- 1Institute of Hydrogeology and Environmental Geology, Chinese Academy of Geological Sciences, Shijiazhuang, China
- 2Technology Innovation Center for Geothermal & Hot Dry Rock Exploration and Development, Ministry of Natural Resources, Shijiazhuang, China
The Huangshadong geothermal field (HGF), situated in the contact zone between Mesozoic granites and NE-striking dominant faults in South China, has great geothermal potential. Petrogenesis of reservoir rock plays an important role in understandings its genetic mechanism and assessing geothermal potential. However, due to the lack of rock sample at depth collected from the geothermal reservoir, the petrogenesis of granites in the geothermal reservoirs of the HGF, remains an enigma. This study elucidated the petrogenetic characteristics of these granites sampled directly from geothermal reservoir at the depth of ∼3,000 km and their geothermal implications through zircon U-Pb dating, geochemical analysis, and Hf isotopic analysis. The zircon U-Pb ages indicate that the magmatism evolution of HGF contains three eras, namely, Cretaceous (135 ± 4 to 143.6 ± 2.8 Ma), Jurassic (152.7 ± 2.7 to 176.7 ± 1.8 Ma), and Permian granites (251 ± 9.1 to 251 ± 5 Ma) from the youngest to oldest. The reservoir granites were emplaced during the latest stage of Cretaceous intrusion, as indicated by the zircon U-Pb ages (135 ± 4 Ma and 135.3 ± 2.4 Ma) of rock samples from the deep part of well HR-1. These Cretaceous rocks are highly fractionated I-type granites, featuring high SiO2, K2O, and Na2O contents, high Rb/Sr ratios, low Zr/Hf, Nb/Ta, and Th/U ratios, and A/CNK values of 1.05–1.13. Compared to other Cretaceous granites outcropping on the margin of the HGF, these granites have undergone the strongest fractional differentiation. The Cretaceous granites in the HGF are high-heat-producing rocks (>5 μW/m3), with an average heat production rate of 6.63 μW/m3. Notably, the Cretaceous reservoir granites (as reservoir rocks) serve as an important heat source for the formation of geothermal resources in the HGF. In addition, the zircon Hf isotopic composition indicates that the reservoir Cretaceous granites originated from Meso-to Paleo-Proterozoic lower crustal materials (TDM2: 1,385 to 1907 Ma).
1 Introduction
Geothermal resources have attracted wide attention worldwide owing to their clean and sustainable nature (Tester et al., 2006; Lin et al., 2016; Lu, 2018; Lin et al., 2022; Ma et al., 2022; Chen et al., 2023). The geothermal energy utilized directly was up to 107,727 MWt in 2020, increasing by 52% since 2015 (Lu, 2018; Lund and Toth, 2021). The petrological characteristics of geothermal reservoir rocks serve as key parameters used to evaluate the geothermal potential and analyze the genesis of geothermal resources, especially for a granite-hosted geothermal system (Zhang et al., 2022; Alqahtani et al., 2023a; Alqahtani et al., 2023b; Ullah et al., 2023; Wang et al., 2023).
The heat for thermal anomalies originates from the radioactive decay of heat-producing elements (U, Th, and K) (Hasterok and Chapman, 2011; Wang et al., 2023). The upper crust has average U, Th, and K2O contents of 2.7 ppm, 10.5 ppm, and 2.8%, respectively, with a radioactive heat production rate of 1.75 μW/m3 (Kemp and Hawkesworth, 2003; Kromkhun, 2010). In contrast, the granites are relatively rich in incompatible elements such as U, Th, and K, with average contents of 3.9 ppm, 16 ppm, and 3.6%, respectively. This enrichment results in a radioactive heat production rate of 2.53 μW/m3. Due to their incompatibility, these heat-producing elements are usually concentrated in the shallow crust, diminishing with depth. The granitic upper crust has depths of mostly 10–20 km, and its radioactive heat production contributes surface heat flow of 10–20 μW/m2, which accounts for 50% of the surface heat in the stable continental crust (Artemieva and Thybo, 2013). High-heat-producing granites, with radioactive heat production rates >5 μW/m3, play a critical role in the formation of geothermal resources (Marshall, 2014; Artemieva et al., 2017). For instance, the Cooper Basin, which is subjected to the most recent intrusion of Carboniferous-Permian granodiorites rather than present-day magmatic events (Big Lake Suite, 310–327 Ma; (Marshall, 2014), records the terrestrial heat flow values measuring >100 mW/m2. However, the minimum heat flow value is only 33 mW/m2, which occurs at the edge of the rock mass in a borehole. This finding indicates that the temperature anomalies are primarily derived from the high-heat-producing granites in the basement, which have a maximum radioactive heat production rate of up to 10 μW/m3 (Roth and Littke, 2022).
A large quantity of Mesozozic granites in South China are genetically related to geothermal energy (Lin et al., 2016; Qiu et al., 2018; Liu et al., 2021; Lin et al., 2022; Lin et al., 2023; Pang and Huang, 2023). The granites in South China have an average heat production rate of 3 μW/m3 (Zhao and Luo, 1995). Among them, granites in Guangdong Province have an average heat production rate of 5.7 μW/m3, classified as high-heat-producing rocks. Many thermal springs are distributed in the HGF, with temperatures of 56–63.7°C and a flow rate of 0.38 L/s (Lin et al., 2016). Geothermal wells ZK 8 and HR-1 revealed high temperatures of 118.3°C and 127.5°C, respectively at depths of 591.5 m and 2,900 m (Xiao et al., 2019; Li et al., 2020a).
Previous studies of granites in the HGF focus on their regional petrogeological characteristics, subjected to rock samples collected from outcrops or shallow buried plutons (∼300 m below the surface; Xiao et al., 2019). Most of the samples were collected from outcrops at a great distance from HGF (G1, G2, G3, and G5 in Figure 2). As a result, the petrogenesis of geothermal reservoir granites buried at depth in the areas where a bunch of hot springs are exposed (locations of wells HR-1 and ZK8), as well as the genetic relationship between them and geothermal energy, remains unclear. This study conducted geochronological, geochemical, and Hf isotopic analyses of granites in the HGF (especially the reservoir granites dredged by HR-1 from the depth of ∼3,000 km), aiming to promote the understanding of the genetic relationship between the geothermal resources and high-heat-producing granites.
2 Geological setting
The South China Block (SCB) was formed by the amalgamation of the Yangtze Block in the northwest and the Cathaysia Block in the southeast during 1.0–0.9 Ga (Zhou et al., 2006) (Figure 1). The NE-striking Qinzhou Bay - Hangzhou Bay (Qin-Hang) suture zone between the latter two blocks was reactivated during the Middle-Late Mesozoic, leading to the formation of numerous plutons (Li et al., 2009; Mao et al., 2011; Hu et al., 2017). Regionally, there are six primary faults striking NE to NEE, namely, the Jiangshan-Shaoxing-Pingxiang, Zhenghe-Dapu, Changle-Nan’ao, Chenzhou-Linwu faults, and the Changlin-Guangchang and the Wuzhou-Sihui concealed faults. The HGF is located approximately 20 km northeast of Huizhou City, Guangdong Province (Figure 1).
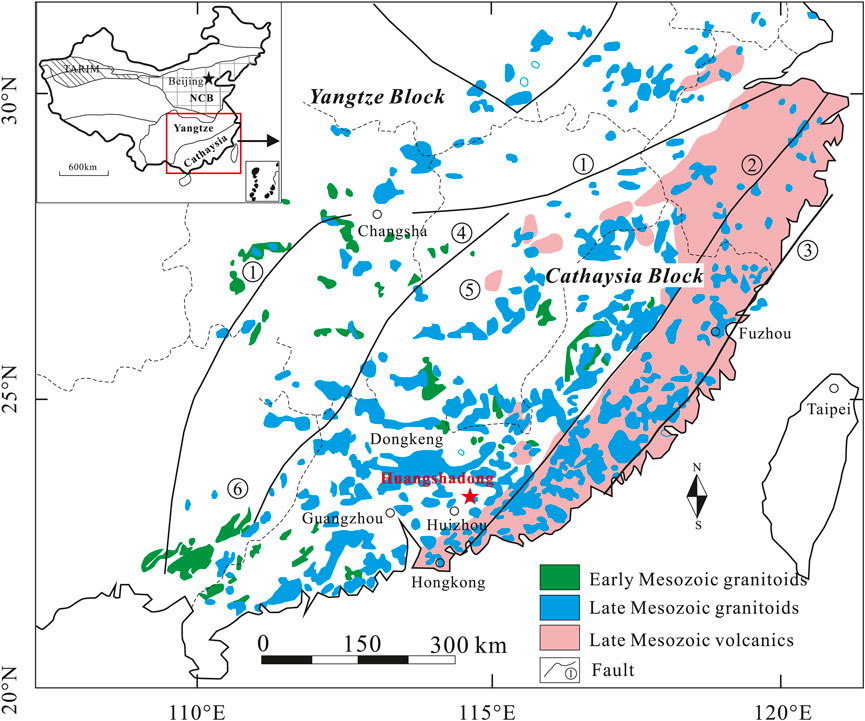
FIGURE 1. Geological map of the Nanling Range, South China (modified after Chen et al. (2014), copyright@Elsevier, 2016). Faults: (1) Jiangshan-Shaoxing-Pingxiang Fault; (2) Zhenghe-Dapu Fault; (3) Changle-NanaoFault; (4) Chenzhou-Linwu Fault; (5) Changlin-Guangchang buried Fault; (6) Wuzhou-Sihui buried Fault. NCB: North China Craton. TARIM: Tarim Basin.
The stratigraphy in the HGF consists primarily of Sinian and Cambrian metasedimentary strata, as well as Devonian, Carboniferous, Triassic, and Quaternary sedimentary strata (Figure 2). The Sinian metasedimentary strata contain two formations, namely, Laohukuang (Zlh, 725 m thick) and Bali (Zb, >500 m thick). The former is located in the eastern part of this gas field, comprising metasandstones and slates. In contrast, the latter is present in the northwestern part of the study area, consisting of metasandstones interbedded with slates. The Cambrian metasedimentary strata (Єn, 600 m thick) comprise metasandstones occasionally interbedded with phyllites. The Devonian sedimentary strata include the Yangxi (Dy, 370–750 m thick), Laohutou (Dl, 470–620 m thick), and Maozifeng (DCm, 65–410 m thick) formations. They occur in the southeastern part, comprising conglomerates, sandstones, and mudstones. The Carboniferous sedimentary strata are mainly present in the southwestern parts, consisting of three formations, namely, Dasaiba (Cds, 340–400 m thick), Ceshui (Cc, 300–400 m thick), and Hutian (CH, >150 m thick). Among them, the Dasaiba and Ceshui formations comprise quartz sandstones and slates, while the Hutian Formation is composed of limestones and dolomites. The Triassic Genkou Group (TG >1,250 m thick), which consists of conglomerates, sandstones, and mudstones, is found at the eastern and western edges of the HGF. The Quaternary sedimentary strata (Qdw, 10–25 m thick), primarily comprising clay, are distributed in the southwestern part.
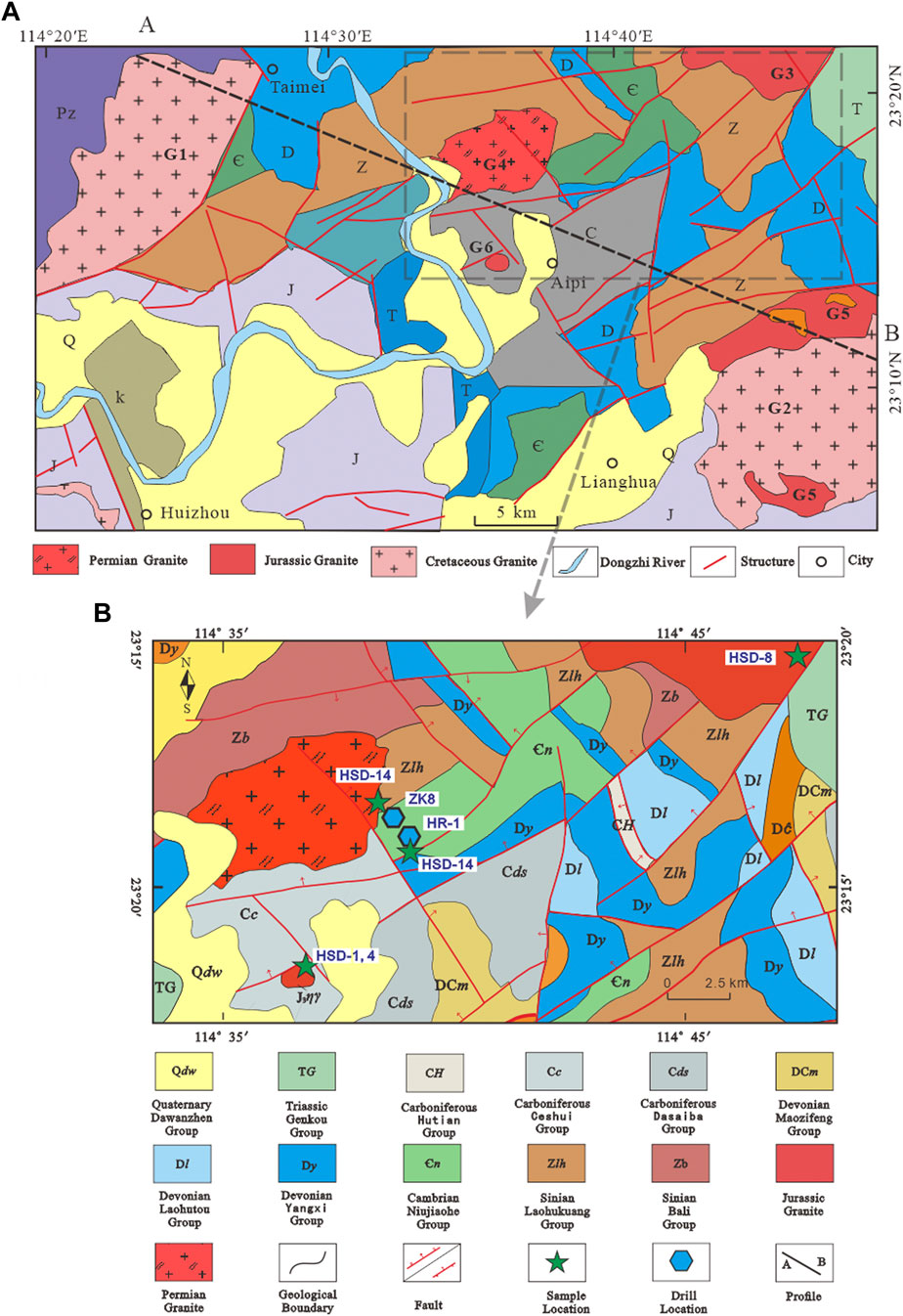
FIGURE 2. (A) Geological sketch maps of the Huangshadong geothermal field (modified after Xiao et al., 2019). Pz = Paleozoic; Z = Sinian; Є = Cambrian; D = Devonian; C = Carboniferous; T = Triassic; J = Jurassic; K = Cretaceous; Q = Quaternary. (B) Location of geothermal wells and samples in the Huangshadong geothermal field (modified after Zuo et al., 2018).
Spatially, thermal springs in Guangdong Province are closely associated with the outcrops of intrusions (especially Yanshanian intrusions), mostly found in the contact zone between intrusions (granite complex) and host rocks (Xiao et al., 2019).
Granite complex in the HGF comprising three parts (Figure 2): 1) Cretaceous granite, dominant geothermal reservoir rock; 2) Jurassic granite, occurring in the northeastern part; 3) Permian granites exposed only in the central part. Geothermal surface manifestations occur in the contact zone between the Permian granites and the Cambrian metasedimentary rocks. Cretaceous granites were excavated at a depth of about 300 m by well ZK8 (Xiao et al., 2019), and concealed granites were revealed at a depth of 1,560 m by geothermal well HR-1 (Li et al., 2020a).
3 Sampling and petrography
Seven granite samples, namely, HSD-1, HSD-4, HSD-8, HSD-14, HZ-1-1, HZ-3-1, and HZ-3-2), were collected from the HGF for dating and geochemical analysis (Figure 3; Table 1). Each sample was 5–10 kg in weight. Samples HSD-1, HSD-4, HSD-8, and HSD-14 were taken from granite outcrops (Figures 2, 3). Among them, samples HSD-1 and HSD-4 were fresh massive rocks obtained from a strip pit in the southwestern part of the HGF, while sample HSD-8 was gathered from the northeastern part. Samples HSD-1 and HSD-8 were medium-to coarse-grained equigranular biotite granites, and sample HSD-4 consisted of fine-grained equigranular biotite granites. Sample HSD-14 was collected from the two-mica granite outcrops near geothermal well HR-1, with its weathered parts chipped off using a hammer. Samples HZ-1, HZ-3-1, and HZ-3-2 were collected at depths of 1831 m, 2,637 m, and 2,702 m, respectively in well HR-1 (Figure 3).
Fresh field samples were cut into blocks of ∼45×25×15 mm using a diamond blade. Afterward, they were planed and then mounted on a 28×48 mm standard petrographic slide using epoxy. The thin sections measured 30 μm in thickness after being polished with abrasive powder. To identify the mineralogy and texture of rocks, the polished thin sections were examined under a binocular microscope and a Leica DM2700P polarization microscope at the Institute of Hydrogeology and Environmental Geology under the Chinese Academy of Geological Sciences.
Figure 4 shows the microscopic photographs of the samples. Samples HSD-1 and HSD-8 were medium-to coarse-grained equigranular biotite granites, which contained quartz (∼37 vol.%), plagioclase (∼30 vol.%), alkali feldspar (∼23 vol.%), biotite/muscovite (∼2 vol.%), and accessory minerals (<3 vol.%), including zircon and apatite. Sample HSD-4 exhibited similar petrological features with samples HSD-1 and HSD-8, except for smaller grain sizes (fine-grained: 0.1–0.3 mm). Samples HZ-1 and HZ-3 were medium-to fine-grained equigranular biotite granites, which contained quartz (∼42 vol.%), plagioclase (∼29 vol.%), alkali feldspar (∼23 vol.%), biotite (∼2 vol.%), and muscovite (∼1 vol.%). Furthermore, zircon is a common accessory mineral in these samples. In contrast, sample HSD-14 was medium-grained two-mica granite, which consisted of quartz (∼40 vol.%), plagioclase (∼30 vol.%), alkali feldspar (∼21 vol.%), biotite (∼2 vol.%), and muscovite (∼2 vol.%).
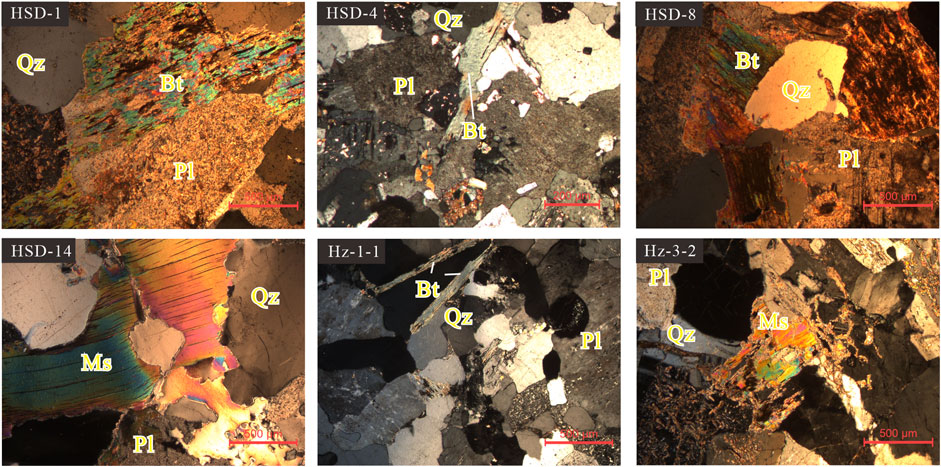
FIGURE 4. Microscope photographs of the granites in the Huangshadong geothermal field. Abbreviations: Qz = Quartz, Pl = Plagioclase, Bt = Biotite, Ms = Muscovite.
4 Methods
4.1 LA-ICP-MS U-Pb dating and Hf isotopes of zircon
This study conducted zircon dating of granite samples HSD-1, HSD-4, HSD-8, HSD-14, HZ-1, and HZ-3. These six samples (∼5 kg each) were processed at the Hebei Institute of Regional Geology and Mineral Resources Survey.
Six zircon grains were separated from these samples using conventional heavy-liquid and magnetic separation techniques. Then, about 100 zircon grains with the highest quality were hand-picked under a binocular microscope. These grains were mounted in epoxy and then polished. Afterward, the grains were photographed using optical microscopy and cathodoluminescence (CL) imaging to reveal their internal morphologies, which were used to select grains and analytical spots. CL images were obtained using a HITACHI S3000-N scanning electron microscope equipped with a Robinson backscattered electron detector and a Gatan Chroma CL imaging system.
To determine zircon U-Pb ages, these above samples were analyzed using an Agilent 7,900 quadrupole ICP-MS equipped with a 193 nm coherent Ar-F laser and a Resonetics S155 ablation cell at the Tianjin Institute of Geology and Mineral Resources. NIST SRM 610 glass used for Pb correction was analyzed after every 15 test samples. The mass bias of Th/U and Pb/Th ratios caused by downhole fractionation and instrumental drift was corrected using zircon reference material 91,500 following instructions of Wiedenbeck et al. (1995). Each zircon analysis involved 15 s blank gas measurement and 50 s analysis. The analytical spots were 29 μm each in size, and laser was emitted at a frequency of 7 Hz, yielding an energy density of approximately 3 J/cm2. The grains ablated by laser were carried by He gas into the chamber at a flow rate of 0.35 L/min, where they were mixed with argon gas before being transported to the plasma torch. Zircon standards Temora (Black et al., 2003) and Plesovice (Sláma et al., 2008) were employed in this study. Data processing was performed using Concordia intercept ages on the Tera-Wasserburg plot which was prepared using ISOPLOT (Ludwig, v. 3.75, 2012). Data reduction was conducted using ICPMSDataCal (Liu et al., 2010). The random and systematic uncertainties were estimated using the method proposed by Paton et al. (2010).
Based on LA-ICP-MS zircon U-Pb dating, the cathodoluminescence (CL) diagram of zircon was plotted. The laser denudation was conducted under a beam spot diameter of 40 μm and a duration of 30 s, with GJ-1 used as the external reference material for the calculation of Hf (Geng et al., 2011). In the solution injection mode, a 200×10−9 standard solution was used. It was fed into a nebulizer at a flow rate of 50 μL/min using a peristaltic pump. Nine Faraday cups simultaneously received 172Yb, 173Yb, 175Lu, 176(Yb+Hf+Lu), 177Hf, 178Hf, 179Hf, 180Hf, and 182W. Data acquisition was performed using the virtual amplifier technology of NEPTUNE (MC-ICPMS). The software automatically replaced the amplifier circuits of the analyzer after the acquisition of a set of data. After nine sets of data were acquired, the amplifier circuits were identical to those of the original analyzer. This technique can be used to effectively eliminate the isotope ratio error caused by the difference in the gain of each Faraday cup receiver, thus improving the accuracy of isotope ratio determination.
The laser ablation test began with the targeting, polishing, and photographing of the staghornite to be tested. Samples were arranged on the double-sided adhesive of the slide and put on the PVC ring. Then, epoxy resin and hardener were mixed thoroughly and injected into the PVC ring. After the resin was fully cured, sample holders were peeled off from the slide and then polished, followed by the taking of the cathodoluminescence, reflected light, and transmitted light photographs of the samples on the target. Based on these three kinds of photographs, the appropriate area of the struvite was selected, and the struvite was stripped using a 193 nm FX laser with a spot diameter of 35, 50, or 75 (um), an energy density of 10–11 J/cm2, and a frequency of 8–10 Hz. The laser-stripped materials were fed into the Neptune (MC-ICPMS) with high-purity He as the carrier gas. The receiver configuration was the same as that of the solution injection.
4.2 Whole-rock geochemical analyses
Seven fresh samples were cut into blocks, each measuring ∼70×50×30 mm in size. These blocks were washed with tap water and ground to <200 meshes using an agate mill. Both major and trace element analyses were performed using the method proposed by Liu et al. (2016) at the Central Laboratory of China Railway Resources Group. The contents of major elements were measured using an X-ray fluorescence (XRF) spectrometer (Thermo Fisher ARL Advant'X), with the loss on ignition (LOI) being determined with an electronic analytical balance (CPA225D). The FeO content in the samples was measured using conventional wet chemical titration. The contents of trace elements, including rare earth elements (REEs), were determined using ICP-MS (Thermo Fisher X-series 2) after complete digestion. A detailed account of the test procedures and the lower detection limit were documented in Chinese national standard GB/T14506-2010 (Lv, 2010). The analytical errors varied in a range of 1%–3% of the present values. The whole-rock geochemical analyses were conducted under temperatures of 20–27°C and humidity of 30%–58%.
5 Results
5.1 Zircon dating
As presented in Figure 5, zircons from sample HZ-1 were typically transparent, ranging from colorless to slightly brown, and exhibited rectangular to prismatic crystal structures. They were 80–180 μm long, with aspect ratios ranging from 1.5:1 to 3:1. Furthermore, these crystals commonly exhibited oscillatory zoning (Figure 5). Zircons from sample HZ-3 were predominantly transparent, clear to pale yellow, and euhedral to subhedral crystals. They were 70–170 μm long, with aspect ratios varying in the range of 2:1–3:1. Among them, euhedral grains showed concentric zoning with relatively bright cores, as shown in CL images (Figure 5). Zircons from samples HSD-1 and HSD-8 were similar in shape and color. They were slightly larger (typical length: 100–200 μm), with aspect ratios between 1:1 and 3:1, and exhibited weakly oscillatory zoning. Furthermore, zircons from sample HSD-4 were considerably smaller (length: 40–100 μm), with aspect ratios of 1:1–4:1.
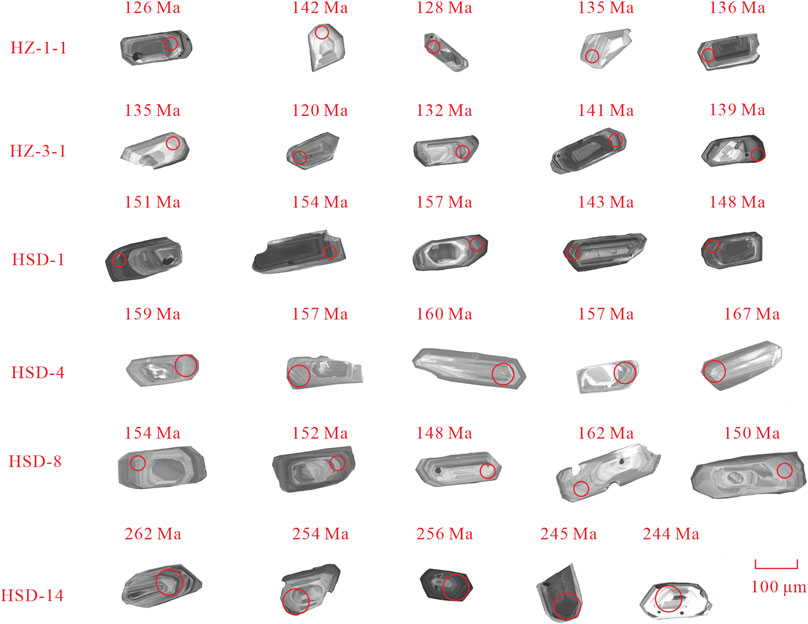
FIGURE 5. Cathodoluminescence images of zircons with corresponding 206Pb/238U ages for the samples from the Huangshadong geothermal field.
The LA-ICP-MS zircon U-Pb ages of the HGF are shown in Figure 6; Table 2. Fifteen analyses showed that sample HZ-1 had a238U/206Pb age of 135 ± 4 Ma (MSWD = 5.7), while 20 analyses revealed that sample HZ-3 had a weighted average 238U/206Pb age of 135.3 ± 2.4 Ma (MSWD = 3.2). The two ages, which were consistent within the given error range, indicate that both samples formed during the Cretaceous. In contrast, samples HSD-1, HSD-4, and HSD-8 exhibited significantly older weighted average 238U/206Pb ages of 152.7 ± 2.7 Ma (MSWD = 4.8), 155.8 ± 3.3 Ma (MSWD = 5.2), and 153 ± 3 Ma (MSWD = 3.8), respectively. These ages, which were also consistent within the given error range, suggest that these three samples intruded during the Jurassic. Sample HSD-14 was emplaced during the Indosinian, as indicated by four data points yielding a238U/206Pb age of 153.7 ± 1.2 (MSWD = 2.2; probability = 0.002). As shown in Figure 7; Table 3, sample HSD-14 exhibited the highest Ti-in-zircon temperatures from 684°C to 781°C (Ferry and Watson, 2007), with an average of 742°C, while the other samples had lower Ti-in-zircon temperatures, with averages ranging from 617°C to 687°C.
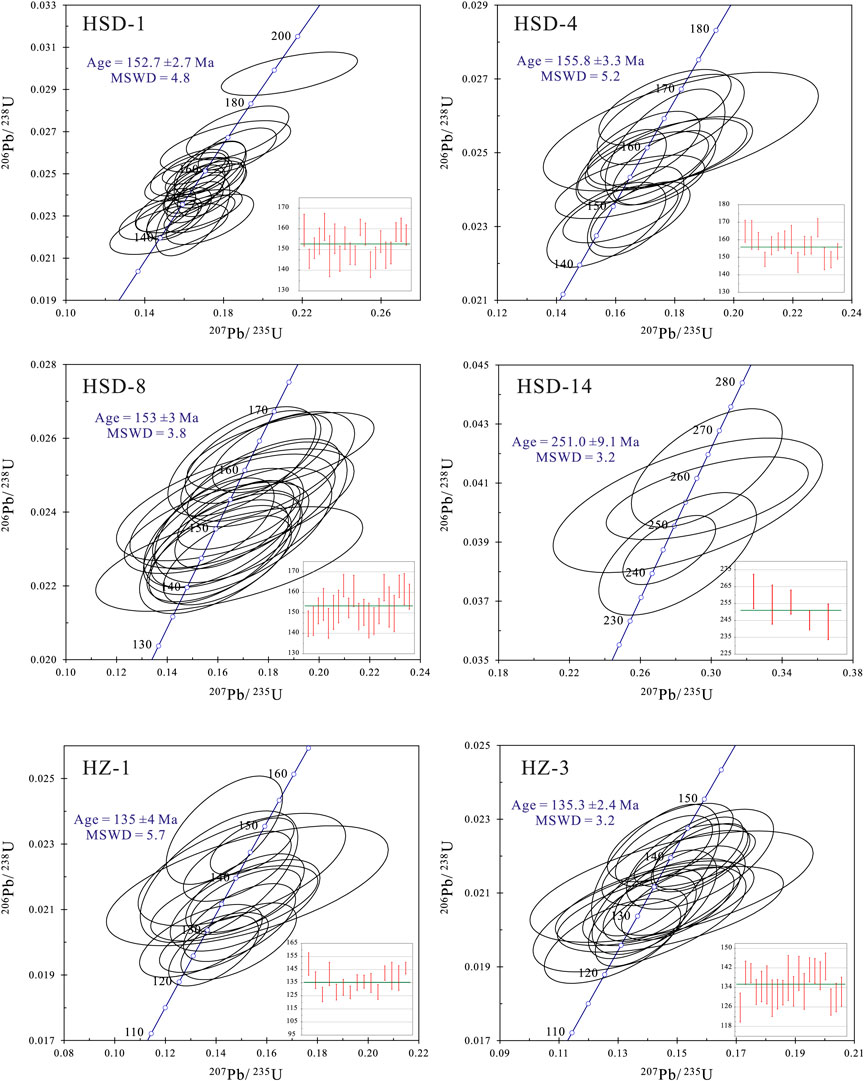
FIGURE 6. Zircon LA-ICP-MS U–Pb concordia diagrams for the Huangshadong geothermal field. Data processing was carried out using concordia intercept ages on the Tera-Wasserburg plot utilizing ISOPLOT.
5.2 Geochemical characteristics
We analyzed the major and trace element compositions of seven representative samples (Table 5). These samples featured high SiO2 (74.4–76.56 wt.%) and K2O (3.76–4.84 wt.%) contents. All the samples fell within the high-K calc-alkaline zone (Figure 8A). Samples HSD-4, HSD-8, and HZ-1 exhibited aluminum saturation index (ASI) values of 1.02, 1.05, and 1.05, respectively, all of which were less than 1.1 (Figure 8B). In comparison, samples HSD-1, HZ-3-1, and HZ-3-2 had ASI values of 1.13, 1.13, and 1.11, respectively, all of which were greater than 1.1.
Compared to the other samples, samples HSD-1, HSD-8, and HSD-14 had higher K2O, CaO, MgO, TiO2, Zr, Sr, and Ba contents but lower Na2O and Al2O3 contents (Figures 8–11; Table 5). All these samples were depleted in P2O5 (Figure 11).
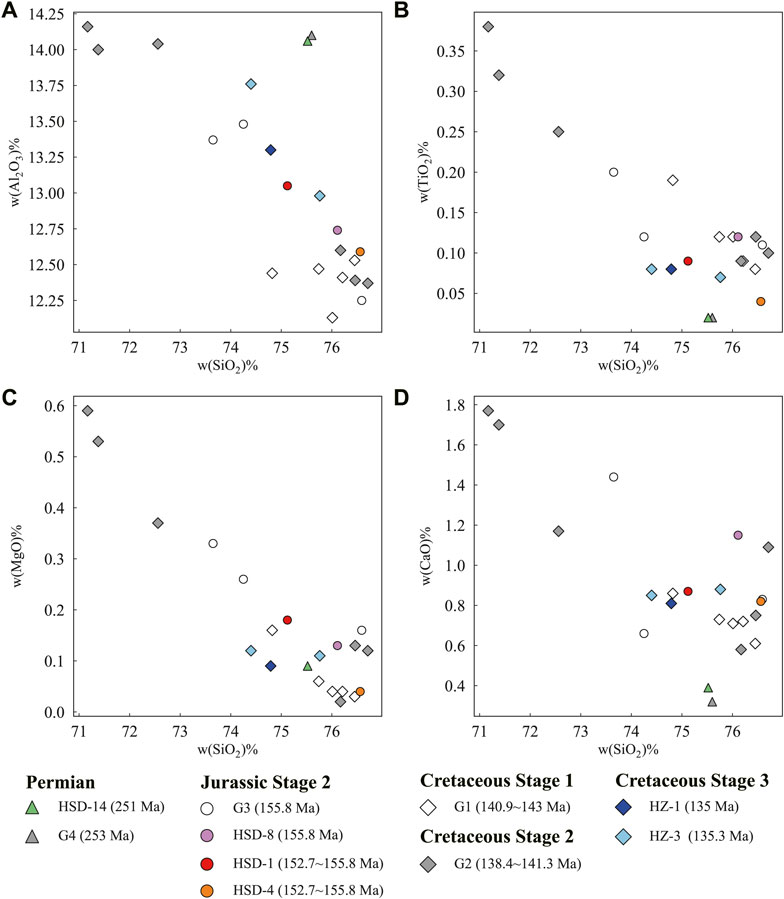
FIGURE 9. Harker diagram of the granites in the Huangshadong geothermal field (A) SiO2 vs. Al2O3; (B) SiO2 vs. TiO2; (C) SiO2 vs. MgO; (D) SiO2 vs. CaO.
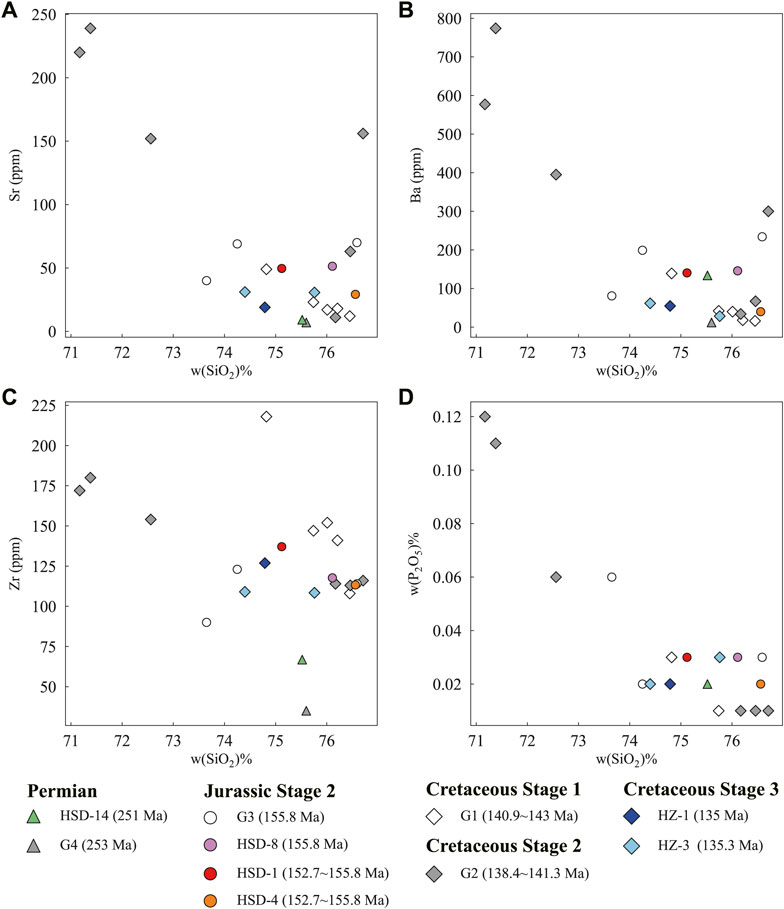
FIGURE 10. SiO2 vs. Sr (A), Ba (B), Zr (C), P2O5 (D) for the granites in the Huangshadong geothermal field.
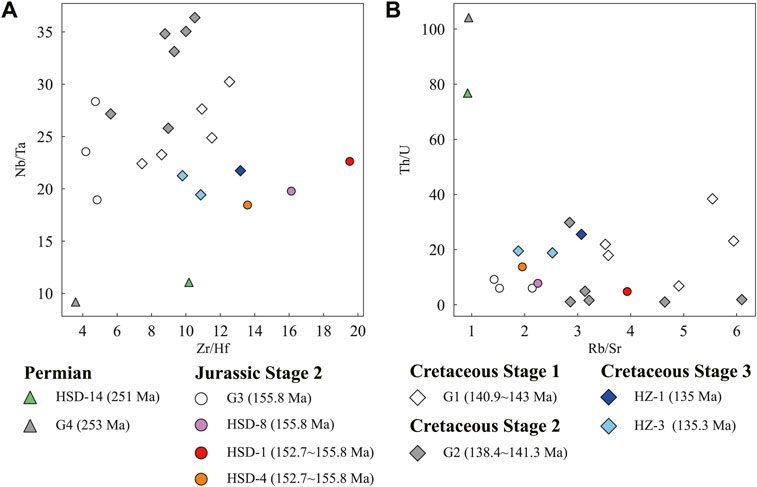
FIGURE 11. Zr/Hf vs Nb/Ta (A), Rb/Sr vs Th/U (B) for the granites in the Huangshadong geothermal field.
As shown in the primitive mantle-normalized diagrams (Figure 12; Table 5), sample HSD-14 exhibited the strongest depletion in Ti, Sr, La, and Ce but notable enrichment in Rb, U, and Nd. Compared with samples HSD-1 and HSD-8, samples HSD-4, HZ-1, and HZ-3 were relatively depleted in Ti, Sr, and Ba but enriched in Rb, U, Nb, and Nd.
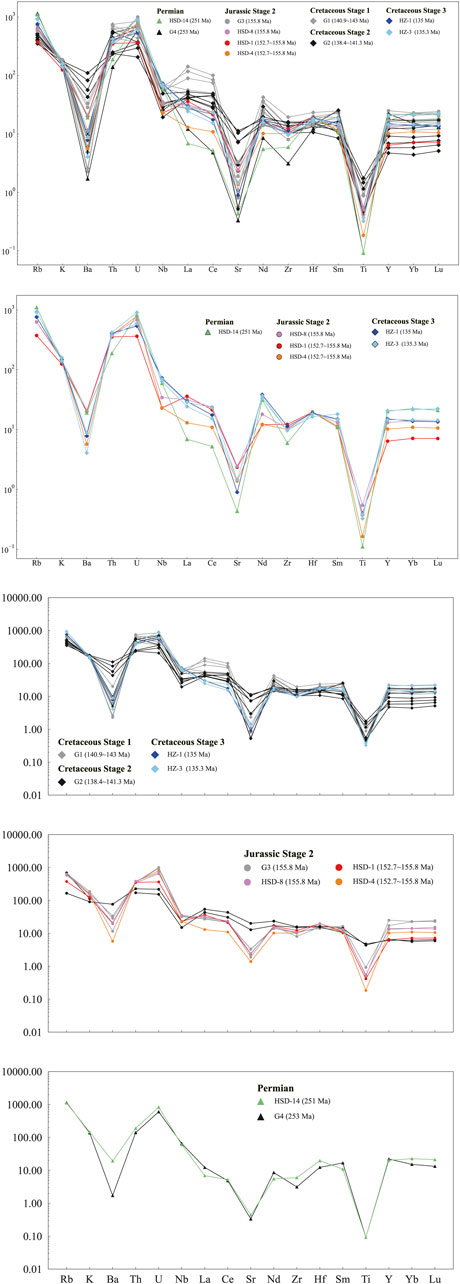
FIGURE 12. Primitive mantle-normalized trace element spider diagrams of granites in the Huangshadong geothermal field (Sun and McDonough, 1989).
The REE patterns (Figure 13; Table 5) showed that sample HSD-14 exhibited a leftward REE pattern with the lowest LaN/YbN ratio (0.56); samples HZ-3-1 and HZ-3-2 showed relatively flat patterns with similar LaN/YbN ratios of 1.47 and 1.32, respectively; sample HZ-1 had a much higher LaN/YbN ratio of 9.7; among the remaining samples, HSD-1 displayed the highest LaN/YbN ratio (5.06), followed by HSD-8 (2.95), while HSD-4 had the lowest LaN/YbN ratio (1.19); samples HSD-4, HSD-14, HZ-3-1, and HZ-3-2 exhibited the lowest δEu values, which were 0.1, 0.1, 0.09, and 0.07, respectively, while sample HSD-1 had the highest δEu value, which was 0.32. The Indosinian granite (sample HSD-14) exhibited relatively low total REE contents (ΣREE; 78.38). In contrast, the Cretaceous granites showed the highest ΣREEs, with values of 188.55 ppm, 111.12 ppm, and 149.78 ppm for samples HZ-1, HZ-2, and HZ-3, respectively.
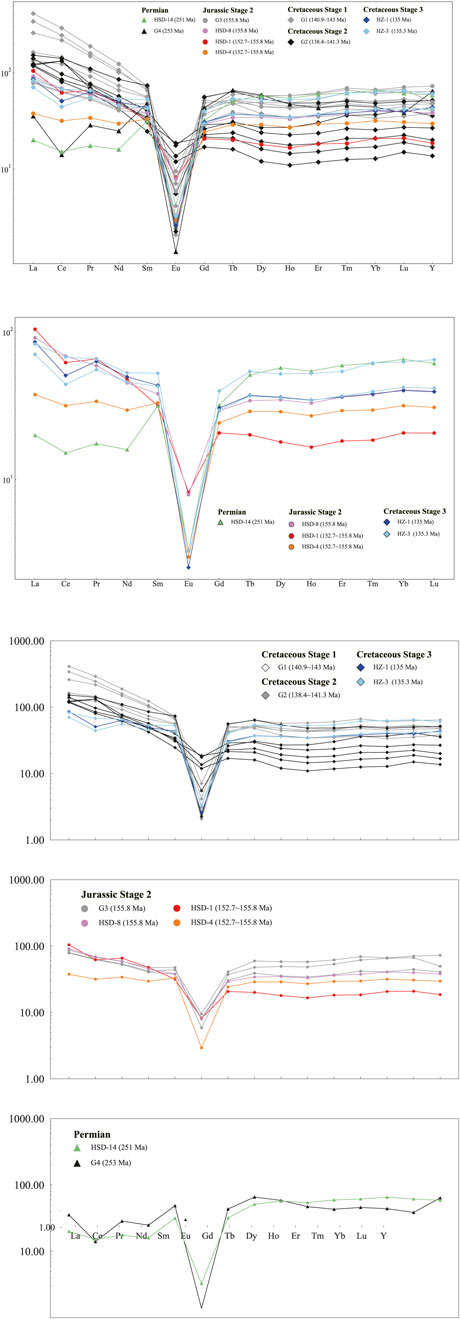
FIGURE 13. Chondrite-normalized REE spider diagrams of granites in the Huangshadong geothermal field (Sun and McDonough, 1989).
5.3 Hf isotopes
This study conducted the Lu-Hf isotope analyses for zircons that were subjected to U-Pb dating, as shown in Table 6; Figure 14. Specifically, Lu-Hf isotope analysis was conducted on 15 and 20 zircon grains from HZ-1 and HZ-3, respectively. These zircons show a wide range of176Yb/177Hf ratios from 0.017479 to 0.126740 (average: 0.050829) and 176Lu/177Hf ratios between 0.000509 and 0.003495 (average: 0.00132), indicating low content of radiogenic Hf. Furthermore, 35 analytical spots yielded εHf(t) values of −11.4334 to −3.0396, which corresponded to two-stage Hf model ages (TDM2) of 1,385–1907 Ma dominated by 1,500–1700 Ma, as depicted in Figure 15.
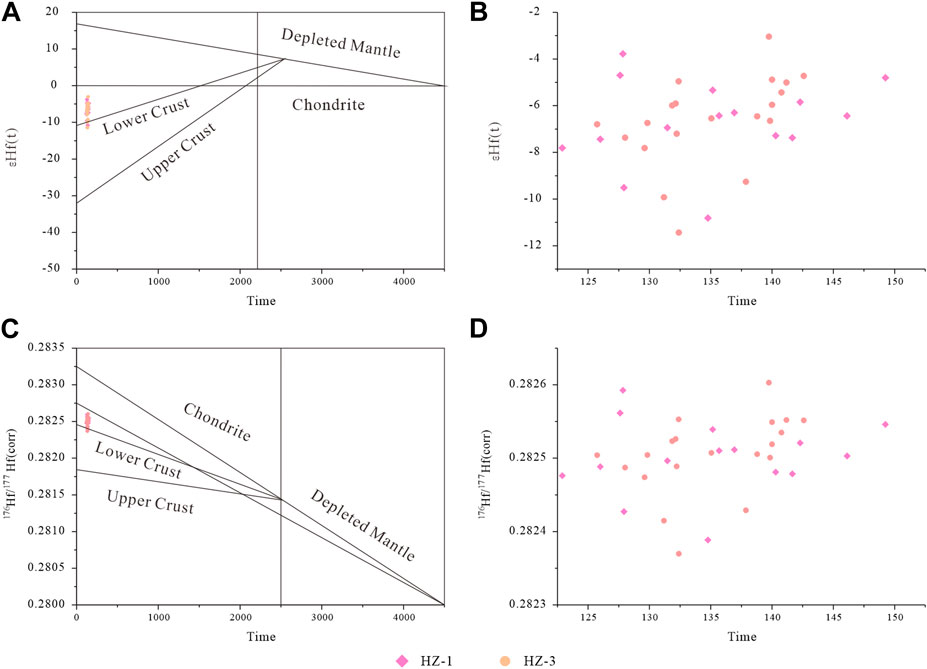
FIGURE 14. Zircon Lu-Hf isotopic compositions for the Cretaceous granite in (geothermal reservoir rock) the Huangshadong geothermal field.
6 Discussion
6.1 Timing of granite emplacement
The zircon ages of the granites in the HGF are summarized in Table 4; Figure 16. These ages indicate three stages of granite emplacement: 1) the Indosinian stage, corresponding to Permian granites (251 ± 9.1 to 253 ± 5 Ma); 2) the Yanshanian stage, including Jurassic granites (152.7 ± 2.7–176.7 ± 1.8 Ma); 3) the Cretaceous stage (135 ± 4–143.6 ± 2.8 Ma) (Xiao et al., 2019). These ages indicate intense Mesozoic (Yanshanian) magmatic activity in the study area. The Cretaceous granites dominate the HGF, exhibiting the largest outcrop area (100 km2; Figure 2), while the Permian granites only occur in the northern part of the geothermal field (Xiao et al., 2019). As indicated by the zircon dating results of samples HZ-1 and HZ-3 taken from the deep part of well HR-1, the surrounding rocks of the geothermal reservoirs in the HGF consist of Cretaceous granites, although Permian granites are the closest to geothermal wells (HR-1 and ZK8).
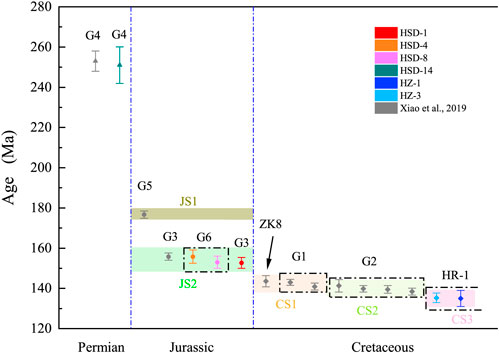
FIGURE 16. Zircon ages of granites in the Huangshadong geothermal field (Xiao et al., 2019). JS1: Jurassic stage 1; JS1: Jurassic stage 2; CS1: Cretaceous stage 1; CS2: Cretaceous stage 2; CS3: Cretaceous stage 3.
6.2 Petrogenesis of granites
As shown in the Harker diagram, the samples collected in this study were the product of felsic magmas and had undergone significant fractionation. The Yanshanian granites, represented by the samples collected in this study, show a downward trend of Al, Mg, and Ca contents with an increase in the SiO2 content (degree of fractionation; Figure 9). The depletion in Sr, Ba, and Ti, as well as prominent negative Eu anomalies (Figure 10), is indicative of the fractionation of plagioclase and Ti-Fe oxides. Figure 11 illustrates decreases in the Zr/Hf, Nb/Ta, and Th/U ratios but increases in the Rb/Sr ratio with an increase in the degree of magmatic fractionation (Stepanov et al., 2016; Breiter and Škoda, 2017; Cai et al., 2020). Furthermore, samples collected from or around geothermal wells exhibited significantly V-shaped REE patterns than those collected at a greater distance from geothermal wells, indicating that they underwent a higher degree of crystal fractionation.
Generally, granites can be categorized into S-, I-, A-, and M-types based on their genesis (Collins et al., 1982; Chappell and White, 2001; Chappell et al., 2012; Zhou et al., 2021). S-type granites originate from sedimentary rocks (Chappell et al., 1999), while I-type granites originate from ancient igneous rocks (Chappell and White, 2001). A-type granites are generally formed by the partial melting of dry granulitic residues in the lower crust, featuring a high abundance of large highly charged cations (Nb, Ga, and REEs) and low Mg, Ca, and Al content (Collins et al., 1982). M-type granites are derived from the subducted oceanic crust or overlying mantle (Whalen et al., 1987). A/CNK serves as an effective factor used to distinguish between S- and I-type granites (Chappell and White, 2001). Sample HSD-14 (Permian granites) had an A/CNK value of 1.2, suggesting that it was S-type granite. This result is consistent with the presence of primary muscovite (Figure 4). In contrast, Yanshanian granites (Jurassic and Cretaceous stages) exhibit A/CNK values of 1.05–1.13, ruling out the possibility of them being S-type granites. As presented in Figure 17, these Yanshanian granites fall within the zone of fractionated granites, suggesting that these granites are highly fractionated I-type granites rather than A-type granites. This finding is consistent with the downward trend of P content with an increase in the SiO2 content (Figure 10) and can also be evidenced by low Ti-in-zircon temperatures of 475–781°C (Table 3; Figure 7).
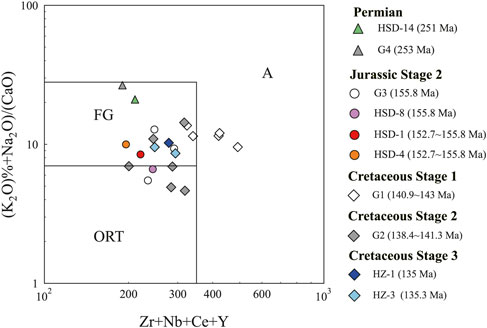
FIGURE 17. Discriminant diagrams of the granites in the Huangshadong geothermal field (Whalen et al., 1987). FG denotes fractionated felsic granites; OGT denotes unfractionated M-, I- and S-type granites.
Zircon Lu-Hf isotope compositions are an important tool for identifying the magma source of granites (Bhattacharya and Janwari, 2015; Bea et al., 2018). As indicated by the Hf isotope composition and TDM2 ages (1,385–1907 Ma) of samples HZ-1 and HZ-3, collected at depths of 2,637 m and 2,702 m, respectively (Table 6; Figures 14, 15), the reservoir granites originated from the mixing of Meso-to Paleo-Proterozoic lower crust and juvenile mantle materials. Two theories have been proposed for the formation of highly fractionated I-type granites, namely, the partial melting of crustal materials (Chappell et al., 1999; Wyborn et al., 2001) and the fractional crystallization of mafic melts (Chappell et al., 1999; Chappell et al., 2012). The latter assumption can be excluded for the following reasons: 1) such a large volume of granitoids (80%) is difficult to form due to the too small quantity of mafic rocks within igneous rocks (Zhang et al., 2015); 2) the parent magmas (peraluminous melts) of I-type granites are unlikely to be produced through the fractional removal of hornblende (Chappell et al., 2012). Instead, Cretaceous I-type granites in the study area are likely derived from the partial melting of the lower crust. Since the Early Jurassic (∼190 Ma), the Cathaysia Block had experienced asthenospheric upwelling and intra-continental lithosphere extension after the Indosinian flat-slab subduction of the Paleo-Pacific plate (Li and Li, 2007; Chen et al., 2008). As presented in Figure 18, these granites fall within the plate granite (WPG) zone, indicating an extensional setting. This finding is consistent with the Jurassic-Cretaceous coastward migration of extensional magmatism in the South China Block (Li and Li, 2007; Qiu et al., 2017), during which mafic magmas produced by the partial melting of the asthenospheric mantle triggered the partial melting of lower crust materials and the following generation of felsic magmas. This conclusion accords with the presence of gabbro-basalt rocks (height: 5 km) in the middle crust (depth: 20 km) (Zhang et al., 2005; Zhang et al., 2013).
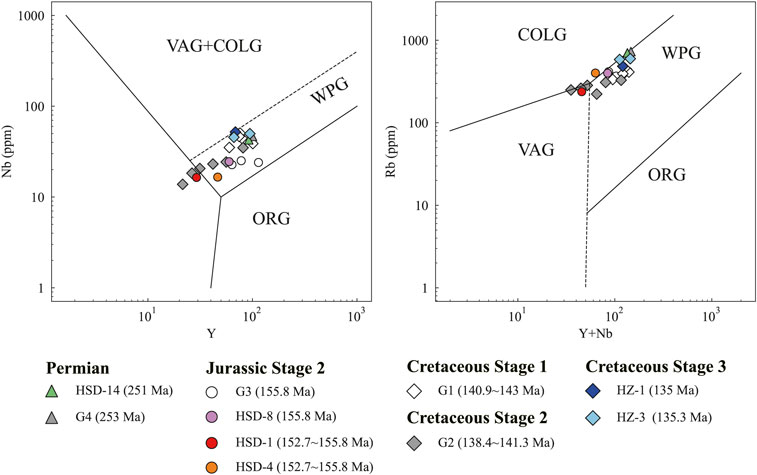
FIGURE 18. Tectonic setting discrimination diagrams of the granites in the Huangshadong geothermal field (after Pearce et al. (1984)). VAG. Volcanic-arc granite; syn-COLG. syn-collisional granite, WPG: within-plate granite; ORG: ocean-ridge granite.
6.3 Contributions of cretaceous granites to geothermal resources
Granites that have heat production rates >5 μW/m3 are classified as high-heat-producing granites, which have significant effects on the surface heat flow. The presence of high-heat-producing granites at depths of 1,650–2,980 m can increase the surface heat flow from the background value of 68 mW/m2 to 93 mW/m2, with an increase by 40% (Wang et al., 2023). Compared to magmas, radioactive heat causes a much lower increase in temperature but an extremely longer timespan of thermal anomalies. Mclaren et al. (1999) attributed the temperature increase of 40°C in the contact zone between plutons and host rocks to the decay of radiogenic elements. In the HGF, the Yanshanian granites exhibit heat production rates of 4.51–7.99 μW/m3, averaging 6.35 μW/m3 (Table 5). Therefore, they are high-heat-producing granites. Furthermore, the Cretaceous and Jurassic granites have average heat production rates of 6.63 μW/m3 and 6.06 μW/m3, respectively (Table 5).
According to lithological records and zircon ages (Tables 2, 6; Figures 6, 16), the concealed Cretaceous granites in the HGF occur at depths of 1,560–3,000 m in geothermal well HR-1 (Li et al., 2020b). The large volume of high-heat-producing Cretaceous granites (geothermal reservoir rocks) is an important heat source of geothermal resources in the study area. The surface heat flow of the HGF is 115.5 mW/m2, about 1.6 times the background heat flow (73 mW/m2) (Xi et al., 2021). In sum, the heat production of Cretaceous granites plays a major role in the formation of geothermal resources in the HGF.
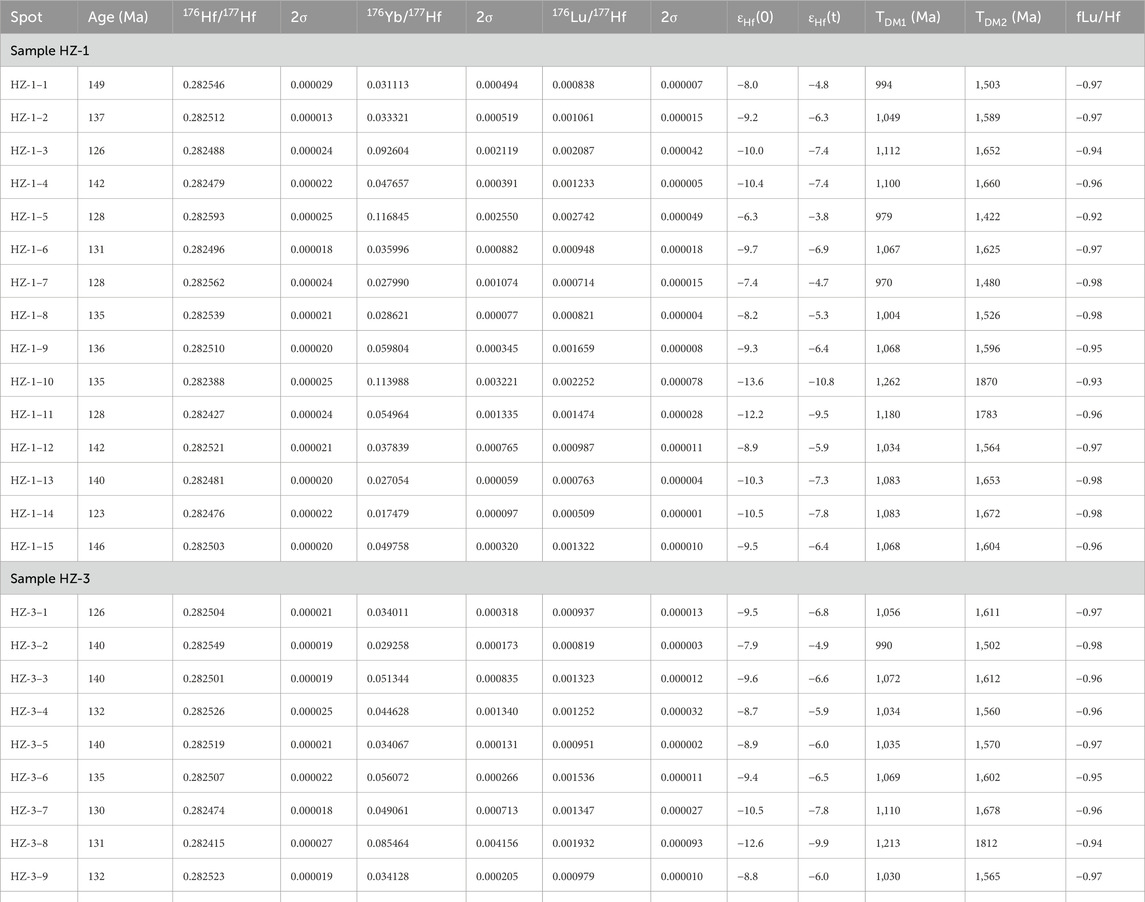
TABLE 6. Hf isotopic data of zircon grains for the granites (HZ-1 and HZ-3) in the Huangshadong geothermal field.
6.4 Magmatism evolution model
Previous studies summarized the regional petrogeological characteristics of granites based on granite samples collected from outcrops at a greater distance from HGF (G1, G2, G3, and G5 in Figure 2; Xiao et al., 2019). By comparison, all the samples used in this study were collected from or around geothermal wells (e.g., HR-1) to figure out the petrogenesis of geothermal reservoir rocks buried at depth and the magmatism evolution model of the HGF. As shown by the sampling locations and zircon ages of the samples (Figures 2, 5; Table 4), the ages of the Yanshanian granites exhibit a downward trend from the periphery to the center of the HGF (geothermal wells HR-1 and ZK8). In other words, the geothermal resources are hosted by relatively younger Yanshanian granites. Most especially, for Cretaceous granites, Xiao et al. (2019) obtained the emplacement ages ranging from 138.4 to 143.6 Ma based on the zircon dating of granite samples from G1, G2, and 300 m at the depth of ZK8 (Figure 2; Table 4). This study discovered that the reservoir granites at depth (∼3,000 m) intruded later than the upper and outer Cretaceous granites, with an emplacement age of ∼135 Ma. The reservoir granites exhibit the flattest REE pattern, indicating the strongest fractional crystallization among Cretaceous granites. This result accords with their higher heat generation. Their average heat production (HZ-1 and HZ-3, Table 4) is 6.44 μW/m3, while that of the upper (Id-33, 3.16 μW/m3) and outer (4.51 μW/m3) granites is much lower (representative samples: G1, G2; Figures 10–13). Regarding the Jurassic granites, two zircon ages were obtained by Xiao et al. (2019): 155.8 Ma for G3 and 176.7 Ma for G5. These ages indicate two episodes of Jurassic magmatism in the HGF. The intrusion ages of the Jurassic granites (HSD-1, HSD-4) exposed around the geothermal wells are consistent with those of granites (HSD-8) collected from G3 within the error range. This suggests that the Jurassic granites around the geothermal wells should be formed during the later Jurassic magmatic event (JS 2 in Figure 16) recorded by Xiao et al. (2019). Their average heat production rate (5.99 μW/m3, average value from HSD-1, HSD-4, HSD-8, and Id-04) is twice that (2.62 μW/m3) of granodiorites formed during the former Jurassic magmatic event. It is noteworthy that fine-grained equigranular biotite granites (HSD-4) intruded into HSD-1 (Figure 3) exhibit the strongest depletion of Ba, Nb, Sr, and Ti (Figure 12) and significant Eu anomalies (Figure 13) in the Jurassic granites, suggesting that they have undergone the strongest fractional differentiation (Chen et al., 2014; Liao et al., 2021). This implies that these granites are the latest phase of Jurassic granites.
In sum, the Yanshanian granites around geothermal wells were formed at the late stage of both the Jurassic and Cretaceous. The geochronological data of the granites in the HGF were integrated with the evolution model for plutonism in this study (Figure 19), yielding findings as follows. The Permian two-mica granites (G4 in Figure 2; Figure 19) intruded into the Paleozoic sandstone at the center of the HGF at 253–251 Ma. During the Jurassic, two magmatic events occurred. At 176.7 Ma, granodiorites were emplaced in the southeast of this study area (Jurassic stage 1), while the intrusion of biotite granites (G3 and G6 in Figure 2; Figure 19) occurred at 155.8–153 Ma (Jurassic stage 2). During the Cretaceous, three stages of magmatism occurred: 1) stage 1: biotite granites (G1) were exposed in the northwest of the HGF, with ages ranging from 143.6 to 140.9 Ma; 2) stage 2: biotite granites (G1) were in intrusive contact with granodiorites (G5) in the southeast of the HGF; 3) stage 3: biotite granite intruded under the two-mica granites and Paleozoic sandstones at 135–135.3 Ma as the reservoir rocks of geothermal resources in the HGF.
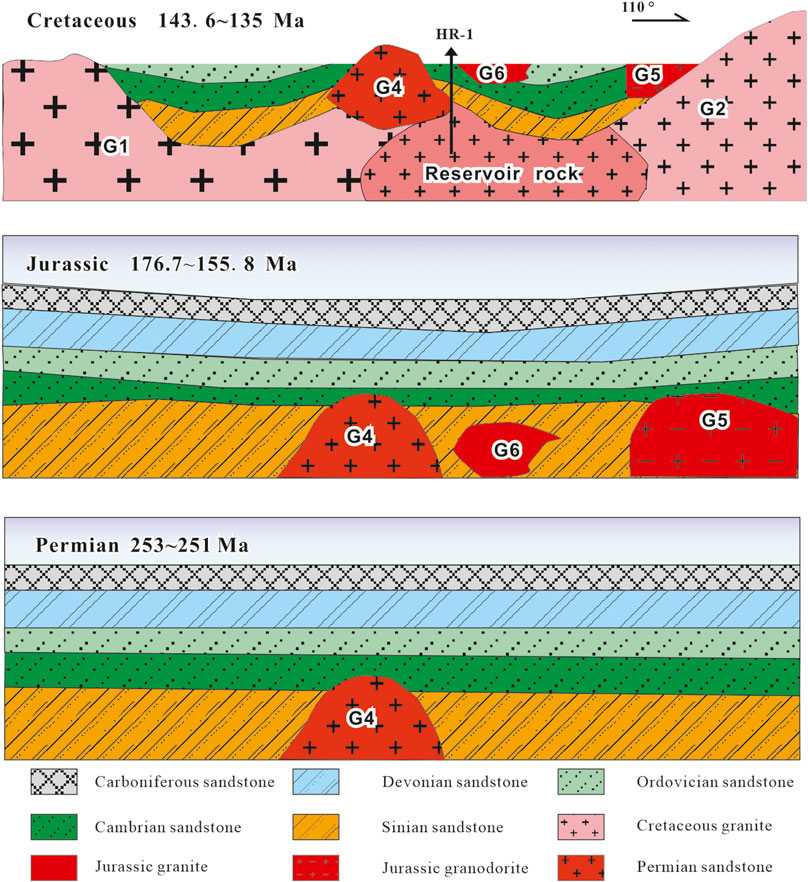
FIGURE 19. Magmatism evolution of Huangshadong geothermal field (the location of profile is shown in Figure 2).
7 Conclusion
(1) In the HGF, igneous rocks are dominated by Yanshanian granites (highly fractionated I-type granites), with Permian granites exposed in only limited areas.
(2) As indicated by the Hf isotopic composition of zircons, the reservoir granites (1,500–300 m) originated from the Meso-to Paleo-Proterozoic lower crustal materials.
(3) The heat generated from Cretaceous granites with high heat production serves as a primary heat source for the formation of geothermal energy in the HGF.
Data availability statement
The original contributions presented in the study are included in the article/Supplementary material, further inquiries can be directed to the corresponding author.
Author contributions
YL: Conceptualization, Data curation, Formal Analysis, Investigation, Methodology, Software, Visualization, Writing–original draft, Writing–review and editing. GW: Conceptualization, Funding acquisition, Project administration, Resources, Validation, Writing–review and editing. YX: Funding acquisition, Project administration, Writing–review and editing. HG: Resources, Writing–review and editing. XY: Resources, Writing–review and editing. MY: Data curation, Writing–review and editing. WZ: Resources, Writing–review and editing. ZZ: Investigation, Writing–review and editing.
Funding
The author(s) declare financial support was received for the research, authorship, and/or publication of this article. This work was funded by a project of National Natural Science Foundation of China (41874100), National Key Research and Development Program of China (2021YFB1507401), China Geological Survey (DD20221676).
Conflict of interest
The authors declare that the research was conducted in the absence of any commercial or financial relationships that could be construed as a potential conflict of interest.
Publisher’s note
All claims expressed in this article are solely those of the authors and do not necessarily represent those of their affiliated organizations, or those of the publisher, the editors and the reviewers. Any product that may be evaluated in this article, or claim that may be made by its manufacturer, is not guaranteed or endorsed by the publisher.
References
Alqahtani, F., Aboud, E., Ehsan, M., Naseer, Z., Abdulfarraj, M., Abdelwahed, M. F., et al. (2023a). Geothermal exploration using remote sensing, surface temperature, and geophysical data in lunayyir volcanic field, Saudi Arabia. Sustainability 15, 7645. doi:10.3390/su15097645
Alqahtani, F., Ehsan, M., Aboud, E., Abdulfarraj, M., and El-Masry, N. (2023b). Integrated approach using petrophysical, gravity, and magnetic data to evaluate the geothermal resources at the rahat volcanic field, Saudi Arabia. Front. Earth Sci. 11, 1135635. doi:10.3389/feart.2023.1135635
Artemieva, I. M., and Thybo, H. (2013). Eunaseis: a seismic model for moho and crustal structure in europe, Greenland, and the north atlantic region. Tectonophysics 609, 97–153. doi:10.1016/j.tecto.2013.08.004
Artemieva, I. M., Thybo, H., Jakobsen, K., Sorensen, N. K., and Nielsen, L. S. K. (2017). Heat production in granitic rocks: global analysis based on a new data compilation granite2017. Earth-Science Rev. 172, 1–26. doi:10.1016/j.earscirev.2017.07.003
Bea, F., Montero, P., Molina, J. F., Scarrow, J. H., Cambeses, A., and Moreno, J. A. (2018). Lu-hf ratios of crustal rocks and their bearing on zircon hf isotope model ages: the effects of accessories. Chem. Geol. 484, 179–190. doi:10.1016/j.chemgeo.2017.11.034
Bhattacharya, S., and Janwari, S. (2015). A short review on lu-hf isotope system in zircon: implications for crustal evolution. J. Indian Inst. Sci. 95 (2), 147–157. doi:10.1007/s00531-007-0239-2
Black, L. P., Kamo, S. L., Allen, C. M., Aleinikoff, J. N., Davis, D. W., Korsch, R. J., et al. (2003). Temora 1: a new zircon standard for phanerozoic u–pb geochronology. Chem. Geol. 200 (1), 155–170. doi:10.1016/s0009-2541(03)00165-7
Breiter, K., and Škoda, R. (2017). Zircon and whole-rock zr/hf ratios as markers of the evolution of granitic magmas: examples from the teplice caldera (Czech republic/germany). Mineralogy Petrology 111 (115), 435–457. doi:10.1007/s00710-017-0509-z
Cai, Y., Liu, H., Feng, Z., Zhou, Y., Liu, X., Wang, Z., et al. (2020). Neoproterozoic active margin of the sw south China block: constraints from u pb ages, sr nd isotopes and geochemical data for the gabbro and granodiorite along the ailaoshan tectonic belt. Lithos 358-359, 105387. doi:10.1016/j.lithos.2020.105387
Chappell, B. W., Bryant, C. J., and Wyborn, D. (2012). Peraluminous i-type granites. Lithos 153, 142–153. doi:10.1016/j.lithos.2012.07.008
Chappell, B. W., Sial, A. N., Stephens, W. E., and Ferreira, V. P. (1999). Aluminium saturation in i- and s-type granites and the characterization of fractionated haplogranites. Lithos 46 (3), 535–551. doi:10.1016/s0024-4937(98)00086-3
Chappell, B. W., and White, A. J. R. (2001). Two contrasting granite types: 25 years later. Aust. J. Earth Sci. 48 (4), 489–499. doi:10.1046/j.1440-0952.2001.00882.x
Chen, C. H., Lee, C. Y., and Shinjo, R. I. (2008). Was there jurassic paleo-pacific subduction in south China? constraints from 40ar/39ar dating, elemental and sr-nd-pb isotopic geochemistry of the mesozoic basalts. Lithos 106 (1-2), 83–92. doi:10.1016/j.lithos.2008.06.009
Chen, H., Song, R., Zhaong, C., Liang, Y., Wang, Y., Zheng, F., et al. (2023). Geothermal storage evaluation of geothermal resources in hot dry rock based on factor analysis method. J. Chengdu Univ. Technol. Sci. Technol. Ed. 50 (03), 333–350. doi:10.3969/j.issn.1671-9727.2023.03.08
Chen, X., Liu, J., Zhang, Q., Yang, Z., Yang, L., and Wu, J. (2014). Characteristics of hf isotopes and zircon u-pb ages of granites in the cuihongshan iron polymetallic deposit, heilongjiang and their geologic implications. Bull. Mineralogy Petrology Geochem. 33 (5), 636–644. doi:10.1002/gj.3224
Collins, W. J., Beams, S. D., White, A. J. R., and Chappell, B. W. (1982). Nature and origin of a-type granites with particular reference to southeastern Australia. Contributions Mineralogy Petrology 80 (2), 189–200. doi:10.1007/bf00374895
Ferry, J. M., and Watson, E. B. (2007). New thermodynamic models and revised calibrations for the ti-in-zircon and zr-in-rutile thermometers. Contributions Mineralogy Petrology 154 (4), 429–437. doi:10.1007/s00410-007-0201-0
Geng, J., Li, H., Zhang, J., Zhou, H., and Li, H. (2011). Zircon hf isotope analysis by means of la-mc-icp-ms. Geol. Bull. China 30 (10), 1508–1513.
Hasterok, D., and Chapman, D. S. (2011). Heat production and geotherms for the continental lithosphere. Earth Planet. Sci. Lett. 307 (1-2), 59–70. doi:10.1016/j.epsl.2011.04.034
Hu, R. Z., Chen, W. T., Xu, D. R., and Zhou, M. F. (2017). Reviews and new metallogenic models of mineral deposits in south China: an introduction. J. Asian Earth Sci. 137, 1–8. doi:10.1016/j.jseaes.2017.02.035
Kemp, A. I. S., and Hawkesworth, C. J. (2003). 3.11 – granitic perspectives on the generation and secular evolution of the continental crust. Treatise Geochem. 3 (6), 349–410. doi:10.1016/j.epsl.2009.05.011
Kromkhun, K. (2010). Petrogenesis of high heat producing granite: implication for mt painter province, south Australia. Thesis. Adelaide: University of Adelaide, School of Earth and Environmental Sciences.
Li, T., Lin, W., Gan, H., Yue, G., Zhang, D., and Wang, G. (2020a). Ocurence prospect of hdr and target site selection study in southeastern of China. Acta Geol. Sinca 026 (002), 187–200.
Li, T., Lin, W., Gan, H., Yue, G., Zhang, D., and Wang, G. (2020b). Research on the genetic model and exploration progress of hot dry rock resources on the southeast coast of China. J. Geomechanics 026 (002), 187–200. doi:10.12090/j.issn.1006-6616.2020.26.02.018
Li, X. H., Li, W. X., Li, Z. X., Lo, C. H., Wang, J., Ye, M. F., et al. (2009). Amalgamation between the yangtze and cathaysia blocks in south China: constraints from shrimp u–pb zircon ages, geochemistry and nd–hf isotopes of the shuangxiwu volcanic rocks. Precambrian Res. 174 (1), 117–128. doi:10.1016/j.precamres.2009.07.004
Li, Z.-X., and Li, X.-H. (2007). Formation of the 1300-km-wide intracontinental orogen and postorogenic magmatic province in mesozoic south China: a flat-slab subduction model. Geology 35 (2), 179–182. doi:10.1130/g23193a.1
Liao, Y., Zhang, D., Danyushevsky, L. V., Li, T., and Liu, Y. (2021). Protracted lifespan of the late mesozoic multistage qianlishan granite complex, nanling range, se China: implications for its genetic relationship with mineralization in the dongpo ore field. Ore Geol. Rev. 139, 104445. doi:10.1016/j.oregeorev.2021.104445
Lin, W., Gan, H., Wang, G., and Ma, F. (2016). Occurrence prospect of hdr and target site selection study in southeastern of China. Acta Geol. Sin. 90 (08), 2043–2058.
Lin, W., Wang, G., and Gan, H. (2023). Differential crustal thermal structure and geothermal significance in the igneous region of southeastern China. Acta Geol. Sin. 2023, 1–14. doi:10.19762/j.cnki.dizhixuebao.2023027
Lin, W., Wang, G., Gan, H., Wang, A., Yue, G., Long, X., et al. (2022). Heat generation and accumulation for hot dry rock resources in the igneous rock distribution areas of southeastern China. Lithosphere 2021 (5), 2039112. doi:10.2113/2022/2039112
Liu, C., Zheng, J., Li, Z., Li, Y., Hao, Q., and Li, J. (2021). Analysis on the situation and countermeasures of water resources supply and demand in the cities of small and medium-sized river basins along southeast coast of China — taking xiamen city as an example. J. Groundw. Sci. Eng. 9 (4), 350. doi:10.19637/j.cnki.2305-7068.2021.04.008
Liu, H., Guo, H., Xing, L., Zhan, Y., Li, F., Shao, J., et al. (2016). Geochemical behaviors of rare earth elements in groundwater along a flow path in the north China plain. J. Asian Earth Sci. 117 (MAR.1), 33–51. doi:10.1016/j.jseaes.2015.11.021
Liu, Y. S., Gao, S., Hu, Z. C., Gao, C. G., Zong, K. Q., and Wang, D. B. (2010). Continental and oceanic crust recycling-induced melt-peridotite interactions in the trans-north China orogen: U-pb dating, hf isotopes and trace elements in zircons from mantle xenoliths. J. Petrology 51 (1-2), 537–571. doi:10.1093/petrology/egp082
Lu, S. M. (2018). A global review of enhanced geothermal system (egs). Renew. Sustain. Energy Rev. 81, 2902–2921. doi:10.1016/j.rser.2017.06.097
Lund, J. W., and Toth, A. N. (2021). Direct utilization of geothermal energy 2020 worldwide review. Geothermics 90, 101915. doi:10.1016/j.geothermics.2020.101915
Lv, Q. (2010). Methods for chemical analysis of silicate rocks - part 28: Determination of 16 major and minor elements content (gb/t 14506.28-2010). Beijing; General Administration of Quality Supervision, Inspection and Quarantine of the People's Republic of China.
Ma, F., Wang, G., Sun, H., and Sun, Z. (2022). Indication of hydrogen and oxygen stable isotopes on the characteristics and circulation patterns of medium-low temperature geothermal resources in the guanzhong basin, China. J. Groundw. Sci. Eng. 10 (1), 70. doi:10.19637/j.cnki.2305-7068.2022.01.007
Mao, J. W., Chen, M. H., Yuan, S. D., and Guo, C. L. (2011). Geological characteristics of the qinhang (or shihang) metallogenic belt in south China and spatial-temporal distribution regularity of mineral deposits. Acta Geol. Sin. 85 (5), 636–658.
Marshall, V. J. (2014). Petrological, geochemical and geochronological characterisation of heat-producing granites. Heat Producing Granite.
Mclaren, S., Neumann, N., Sandiford, M., and Wyborn, L. (1999). Post-intrusion heating associated with high-heat-producing proterozoic granites implications for mineralisation?: AGSO Research Newsletter, 30–31.
Pang, Z., Huang, T., Tian, J., Cheng, Y., Huang, T., et al. (2023). Formation of the hydrothermal system from granite reservoir for power generation in igneous rock areas of south China. Geothermics 110, 102673. doi:10.1016/j.geothermics.2023.102673
Paton, C., Woodhead, J. D., Hellstrom, J. C., Hergt, J. M., Greig, A., and Maas, R. (2010). Improved laser ablation u-pb zircon geochronology through robust downhole fractionation correction. Geochem. Geophys. Geosystems 11 (3). doi:10.1029/2009gc002618
Pearce, J. A., Harris, N. B. W., and Tindle, A. G. (1984). Trace-element discrimination diagrams for the tectonic interpretation of granitic-rocks. J. Petrology 25 (4), 956–983. doi:10.1093/petrology/25.4.956
Qiu, X. L., Wang, Y., Wang, Z. Z., Regenauer-Lieb, K., Zhang, K., and Liu, J. (2018). Determining the origin, circulation path and residence time of geothermal groundwater using multiple isotopic techniques in the heyuan fault zone of southern China. J. Hydrology 567, 339–350. doi:10.1016/j.jhydrol.2018.10.010
Qiu, Z., Yan, Q., Li, S., Wang, H., Tong, L., Zhang, R., et al. (2017). Highly fractionated early cretaceous i-type granites and related sn polymetallic mineralization in the jinkeng deposit, eastern guangdong, se China: constraints from geochronology, geochemistry, and hf isotopes. Ore Geol. Rev. 88, 718–738. doi:10.1016/j.oregeorev.2016.10.008
Roth, J., and Littke, R. (2022). Down under and under cover-the tectonic and thermal history of the cooper and central eromanga basins (central eastern Australia). Geosciences 12 (3), 117. doi:10.3390/geosciences12030117
Sláma, J., Košler, J., Condon, D. J., Crowley, J. L., Gerdes, A., Hanchar, J. M., et al. (2008). Plešovice zircon—a new natural reference material for u–pb and hf isotopic microanalysis. Chem. Geol. 249 (1-2), 1–35. doi:10.1016/j.chemgeo.2007.11.005
Stepanov, A. S., Meffre, S., Mavrogenes, J., and Steadman, J. (2016). Nb-ta fractionation in peraluminous granites: a marker of the magmatichydrothermal transition. Geology 44, 231–234. doi:10.1130/G37475.1
Sun, S.-S., and Mcdonough, W.-S. (1989). Chemical and isotopic systematics of oceanic basalts: implications for mantle composition and processes. Geol. Soc. Lond. Spec. Publ. 42 (1), 313–345. doi:10.1144/gsl.sp.1989.042.01.19
Tester, J. W., Anderson, B. J., Batchelor, A. S., Blackwell, D. D., Dipippo, R., and Drake, E. M. (2006). The future of geothermal energy. Idaho Falls: Massachusetts Institute of Technology.
Ullah, J., Li, H., Ashraf, U., Heping, P., Ali, M., Ehsan, M., et al. (2023). Knowledge-based machine learning for mineral classification in a complex tectonic regime of yingxiu-beichuan fault zone, sichuan basin. Geoenergy Sci. Eng. 229, 212077. doi:10.1016/j.geoen.2023.212077
Wang, Y., Furlong, K., Fuchs, S., He, L., and Hu, S. (2023). Terrestrial heat flow variation with depth caused by anomalously high radiogenic heat production. Geophys. Res. Lett. 50 (8), e2022GL102312. doi:10.1029/2022GL102312
Whalen, J. B., Currie, K. L., and Chappell, B. W. (1987). A-type granites: geochemical characteristics, discrimination and petrogenesis. Contributions Mineralogy Petrology 95 (4), 407–419. doi:10.1007/bf00402202
Wiedenbeck, M., Allé, P., Corfu, F., Griffin, W. L., Meier, M., Oberli, F., et al. (1995). Three natural zircon standards for u-th-pb, lu-hf, trace element and ree analyses.
Wyborn, D., Chappell, B. W., and James, M. (2001). Examples of convective fractionation in high-temperature granites from the lachlan fold belt. Aust. J. Earth Sci. 48 (4), 531–541. doi:10.1046/j.1440-0952.2001.00877.x
Xi, Y., Zhao, Y., and Da, Y. (2021). Geothermal structure revealed by curie isothermal surface under guangdong province, China. J. Groundw. Sci. Eng. 9 (2), 114. doi:10.19637/j.cnki.2305-7068.2021.02.003
Xiao, Z., Wang, S., Qi, S., Kuang, J., Han, Y., Tian, F., et al. (2019). Petrogenesis, tectonic evolution and geothermal implications of mesozoic granites in the huangshadong geothermal field, south China. J. Earth Sci. 31 (3), 141–158. doi:10.1007/s12583-019-1242-9
Zhang, C., Feng, Q., Zhang, L., Qin, S., Jiang, G., Hu, J., et al. (2022). Characteristics of radiogenic heat production of widely distributed granitoids in western sichuan, southeast Tibetan plateau. Lithosphere 2022, 4165618. doi:10.2113/2022/4165618
Zhang, Y., Yang, J. H., Sun, J. F., Zhang, J. H., Chen, J. Y., and Li, X. H. (2015). Petrogenesis of jurassic fractionated i-type granites in southeast China: constraints from whole-rock geochemical and zircon u-pb and hf-o isotopes. J. Asian Earth Sci. 111, 268–283. doi:10.1016/j.jseaes.2015.07.009
Zhang, Z. J., Badal, J., Li, Y. K., Chen, Y., Yang, L. Q., and Teng, J. W. (2005). Crust-upper mantle seismic velocity structure across southeastern China. Tectonophysics 395 (1-2), 137–157. doi:10.1016/j.tecto.2004.08.008
Zhang, Z. J., Xu, T., Zhao, B., and Badal, J. (2013). Systematic variations in seismic velocity and reflection in the crust of cathaysia: new constraints on intraplate orogeny in the south China continent. Gondwana Res. 24 (3-4), 902–917. doi:10.1016/j.gr.2012.05.018
Zhao, P., and Luo, D. (1995). Characteristics of heat production distribution in se China. Acta Petrol. Sin. 11 (3), 292–305.
Zhou, X. M., Sun, T., Shen, W. Z., Shu, L. S., and Niu, Y. L. (2006). Petrogenesis of mesozoic granitoids and volcanic rocks in south China: a response to tectonic evolution. Episodes 29 (1), 26–33. doi:10.18814/epiiugs/2006/v29i1/004
Zhou, Y., Sun, S., Feng, Z., Xu, C., Cai, Y., Liang, X., et al. (2021). A new insight into the eastern extension of the proto-tethyan margin of gondwana by early paleozoic volcanic rocks in south China. Lithos 398-399, 106328. doi:10.1016/j.lithos.2021.106328
Keywords: high-heat-producing granite, Huangshadong geothermal field, cretaceous granite, highly fractionated granite, heat source
Citation: Liao Y, Wang G, Xi Y, Gan H, Yan X, Yu M, Zhang W and Zhao Z (2024) Petrogenesis of high heat producing granites and their contribution to geothermal resource in the Huangshadong geothermal field, South China. Front. Earth Sci. 12:1342969. doi: 10.3389/feart.2024.1342969
Received: 22 November 2023; Accepted: 08 January 2024;
Published: 08 February 2024.
Edited by:
Paolo Capuano, University of Salerno, ItalyReviewed by:
Muhsan Ehsan, Bahria University, PakistanHua-Wen Cao, Chengdu University of Technology, China
Copyright © 2024 Liao, Wang, Xi, Gan, Yan, Yu, Zhang and Zhao. This is an open-access article distributed under the terms of the Creative Commons Attribution License (CC BY). The use, distribution or reproduction in other forums is permitted, provided the original author(s) and the copyright owner(s) are credited and that the original publication in this journal is cited, in accordance with accepted academic practice. No use, distribution or reproduction is permitted which does not comply with these terms.
*Correspondence: Guiling Wang, aWhlZ3dhbmdndWlsaW5nQHNpbmEuY29t