- College of Petroleum Engineering, Xi’an Shiyou University, Xi’an, China
The study aims to investigate the rock mechanical properties of the Chang 7 member tight oil reservoir in the Longdong region of the Ordos Basin, China, with the goal of enhancing the efficiency of oilfield development. Despite numerous contributions in the field of rock mechanics, challenges persist in reconciling experimental results with actual geological conditions and achieving comprehensive understanding of rock mechanical properties in tight oil reservoirs. To address this, a variety of experimental methods were employed to systematically assess the mechanical properties of the target reservoir. Rock density was measured using volumetric methods, tensile strength was evaluated through the Brazilian test, uniaxial and triaxial compression tests were conducted to assess rock mechanics properties, and dynamic elastic modulus and Poisson’s ratio were obtained via sonic velocity measurements. Furthermore, differential strain analysis and imaging log analysis were employed to determine the magnitude and direction of geostress. The results revealed that fine-grained sandstone exhibited higher rock density and relatively higher tensile strength, while muddy siltstone exhibited comparatively lower values in these aspects. Analysis of the influence of confining pressure on rock mechanics properties demonstrated a strong positive correlation between compressive strength and Young’s modulus with confining pressure, while Poisson’s ratio exhibited more irregular variations. Additionally, a mathematical relationship between dynamic and static rock mechanical parameters was established. Lastly, based on the characteristics of geostress, reliable foundations for optimizing hydraulic fracturing and wellbore layout were provided. This study has enriched and complemented the investigation of rock mechanical properties in tight reservoirs, offering vital parameters and theoretical support for the development of tight oil reservoirs. This bears significant importance in enhancing petroleum extraction efficiency and optimizing engineering design.
1 Introduction
In the field of reservoir rock physical mechanics, previous research has covered various aspects. Key studies in this field include Gao et al. (2016), who explored rock deformation in the Lucaogou Formation in the Jimsar Sag, China, to reveal rock deformation characteristics and strength, providing a basis for establishing reservoir rock mechanics parameter logging evaluation models. Additionally, Li et al. (2022) performed triaxial stress tests on the continental mixed fine-grained sedimentary rocks in the western part of the Qaidam Basin, China, to investigate the correlation between rock mechanics properties and brittleness. Xiong et al. (2023) analyzed the rock mechanics properties of the fluvial shale reservoir of the Fengcheng Formation in the Mahu Sag, Junggar Basin, China, using reservoir logging and post-fracturing oil test data, and studied the influence of rock mechanics properties on the fracturing effect of the fluvial shale reservoir. However, despite the valuable contributions of these studies in the field of rock mechanics, there are still some limitations. Firstly, although experimental research provides valuable information about the mechanical properties and strength of rocks, there are differences between experimental conditions and actual geological conditions. Therefore, the applicability of research results in practical applications needs further validation. Secondly, the current research understanding of the rock mechanics properties of specific regions or oil and gas fields is still not comprehensive enough to serve as a basis for engineering applications. The rock physical mechanical properties play a crucial role in the development of tight oil reservoirs, which refer to oil-bearing formations with low porosity and poor permeability. Accurate assessment and understanding of rock physical mechanical parameters are essential for the efficient development of such reservoirs (Liu et al., 2009; Zhang P. et al., 2022). A comprehensive understanding of the physical-mechanical properties of rocks in tight oil reservoirs, such as elastic modulus, Poisson’s ratio, compressive strength, etc., can help predict reservoir deformation behavior, fracture propagation characteristics, and oil and gas migration paths, thereby guiding the optimization of development strategies and increasing production capacity.
2 Geological backgrouond
The Chang 7 Member reservoir in the Longdong area is one of the significant targets in the Ordos Basin, China, with important potential for tight oil resources. As a large polyphase cratonic basin, the Ordos Basin has undergone overall subsidence and basin migration processes (Zhong et al., 2013; He and Yun, 2021). During its sedimentary evolution, the Chang 7 period represents the largest expansion phase of the lake basin, characterized by deep and extensive water bodies, and the deposition of a nearly 100-m-thick series of oil-bearing rocks dominated by oil shale, providing the foundation for Mesozoic continental oil generation (Yang et al., 2022). The study area in the Longdong area extends from Wuqi in the north to Zhengning in the south, Zhenyuan in the west, and Ta’erwan in the east, covering an area of approximately 3×104 km2 (Figure 1), with proven oil reserves reaching 5×108 km2. However, due to the complex geological and rock physical mechanical properties, the development of the tight oil reservoir in the Chang 7 Member faces challenges. The rock physical mechanical properties are crucial factors in assessing reservoir’s development feasibility, determining development strategies, and optimizing production efficiency. Nevertheless, the insufficient understanding of the rock physical mechanical properties of the tight oil reservoirs in the study area has constrained the targeted and effective implementation of engineering measures.
3 Methods
In this study, we conducted rock density measurements, triaxial rock mechanics experiments, acoustic experiments, and geostress tests, and collected FMI (Formation Micro-Imager) logging data.
The dry density of 33 rock samples was measured using the volumetric method. Cylindrical specimens with dimensions of Φ25×50 mm were prepared, ensuring a height-to-diameter ratio of 2.0–2.5. The dimensions of the specimens were measured, and their volumes were calculated. The specimens were then dried continuously for 1–2 days at a temperature of 105ºC–110°C and cooled to room temperature. The dry mass of the specimens was measured with an accuracy of 0.01 g. The dry density was calculated using the mass and volume of the specimens.
The research employed the Brazilian tensile test to measure the tensile strength of the rocks. Firstly, the dimensions of the specimens were measured, and then the specimens were dried continuously for 1–2 days at a temperature of 105°C–110 °C and cooled to room temperature in a drying oven. Next, the specimens were accurately placed in the testing apparatus, and a relatively linear load was applied using the Brazilian tensile testing machine to induce tensile stress along the radial direction of the specimen until failure occurred, and the failure load value was recorded. Finally, the tensile strength of the rock was calculated using the formula St = 2P/πDL, where St is the tensile strength of the rock (MPa), P is the failure load (KN), D is the diameter of the specimen (cm), and L is the thickness of the specimen (cm).
The uniaxial compression test method was used to evaluate the mechanical properties of rocks. The oil and gas reservoir geostress testing system (Figure 2) was utilized as the testing instrument, and the specimens were prepared according to the standard procedures. During the test procedure, axial deformation sensors were connected, and initial values were set. The test was conducted using axial load control and radial deformation control until the specimen failed. The oil and gas reservoir geostress testing system automatically collected data on stress, strain, and other parameters. Based on relevant calculation formulas, the axial failure stress, elastic modulus, and Poisson’s ratio were determined.
The rock triaxial compression test was used to study the deformation and strength characteristics of the rocks. In the high-temperature high-pressure dynamic rock triaxial testing system (Figure 2), the longitudinal and lateral deformations of the rock samples were measured under different confining pressures to obtain the elastic modulus, Poisson’s ratio, and triaxial compressive strength of the rocks. The specimens were cylindrical with dimensions of Φ25×50 mm, meeting the preparation requirements. The test procedure included connecting deformation sensors, applying confining pressure, and controlling axial load and radial deformation. Computer software was used to collect and determine the elastic modulus and Poisson’s ratio under different confining pressure conditions.
The ultrasonic pulse transmission method was used to conduct rock acoustic wave characterization using the ultrasonic rock parameter tester (Figure 3). By measuring the propagation time of longitudinal or transverse waves along the length direction of the specimen, the longitudinal and transverse wave velocities of the rock sample were calculated. Furthermore, using the obtained velocity values in conjunction with the sample’s density, we can determine the elastic modulus and Poisson’s ratio of the sample under a given pressure.
The differential strain analysis test method was used to determine the direction and magnitude of geostress (Zhang X. J. et al., 2022). This method is based on the assumption that the rock mechanical properties are isotropic and utilizes the ratio relationship of principal strains to determine the magnitude of geostress. Firstly, rock samples were extracted from underground to eliminate the effect of geostresses, causing the opening of microcracks within the rocks. The direction and density of these microcracks are proportional to the pre-existing geostress state. Then, the extracted rock samples were processed into cubic rock blocks, and three strain gauges were attached to three mutually perpendicular planes at 45° angles. Subsequently, the rock samples were placed inside a pressure chamber, and three equal confining pressures were applied in different directions. Simultaneously, the strains in each direction were measured. By analyzing the principal strain features and their corresponding stress values, determine the direction and magnitude of geostresses.
4 Results
4.1 Rock density test
Rock density measurements were conducted using the volumetric method, which involves measuring the mass of the rock sample per unit volume after it is dried. The test results (Table 1) indicate that the average rock density of fine-grained sandstone is 2.543 g/cm3, and the average rock density of muddy siltstone is 2.597 g/cm3. The fine-grained sandstone in the Longdong area exhibits relatively high rock density (typically around 2.3 g/cm3 for clastic rocks), indicating that the rocks are dense (Wang et al., 2009).
4.2 Rock tensile strength test
The maximum capacity to resist tensile failure is referred to as the rock tensile strength (Zhang et al., 2021). There are various methods for testing tensile strength, and in this experiment, the split tensile test method was used to determine the rock tensile strength. The test results (Table 2) indicate that the average tensile strength of fine-grained sandstone is 6.43 MPa, while the average tensile strength of muddy siltstone is 4.08 MPa.
4.3 Rock uniaxial compression test
Rock uniaxial compression testing involves measuring the longitudinal and lateral deformations of regular-shaped rock specimens under uniaxial pressure to determine the rock’s elastic modulus, Poisson’s ratio, and uniaxial compressive strength (Gao et al., 2022; Zhao et al., 2023). The test results (Table 3) indicate that the average compressive strength of fine-grained sandstone is 137.38 MPa, the average elastic modulus is 2.623×104 MPa, and the average Poisson’s ratio is 0.246. For muddy siltstone, the average compressive strength is 108.969 MPa, the average elastic modulus is 2.330×104 MPa, and the average Poisson’s ratio is 0.198.
4.4 Rock triaxial compression test
The purpose of rock triaxial compression test is to understand the deformation and strength characteristics of rocks under complex stress conditions. The experiment provides mechanical parameters and the Mohr circle envelope (Figure 4) for rocks under different pressure conditions. The Mohr circle envelope represents the enveloping circle of the maximum principal stress and can fully describe the failure characteristics of rocks under any stress state, enabling the determination of the internal friction angle and cohesion of the rocks (Chen et al., 2021; Wang et al., 2023). By measuring the longitudinal and lateral deformations of regularly-shaped rock specimens under different confining pressures, the elastic modulus, Poisson’s ratio, and triaxial compressive strength of the rocks were determined. In this experiment, the equal lateral pressure triaxial compression test (σ1>σ2=σ3) was conducted to simulate real geological conditions (Wang S. L. et al., 2022; Li, 2022). The loading pressure levels were determined based on the lithology and depth. The test results (Table 4–6) indicate that the average cohesion of fine-grained sandstone is 28.6 MPa, and the internal friction angle is 45.7°. For muddy siltstone, the average cohesion is 25.075 MPa, and the internal friction angle is 38.06°.
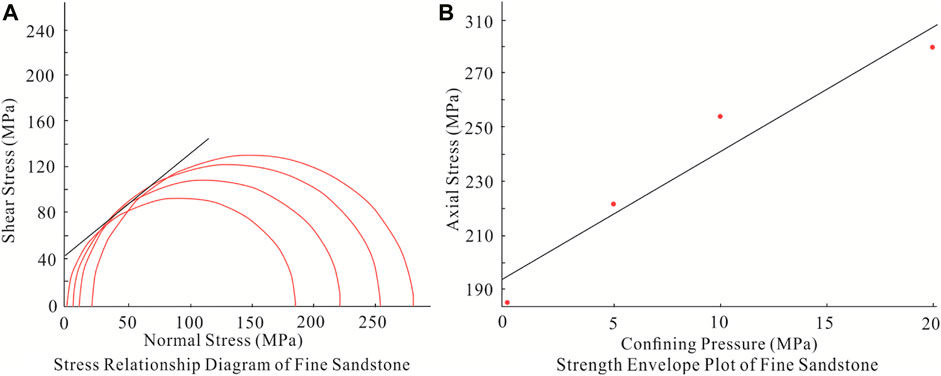
FIGURE 4. Relevant diagrams for triaxial compression (N25 well, 1,407.12m–1,412.05 m). (A) Stress relationship diagram of fine sandstone. (B) Strength envelope plot of fine sandstone.
4.5 Rock acoustic properties test
Using ultrasonic pulse transmission method, the propagation time of longitudinal or transverse waves along the sample length direction is measured (Figure 5), and the longitudinal and transverse wave velocities of the sample are calculated to obtain the elastic modulus and Poisson’s ratio of the sample under a given confining pressure. (Liu et al., 2009; Zuo et al., 2011; Han et al., 2017). The rock acoustic properties test (Table 7) demonstrates that with the increase of confining pressure, both longitudinal and transverse wave velocities, as well as the elastic modulus, increase.
4.6 Magnitude of geostress and its well logging evaluation
In this study, a total of 8 core samples underwent differential strain testing and analysis. The test results (Table 8) indicate that the vertical principal stress gradient is 0.025 MPa/m, the average gradient of the maximum horizontal principal stress gradient is 0.020 MPa/m, and the average gradient of the minimum horizontal principal stress gradient is 0.016 MPa/m. The results of the differential strain test show that the vertical principal stress is greater than the maximum horizontal principal stress.
5 Discussion
5.1 The influence of confining pressure on mechanical parameters in rocks
5.1.1 The influence of confining pressure on compressive strength in rocks
Analyzing the influence of confining pressure on compressive strength, it is observed that there is a positive correlation between the compressive strength of the rock and the confining pressure (Figure 6A). According to the Mohr-Coulomb criterion, during the compressive failure of the rock under confining pressure (σ3), it satisfies the following relationship with the maximum axial stress (σ1):
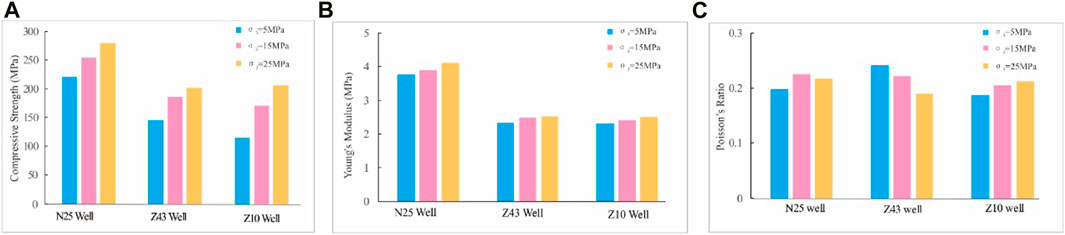
FIGURE 6. The influence of confining pressure on rock mechanics parameters. (A) Confining pressure—compressive strength; (B) Confining pressure—Young’s modulus; (C) Confining pressure—Poisson’s ratio.
In the equation, K = ctg (45°-θ/2);
θ—the internal friction angle of the rock, °;
C—the cohesion of the rock, MPa.
When the rock reaches critical failure, σ1 represents the triaxial compressive strength of the rock. Since the internal friction angle and cohesion are inherent properties of the rock and independent of confining pressure, the compressive strength of the rock under triaxial compression will increase linearly with the increase in confining pressure (Duan et al., 2023).
5.1.2 The influence of confining pressure on Young’s modulus in rocks
Analyzing the influence of confining pressure on Young’s Modulus, it can be observed that the Young’s modulus increases with the increase in confining pressure (Figure 6B). Due to the confinement’s limiting effect on strain, as the confining pressure increases, the axial strain decreases under the same axial stress (Wang et al., 2016) (Figure 7). Consequently, Young’s modulus increases with the increase in confining pressure.
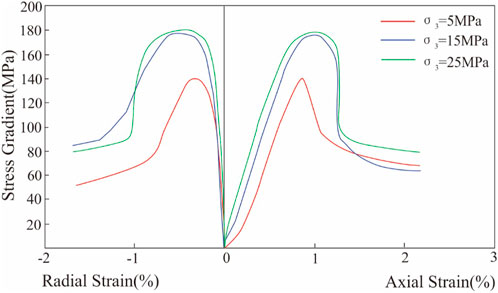
FIGURE 7. Triaxial compression stress-strain curves of Z43 well samples at different confining pressures.
5.1.3 The influence of confining pressure on Poisson’s ratio in rocks
Analyzing the influence of confining pressure on Poisson’s Ratio, it can be observed that with the increase in confining pressure, the Poisson’s ratio of the N25 well sample first increases and then decreases (Figure 6C). For the Z43 well sample, the Poisson’s ratio decreases with the increase in confining pressure, while for the Z10 well sample, the Poisson’s ratio increases with the increase in confining pressure. From the figures, it is evident that under the same axial stress, with the increase in confining pressure, both axial strain and radial strain decrease, but the reduction ratio is not consistent. This irregular variation in Poisson’s ratio is attributed to the effects of pore and microcrack closure.
5.2 Correlation between dynamic and static rock mechanical parameters
The rock triaxial compression test can provide static elastic parameters, while the rock acoustic wave test can provide dynamic elastic parameters. By utilizing the correlation between dynamic and static elastic parameters, dynamic elastic parameters obtained from logging and other methods can be converted into static elastic parameters (Zhong, et al., 2012; Wang, et al., 2020). This approach not only avoids expensive experimental costs but also allows obtaining continuous static elastic parameter values. Analyzing the relationship between dynamic elastic modulus and static elastic modulus, the mathematical relationship can be derived (Figure 8):
The static elastic modulus is 0.9015, the dynamic elastic modulus is 0.0489, and the correlation coefficient between them is 84.41%.
It can be inferred that the dynamic and static Young’s moduli have a strong correlation. Furthermore, by analyzing the acoustic data from wells N25, Z43, and Z10, it is evident that with the increase in confining pressure, both longitudinal and transverse wave velocities, as well as elastic modulus, increase. Additionally, the mutual comparison of dynamic and static mechanical parameters of rocks can enhance the effectiveness of their utilization in oilfield development.
5.3 Impact of present-day geostress orientation on development
In oilfield development, the present-day geostress plays a crucial role as it represents the internal stress state within the crust. It is composed of factors such as formation gravity, formation pressure, tectonics, and pore fluids. Accurate understanding of stress orientation is of paramount importance for devising hydraulic fracturing and optimizing injection-production well layouts in development projects (Ge, et al., 1998; Zu, et al., 2014; Wang, et al., 2022b).
In this study, the orientation of drilling-induced fractures was directly determined using Formation Micro-Imaging (FMI) technology to ascertain the maximum principal geostress direction (Figure 9). This technique utilizes drilling-induced fractures formed during the drilling process to determine the direction of geostress. Due to the anisotropy of horizontal stress in geological formations, it results in the formation of maximum and minimum stress vector fields in the horizontal direction. The direction of the drilling-induced fractures corresponds to the direction of maximum horizontal geostress in the formation. Analysis of well Z233 in the study area revealed that the direction of maximum horizontal principal stress ranged from 77° to 98°, with an average of 95°, aligning closely with an east-west direction (Figure 9).
The Chang 7 member reservoir in the study area is a tight sandstone reservoir with poor properties and limited natural fractures, making hydraulic fracturing a critical production enhancement technique. In hydraulic fracturing, the morphology and extension of induced fractures are influenced by the geostress. Generally, these fractures are perpendicular to the minimum horizontal principal geostress and aligned with the maximum horizontal principal geostress. Consequently, fractures resulting from hydraulic fracturing also exhibit an east-west distribution. Due to the alignment of hydraulic fractures with the direction of maximum principal geostress, fluid flow within fractures will be dominant along this direction. Hence, post-fracturing well layout should consider the direction of maximum fluid flow. For tight sandstone reservoirs, the direction of hydraulic fractures corresponds to the direction of maximum fluid flow. Therefore, in well network design, the direction of maximum principal stress should be the primary consideration to achieve effective oil and gas production.
Conclussion
1) Experimental analysis of the Chang 7 tight oil reservoir in Longdong shows rock densities of 2.543 g/cm³ for fine sandstone and 2.597 g/cm³ for muddy siltstone, with tensile strengths of 6.43 MPa and 4.08 MPa, respectively. Uniaxial compression tests reveal compressive strengths of 137.38 MPa for fine sandstone and 108.969 MPa for muddy siltstone, while triaxial tests highlight key mechanical parameters such as elastic modulus and Poisson’s ratio.
2) The study finds a strong positive correlation between compressive strength, Young’s modulus, and confining pressure. However, Poisson’s ratio varies irregularly with confining pressure. This relationship between dynamic and static mechanical parameters suggests a method for estimating static elastic parameters from well log data.
3) Employing differential strain and imaging well log techniques, the study determines the magnitude and orientation of geostress. This information is crucial for optimizing oilfield fracturing processes and planning well network layouts.
Data availability statement
The original contributions presented in the study are included in the article/Supplementary Material, further inquiries can be directed to the corresponding author.
Author contributions
RW: Investigation, Resources, Writing–review and editing. YT: Writing–original draft.
Funding
The author(s) declare that no financial support was received for the research, authorship, and/or publication of this article.
Conflict of interest
The authors declare that the research was conducted in the absence of any commercial or financial relationships that could be construed as a potential conflict of interest.
Publisher’s note
All claims expressed in this article are solely those of the authors and do not necessarily represent those of their affiliated organizations, or those of the publisher, the editors and the reviewers. Any product that may be evaluated in this article, or claim that may be made by its manufacturer, is not guaranteed or endorsed by the publisher.
References
Chen, G. Q., Li, H., Wei, T., and Zhu, J. (2021). Searching for multistage sliding surfaces based on the discontinuous dynamic strength reduction method. Eng. Geol. 286 (4), 106086. doi:10.1016/j.enggeo.2021.106086
Duan, S. Q., Sun, Y. D., Xiong, J. C., and Gao, P. (2023). A review of research on the criteria of high geostress and its influencing factors. Chin. J. Under. Space Eng. 19 (3), 1038–1050.
Gao, Y., Guo, P., Li, X., Li, Y. Y., Xu, D. S., Zou, Z. Y., et al. (2022). Investigation of triaxial compression failure and acoustic emission characteristics of different reservoir rocks. J. Eng. Geol. 30 (4), 1169–1178. doi:10.13544/j.cnki.jeg.2022-0177
Gao, Y., Wang, Y. W., Wang, Y. D., Xie, T. S., Liu, G., and Wang, L. (2016). Rock mechanics characteristics of Lucaogou tight oil reservoir in jimusaer Sag, Junggar Basin. Xinjiang Pet. Geol. 37 (2), 158–162. doi:10.7657/xjpg20160206
Ge, H. K., Lin, Y. S., and Wang, S. C. (1998). Geostresses determination technique and its applications in petroleum exploration and development. J. Univ. Pet. Chin. Ed. Nat. Sci. 22 (1), 94–99.
Han, W. G., Xiao, J., Cui, Z. D., Si, K., Wang, B. N., and Zhang, J. Y. (2017). Acoustic emission characteristics of tight sandstone during failure processes with different confining pressures. J. Eng. Geol. 25 (5), 1270–1278. doi:10.13544/j.cnki.jeg.2017.05.012
He, T. P., and Yun, Y. P. (2021). A comparative study on the composition of clastic and the parent rock of the source area in the yanchang Formation in the Longdong area of the Ordos Basin. Earth Sci. 110 (2), 64–69. doi:10.11648/j.earth.20211002.13
Li, H. (2022). Research progress on evaluation methods and factors influencing shale brittleness: a review. Energy Rep. 8, 4344–4358. doi:10.1016/j.egyr.2022.03.120
Li, X., Wang, K. Y., Wang, J. G., Yang, S. Y., Zhang, Q. H., and Zhang, Q. (2022). Impact of lithologic heterogeneity on brittleness of cenozoic unconventional reservoirs (Fine-Grained) in western Qaidam Basin. Miner 12 (11), 1443. doi:10.3390/min12111443
Liu, J. P., Wang, H. Y., Yang, Y. J., and Li, Y. H. (2009). Experimental study on different rock locating algorithms with acoustic emission. J. Northeast. Univ. Nat. Sci. 30 (8), 1193–1196. doi:10.1360/972009-1549
Liu, Z., Shi, B., Ge, T., Sui, F., Wang, Y., Zhang, P., et al. (2022). Tight sandstone reservoir sensitivity and damage mechanism analysis: a case study from Ordos Basin, China and implications for reservoir damage prevention. Energy Geosci. 3 (4), 394–416. doi:10.1016/j.engeos.2021.05.001
Wang, P. T., Liu, C., Ma, C., Ren, F. H., and Cai, M. F. (2023). On the anisotropic mohr-coulomb criterion of fractured rock masses based on the discrete fracture network. Chin. J. Rock Mech. Eng. 42 (S1), 3266–3280. doi:10.13722/j.cnki.jrme.2022.0627
Wang, S., Dai, J. S., Fu, X. L., Wang, Y., Chen, G. Q., and Xu, F. G. (2016). Numerical simulation research on current stress of Es3 of the 5th block of bonan oilfield and analysis of its influence factors. Pet. Geol. Recovery Effic. 23 (3), 26–32. doi:10.13673/j.cnki.cn37-1359
Wang, S. L., Li, H., Lin, L. F., and Yin, S. (2022a). Development characteristics and finite element simulation of fractures in tight oil sandstone reservoirs of yanchang Formation in western Ordos Basin. Front. Earth Sci. 9, 823855. doi:10.3389/feart.2021.823855
Wang, X. H., Feng, Z. C., and Liu, Z. H. (2009). Study on distribution laws of rock density. Chin. J. Rock Mech. Eng. 28 (A02), 3484–3489. doi:10.3321/j.issn:1000-6915.2009.z2.029
Wang, X. X., Hou, J. G., Li, S. H., Dou, L. X., Song, S. H., Kang, Q. Q., et al. (2020). Insight into the nanoscale pore structure of organic-rich shales in the bakken formation, USA. J. Pet. Sci. Eng. 191, 107182. doi:10.1016/j.petrol.2020.107182
Wang, X. X., Yu, S. Y., Li, S. H., and Zhang, N. D. (2022b). Two parameter optimization methods of multi-point geostatistics. J. Pet. Sci. Eng. 208, 109724. doi:10.1016/j.petrol.2021.109724
Xiong, J., Wu, J., Liu, X. J., Zhang, L., and Liang, L. X. (2023). Investigation on the geomechanical characteristics of the continental shale reservoirs and its influence on the fracturing effect. J. Southwest Pet. Univ. Sci. Technol. Ed., doi:10.11885/j.issn.1674.5086.2021.12.28.01
Yang, J. R., Liu, X. Y., and Xu, W. L. (2022). Reservoir forming dynamics of differential accumulation of tight oil in the yanchang formation Chang 8 member in the Longdong area, Ordos Basin, Central China. Front. Earth Sci. 9, 1414. doi:10.3389/feart.2021.788826
Zhang, K. S., Tang, M. R., Wang, C. W., Wang, G. T., Dou, L. B., and Sun, H. B. (2021). Study on prediction method of tensile strength for tight sandstone formation. Prog. Geophys. 36 (1), 318–324. doi:10.6038/pg2021EE0076
Zhang, P., Xu, D., Fu, X., Xie, J., Dong, Y., and Zhang, X. (2022a). Evaluation of hydraulic conductivity based on fault confinement studies. J. Min. Strata Control Eng. 4 (2), 23–33. doi:10.13532/j.jmsce.cn10-1638/td.20211215.001
Zhang, X. J., He, J. H., Xu, Q. L., Ye, T. R., Deng, H. C., Xu, Z. Q., et al. (2022b). Distribution characteristics and disturbance mechanism of present geostress field in the second member of xujiahe Formation in hechuan area. Min. Petrol. 42 (4), 71–82. doi:10.19719/j.cnki.1001-6872.2022.04.07
Zhao, Z., Shou, Y. D., and Zhou, X. P. (2023). Microscopic cracking behaviors of rocks under uniaxial compression with microscopic multiphase heterogeneity by deep learning. Int. J. Min. Sci. Technol. 33 (4), 411–422. doi:10.1016/j.ijmst.2022.12.008
Zhong, D. K., Zhou, L. J., Sun, H. T., Yao, J. L., Liu, X. Y., Luo, A. X., et al. (2013). Petrology of sandstone reservoirs in Longdong area, Ordos Basin. Earth Sci. Front. 20 (2), 52–60.
Zhong, D. K., Zhou, L. J., Sun, H. T., Yao, J. L., Ma, S. Y., and Zhu, H. H. (2012). Influences of petrologic features on diagenesis and pore development: an example from the triassic yanchang Formation in Longdong area, Ordos Basin. Oil Gas. Geol. 33 (6), 890–899. doi:10.11743/ogg20120610
Zu, K. W., Zeng, L. B., Liu, X. Z., Zhang, J. H., Zhao, X. Y., and Liu, G. P. (2014). Analysis of influencing factors for Ground stress in channel sandstone. J. Geomech. 20 (2), 149–158. doi:10.3969/j.issn.1006-6616.2014.02.006
Keywords: Ordos Basin, Chang 7 member, tight oil reservoir, rock physical mechanical properties, experiments
Citation: Wang R and Tang Y (2024) Study on the rock physical mechanical properties evaluation of tight oil reservoir in Chang 7 member, Longdong area, Ordos Basin, China. Front. Earth Sci. 12:1342561. doi: 10.3389/feart.2024.1342561
Received: 22 November 2023; Accepted: 29 January 2024;
Published: 23 February 2024.
Edited by:
Wenlong Ding, China University of Geosciences, ChinaCopyright © 2024 Wang and Tang. This is an open-access article distributed under the terms of the Creative Commons Attribution License (CC BY). The use, distribution or reproduction in other forums is permitted, provided the original author(s) and the copyright owner(s) are credited and that the original publication in this journal is cited, in accordance with accepted academic practice. No use, distribution or reproduction is permitted which does not comply with these terms.
*Correspondence: Ruifei Wang, c2lyd3JmMjAwM0AxNjMuY29t