the relationship between sulfide droplets and silicate melt is crucial for comprehending the formation process of magmatic sulfide deposits.
Nanoscale sulfide droplets were initially identified in MORB glasses by FIB-cut and TEM analyses. These droplets might form rapidly before an eruption and then undergo immediate supercooling, and they have characteristics of smooth edges and crystalline features. The discovery of nanoscale sulfide droplets in natural MORB glasses demonstrates a new form of sulfur saturation. It is more likely that the nanoscale sulfide droplets form during the initial stage of sulfide saturation. In addition, the sulfide droplets in this study exhibit a crystalline structure, suggesting that their lattices likely formed during supercooling. At the initial phase of sulfide saturation, the chalcophile elements enter the sulfide droplet in the early stage and are distributed uniformly in the droplet rather than selectively enriching the siderophile and chalcophile elements. However, it is still unclear when the siderophile and chalcophile elements behave inconsistently in sulfide droplets, which warrants further investigation. Furthermore, according to the element distribution map of sulfide droplets, sulfide droplets are relatively enriched in Fe, Cu, and Ni but lack Si, O, and Al. This observation further supports the occurrence of immiscibility between sulfide and silicate melts at the nanometer scale, providing new insights for studying the immiscibility stage during magma evolution.
The raw data supporting the conclusions of this article will be made available by the authors, without undue reservation.
LZ: conceptualization and writing–original draft. PZ: investigation, writing–review and editing. YW: methodology, writing–review and editing. RL: data curation and writing–review and editing.
The author(s) declare that financial support was received for the research, authorship, and/or publication of this article. This work was supported by the National Natural Science Foundation of China (NSFC) (grant numbers 42102076 and 42077129); the Natural Science Foundation of Shandong Province (grant numbers ZR2020ZD19 and ZR2021QD037); and the SDUT & Zibo City Integration Development Project (grant number 2021SNPT0012).
The authors declare that the research was conducted in the absence of any commercial or financial relationships that could be construed as a potential conflict of interest.
All claims expressed in this article are solely those of the authors and do not necessarily represent those of their affiliated organizations, or those of the publisher, the editors, and the reviewers. Any product that may be evaluated in this article, or claim that may be made by its manufacturer, is not guaranteed or endorsed by the publisher.
Arndt, N. T., Lesher, C. M., and Czamanske, G. K. (2005). Mantle-derived magmas and magmatic Ni-Cu-(PGE) deposits. Economic Geology 100th Aniversary volume, pp. 5‒24. doi:10.5382/AV100.02
Bézos, A., Lorand, J. P., Humler, E., and Gros, M. (2005). Platinum-group element systematics in mid-oceanic ridge basaltic glasses from the pacific, atlantic, and Indian oceans. Geochimical Cosmochimical Acta 69, 2613–2627. doi:10.1016/j.gca.2004.10.023
Brunelli, D., Cipriani, A., and Bonatti, E. (2018). Thermal effects of pyroxenites on mantle melting below mid-ocean ridges. Nat. Geosci. 11 (7), 520–525. doi:10.1038/s41561-018-0139-z
Brunelli, D., Paganelli, E., and Seyler, M. (2014). Percolation of enriched melts during incremental open-system melting in the spinel field: a REE approach to abyssal peridotites from the Southwest Indian Ridge. Geochimica Cosmochimica Acta 127, 190–203. doi:10.1016/j.gca.2013.11.040
Charlier, B., and Grove, T. L. (2012). Experiments on liquid immiscibility along tholeiitic liquid lines of descent. Contribution Mineralogy Petrology 164, 27–44. doi:10.1007/s00410-012-0723-y
Cox, K. G. (2013). The interpretation of igneous rocks. Berlin, Germany: Springer Science & Business Media.
Czamanske, G. K., and Moore, J. G. (1977). Composition and phase chemistry of sulfide globules in basalt from the Mid-Atlantic Ridge rift valley near 37N lat. Geol Soc Am Bull. 88, 587–599.
De, A. (1974). Silicate liquid immiscibility in the Deccan Traps and its petrogenetic significance. Geol Soc Am Bull 85, 471–474.
Dick, H. J., Lin, J., and Schouten, H. (2003). An ultraslow-spreading class of ocean ridge. Nature 426 (6965), 405–412. doi:10.1038/nature02128
Hamlyn, P. R., Keays, R. R., Cameron, W. E., Crawford, A. J., and Waldron, H. M. (1985). Precious metals in magnesian low-Ti lavas: implications for metallogenesis and sulfur saturation in primary magmas. Geochim A. T. Cosmochim. Acta 49, 1797–1811. doi:10.1016/0016-7037(85)90150-4
Holzheid, A. (2010). Separation of sulfide melt droplets in sulfur saturated silicate liquids. Chem Geol. 274, 127–135. doi:10.1016/j.chemgeo.2010.03.005
Kamenetsky, V. S., Charlier, B., Zhitova, L., Sharygin, V., Davidson, P., and Feig, S. (2013). Magma chamber-scale liquid immiscibility in the siberian traps represented by melt pools in native iron. Geology 41, 1091–1094. doi:10.1130/G34638.1
Kanehira, K., Yui, S., Sakai, H., and Sasaki, A. (1973). Sulphide globules and sulphur isotope ratios in the abyssal tholeiite from the Mid-Atlantic Ridge near 30N latitude. Geochem J 7 (2), 89–96. doi:10.2343/geochemj.7.89
Keays, R. (1995). The role of komatiitic and picritic magmatism and S-saturation in the formation of ore deposits. Lithos 34, 1–18. doi:10.1016/0024-4937(94)00029-2
Magloughlin, J. F. (2005). Immiscible sulfide droplets in pseudotachylyte: evidence for high temperature (>1200 °C) melts. Tectonophysics 402 (1‒4), 81–91. doi:10.1016/j.tecto.2004.11.011
Mathez, E. A. (1976). Sulfur solubility and magmatic sulfides in submarine basalt glass. J Geophys Res 81, 4269–4276. doi:10.1029/JB081i023p04269
Mathez, E. A., and Yeats, R. S. (1976). Magmatic sulfides in basalt glass from DSDP sites 319A and 320, nazca plate. Initial Reports of the deep-sea drilling project 34.
Mavrogenes, J. A., and O’Neill, H. S. C. (1999). The relative effects of pressure, temperature and oxygen fugacity on the solubility of sulfide in mafic magmas. Geochimical Cosmochimical Acta 63, 1173–1180. doi:10.1016/S0016-7037(98)00289-0
Mazurin, O. V., and Porai-Koshits, E. A. (1984). Phase separation in glass. Amsterdam: North-Holland.
Moore, J. G., and Calk, L. (1971). Sulfide spherules in vesicles of dredged pillow basalt. Am Mineralogist J Earth Planet Mater 56 (3‒4), 476–488.
Moore, J. G., and Schilling, J. G. (1973). Vesicles, water, and sulfur in Reykjanes Ridge basalts. Contrib Mineral Petr 41, 105–118. doi:10.1007/BF00375036
Mungall, J. E., and Naldrett, A. J. (2008). Ore deposits of the platinum-group elements. Elements 4 (4), 253–258. doi:10.2113/GSELEMENTS.4.4.253
Paquet, M., Cannat, M., Brunelli, D., Hamelin, C., and Humler, E. (2016). Effect of melt/mantle interactions on MORB chemistry at the easternmost S outhwest I ndian R idge (61°–67° E). Geochem Geophys Geosystems 17 (11), 4605–4640. doi:10.1002/2016GC006385
Patten, C., Barnes, S. J., and Mathez, E. A. (2012). Textural variations in morb sulfide droplets due to differences in crystallization history. Can Mineralogist 50, 675–692. doi:10.3749/canmin.50.3.675
Patten, C., Barnes, S. J., Mathez, E. A., and Jenner, F. E. (2013). Partition coefficients of chalcophile elements between sulfide and silicate melts and the early crystallization history of sulfide liquid: LA-ICP-MS analysis of MORB sulfide droplets. Chem Geol 358, 170–188. doi:10.1016/j.chemgeo.2013.08.040
Peach, C. L., Mathez, E. A., and Keays, R. R. (1990). Sulfide melt-silicate melt distribution coefficients for noble metals and other chalcophile elements as deduced from MORB: implications for partial melting. Geochimica Cosmochimica Acta 54, 3379–3389. doi:10.1016/0016-7037(90)90292-S
Philpotts, A. R. (1972). Density, surface tension and viscosity of the immiscible phase in a basic, alkaline magma. Lithos 5, 1–18. doi:10.1016/0024-4937(72)90076-X
Philpotts, A. R. (1982). Compositions of immiscible liquids in volcanic rocks. Contrib Mineral Petr 80, 201–218. doi:10.1007/BF00371350
Rehkämper, M., Halliday, A. N., Fitton, J. G., Lee, D. C., Wieneke, M., and Arndt, N. T. (1999). Ir, Ru, Pt, and Pd in basalts and komatiites: new constraints for the geochemical behavior of the platinum-group elements in the mantle. Geochimical Cosmochimical Acta 63, 3915–3934. doi:10.1016/S0016-7037(99)00219-7
Robertson, J. C., Barnes, S. J., and Le Vaillant, M. (2015). Dynamics of magmatic sulphide droplets during transport in silicate melts and implications for magmatic sulphide ore formation. J Petrology 56 (12), 2445–2472. doi:10.1093/petrology/egv078
Roedder, E., and Weiblen, P. W. (1970). “Lunar petrology of silicate melt inclusions, Apollo 11 rocks,” in Geochimica et Cosmochimica Acta Supplement.: Mineraolgy and Petrology (New York: Pergammon Press), Vol. 1, 801.
Skinner, B. J., and Peck, D. L. (1969). An immiscible sulfide melt from Hawaii. Econ. Geol. Monogr. 4, 310–322.
Song, X. Y., Zhou, M. F., Keays, R. R., Cao, Z. M., Sun, M., and Qi, L. (2006). Geochemistry of the emeishan flood basalts at yangliuping, sichuan, SW China: implications for sulfide segregation. Contribution Mineralogy Petrology 152, 53–74. doi:10.1007/s00410-006-0094-3
Thompson, A. B., Aerts, M., and Hack, A. C. (2007). Liquid immiscibility in silicate melts and related systems. Rev Mineralogy Geochem 65, 99–127. doi:10.2138/rmg.2007.65.4
Vakhrushev, V. A., and Prokoptsev, N. G. (1972). Primary magmatic formations in basalts from oceanic crust and inclusions of ultrabasic rocks in basalts. Int Geol Rev 14 (2), 125–135. doi:10.1080/00206817209475675
Veksler, I. V., Dorfman, A. M., Borisov, A. A., Wirth, R., and Dingwell, D. B. (2008). Liquid immiscibility and evolution of basaltic magma: reply to SA Morse, AR McBirney and AR Philpotts. J Petrology 49, 2177–2186. doi:10.1093/petrology/egn064
Wallace, P. J., and Edmonds, M. (2011). The sulfur budget in magmas: evidence from melt inclusions, submarine glasses, and volcanic gas emissions. Rev Mineralogy Geochem 73 (1), 215–246. doi:10.2138/rmg.2011.73.8
Yang, A. Y., Zhao, T. P., Zhou, M. F., Deng, X. G., Wang, G. Q., and Li, J. (2013). Os isotopic compositions of MORBs from the ultra-slow spreading Southwest Indian Ridge: constraints on the assimilation and fractional crystallization (AFC) processes. Lithos 179, 28–35. doi:10.1016/j.lithos.2013.07.020
Yang, A. Y., Zhou, M. F., Zhao, T. P., Deng, X. G., Qi, L., and Xu, J. F. (2014). Chalcophile elemental compositions of MORBs from the ultraslow-spreading Southwest Indian Ridge and controls of lithospheric structure on S-saturated differentiation. Chem Geol 382, 1–13. doi:10.1016/j.chemgeo.2014.05.019
Keywords: MORB, sulfide melt, silicate melt, TEM, SEM
Citation: Zuo L, Zhang P, Wang Y-q and Liu R (2024) A discovery of nanoscale sulfide droplets in MORB glasses: implications for the immiscibility of sulfide and silicate melts. Front. Earth Sci. 12:1403504. doi: 10.3389/feart.2024.1403504
Received: 19 March 2024; Accepted: 02 July 2024;
Published: 31 July 2024.
Edited by:
Hongye Feng, Chinese Academy of Geological Sciences, ChinaReviewed by:
Fernando Gervilla, University of Granada, SpainCopyright © 2024 Zuo, Zhang, Wang and Liu. This is an open-access article distributed under the terms of the Creative Commons Attribution License (CC BY). The use, distribution or reproduction in other forums is permitted, provided the original author(s) and the copyright owner(s) are credited and that the original publication in this journal is cited, in accordance with accepted academic practice. No use, distribution or reproduction is permitted which does not comply with these terms.
*Correspondence: Rui Liu, bGl1cnVpQHNkdXQuZWR1LmNu
Disclaimer: All claims expressed in this article are solely those of the authors and do not necessarily represent those of their affiliated organizations, or those of the publisher, the editors and the reviewers. Any product that may be evaluated in this article or claim that may be made by its manufacturer is not guaranteed or endorsed by the publisher.
Research integrity at Frontiers
Learn more about the work of our research integrity team to safeguard the quality of each article we publish.