- 1Department of Water and Water Structures Engineering, Faculty of Engineering, Zagazig University, Zagazig, Egypt
- 2Drainage Research Institute (DRI), National Water Research Center (NWRC), Cairo, Egypt
- 3CERIS, Instituto Superior Técnico, Universidade de Lisboa, Lisbon, Portugal
- 4Department of Civil and Environmental Engineering, Brunel University London, Uxbridge, United Kingdom
Groundwater is an important water supply for households, industry, and agriculture: one-third of the world’s drinking water is sourced from groundwater. The main objective of this study is to investigate the effects of groundwater flow directions on the design of drainage plans. The study involved field investigation to measure modeling input parameters and numerical modeling using the groundwater modeling system (GMS) code. It examined the effects of a subsurface drainage system, including net drainage recharge rate, equivalent drain depth, and drain spacing. This research was applied to the real-world case of the Mashtul Pilot Area (MPA) in Egypt. The results showed that the lateral arrangement had a high performance by orienting at 90°, 135°, and 45° angles. The groundwater levels reached 5.63, 5.33, and 5.51 m above mean sea level for the three orientations, respectively, at the baseline case. Also, the results showed that decreasing the drainage rates and increasing the equivalent drain depth have a greater effect on increasing groundwater levels, while increasing drain spacing led to an increase in the groundwater levels in the study. This approach is useful for the future arrangement of lateral subsurface drainage systems, which should be laid perpendicular to the direction of groundwater flow. In addition, the feasibility of this approach should be considered in the future design of this system. The results of the current study are useful for all stakeholders.
1 Introduction
Water is a necessity for population growth and continuous development. It is difficult to meet water demand when available water resources are almost constant (El Bedawy, 2014; Abd-Elaty et al., 2022). Groundwater, or subsurface water, refers to all water found beneath the Earth’s surface and is a component of the hydrological cycle. Water resources have become a vital energy source in the 21st century as water demand increases in agriculture, industry, households, recreation, and the environment, but their availability has decreased due to climate change (El-Atfy, 2007; Gleick, 2000; Abd-Elaty et al., 2024). Groundwater is an important component of a country’s water resources. Groundwater stores over 30% of the world’s freshwater supplies, while surface water resources such as rivers, lakes, and soil moisture store less than 1% (Taylor et al., 2013). The remaining freshwater supply is locked up in polar ice and glaciers. Because groundwater remains hidden, it is often overlooked.
Egypt’s irrigation, industrial, and domestic water demand exceeds the Nile’s supply. This is replaced by multiple reuses of fresh water, indicating a shortage of freshwater resources and reflecting the system’s high efficiency and sensitivity to water quality problems (El Bedawy, 2014; Mishra et al., 2021). The Nile River provides approximately 95% of Egypt’s total water demand, including water-intensive irrigation for agriculture, which is limited to the narrow corridor of the river and delta (El-Ramady et al., 2013).
Surface and subsurface drainage are two types of drainage systems. Surface drainage has the advantage of directly receiving precipitation. Nowadays, Egypt is entering a new phase in which human food needs must harmonize with environmental and sustainability principles. Drainage impacts on the environment have given rise to much concern, so in the future, the design and operation of drainage systems should satisfy both agricultural and environmental objectives (Abd-Elaty et al., 2017). On the other hand, subsurface drainage has many advantages, such as land reclamation, long life, and low maintenance costs, which is why it is preferred (Sallam, 2019; Ritzema et al., 2008). A national program was started after the government became convinced that equipping agricultural land with field drains would increase crop yields while preventing waterlogging and soil salinization. The Egyptian Public Authority for Drainage Projects (EPADP) was established in 1970 as a separate drainage authority of the Ministry of Irrigation to manage the construction of drainage projects in Egypt (Abdel-Dayem et al., 2007). Up to 1995, EPADP provided 1.81 million hectares (4.3 million feddan) of agricultural land with pipe drainage, and another 0.88 million hectares (2.1 million feddans) were to be constructed between 1996 and 2010. By 2010, an estimated 2.69 million hectares (6.4 million feddans) were served by subsurface drainage systems (Abd-Elaty et al., 2010; Sallam and Abd-Elaty, 2012; Abd-Elaty et al., 2019).
Figure 1 presents a typical drainage system consisting of open and covered (lateral) drains, which collect water within the field and discharge it into open or pipe collector drains. The collector drains discharge water into a carrier network of main open drains that convey water and transfer it within pumping stations to coastal lakes or the sea (Sallam, 2019).
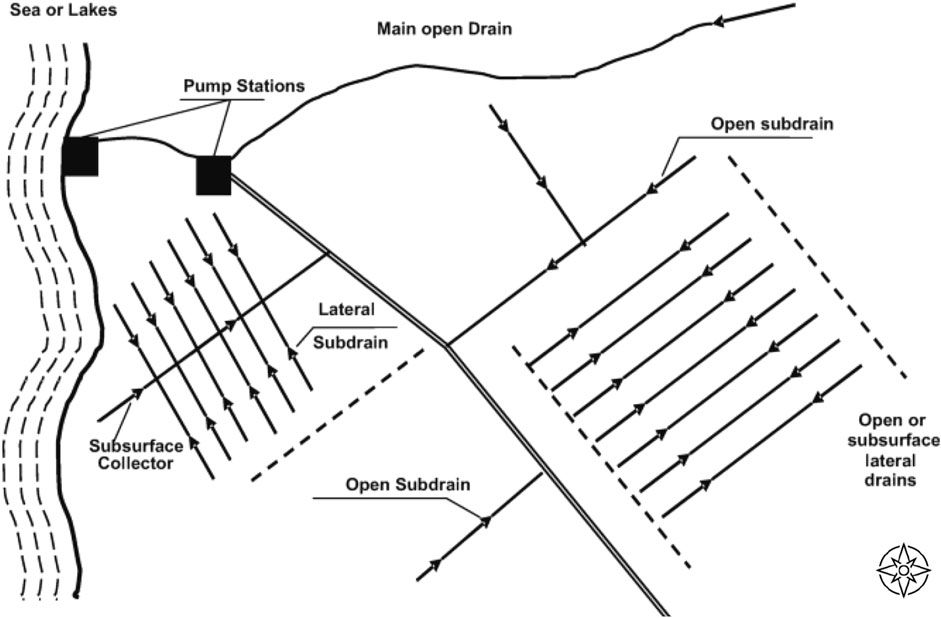
Figure 1. Schematic presentation of a typical surface and subsurface drainage network, after Sallam (2019).
Several studies indicate that waterlogging and salinity problems are the result of poor water management in irrigated agriculture. These problems have a negative impact on crop production, especially in arid and semi-arid regions. Decision-makers around the world have realized that the absence of adequate drainage is the reason for declines in crop production. Therefore, they have undertaken large-scale projects to install drains (Abdel-Dayem, 1994). Drainage projects have direct and indirect positive and negative impacts. The expert evaluation of Egypt’s subsurface drainage system has indicated that the environmental impact assessment of such projects, given the current feasible conditions and substantial benefits, is highly likely to have a positive impact (85.50%), no impact (6.5%), and a negative impact (5%) (Abd-Elaty et al., 2017). Negative impacts, including the pollution of drainage water with salts, nutrients, organic compounds, and harmful substances, are accompanied by the benefits of the subsurface drainage system (Sallam and Abd-Elaty, 2012). In addition, the higher the hydraulic conductivity of the soil, the lower the GWT (Negm et al., 2017).
Soil becomes waterlogged when water is unable to drain away. This leaves no air spaces in the saturated soil, so plant roots literally drown. Waterlogging is common on naturally poorly drained soils or when heavy soils are compacted. The problem of a rise in the groundwater table leads to water logging due to seepage and increased levels of salinization (Tewari et al., 1997). Particularly in arid and semi-arid regions, soil salinity adversely impacts crop growth and yield and causes land degradation (Gerba and And Pepper, 2019). By using irrigation water with a high salt content, humans can either naturally cause or contribute to soil salinity results from the weathering of rocks and fundamental minerals (Shahid et al., 2018). The connection between waterlogging, soil salinization, and plant yields has been the subject of numerous studies. Because waterlogged soil stops the salt imports of irrigation water from leaching, soil salinization can thus occur.
Dadgar et al. (2020) studied the potential groundwater recharge from the deep drainage of irrigation water and found that at a depth of 10 m, the flux showed some continuity (base flux) during a long-term recharge simulation. In total, 12.7% of the input water contributed to the recharge of the groundwater. Toro et al. (2022) studied groundwater flow patterns in a coastal fen exposed to drainage, rewetting, and interaction with the Baltic Sea. The results showed that ditches still influence flow pathways in rewetted coastal peatlands and that about 30% of groundwater originates from infiltrated seawater, irrespective of age. Guo et al. (2024) developed field and numerical experiments of subsurface drainage systems in saline and low-permeability interlayered fields in arid regions. They found that the buried depth and spacing of drainage pipes and the saturated hydraulic conductivity of the low-permeability interlayer had significant effects on the rate of desalination (P < 0.01).
In contrast, the position of the low-permeability interlayer had no significant effect (P > 0.05); subsurface drainage pipes should be placed below it. Lai et al. (2024) studied the optimal design of a subsurface conveyance system based on bio-ecological drainage system simulation; their results showed that the subsurface modular conduit in the study area has an optimal height of 0.45 m and is capable of attenuating peak flow at the downstream outlet by more than 60%. It is expected that the innovative modeling technique and the optimum design of a subsurface conveyance system will be of wide interest. Essien et al. (2024) studied the design and hydrologic performance estimation of highway filter drains using a novel analytical probabilistic model. The results showed that the relative differences between the analytical probabilistic model’s (APM) estimated and continuous simulation-determined runoff capture ratios for all the simulated design cases are less than 8.5%.
There is a gap in understanding how the direction of groundwater flow affects the effectiveness of drainage systems. Existing literature focuses on the design and operation of drainage networks, primarily considering surface and subsurface water flow patterns. However, there is a lack of comprehensive studies that specifically address how a change in direction relative to groundwater flow affects dewatering efficiency.
While drainage systems in Egypt and similar regions are crucial for containing waterlogging and salinization, there is a need for research that considers both agricultural productivity and environmental sustainability in its design and operation. Current research often prioritizes either agricultural benefits or environmental impacts without fully exploring how these objectives can be reconciled. While there are studies on the design parameters of subsurface drainage systems, such as depth, spacing, and hydraulic conductivity, there is a lack of comprehensive optimization approaches that consider different soil conditions, water quality aspects, and environmental impacts. Improved design methodologies could increase the efficiency and sustainability of drainage systems in different agricultural contexts.
The main objective of this study is to analyze how a change in the orientation of drainage systems (parallel or perpendicular to the groundwater flow) affects their performance. This will be achieved through numerical simulations with the GMS model, evaluating factors such as drainage efficiency, prevention of waterlogging, and possible effects on groundwater quality.
This research aims to address critical knowledge gaps related to the design and operation of drainage systems in arid and semi-arid regions, focusing on the direction of groundwater flow and its impact on agricultural and environmental sustainability. Such a focus can provide insights into optimizing drainage systems. By understanding how different orientations affect system performance, we can develop more effective strategies for managing waterlogging and salinization in agricultural areas.
In addition, this study aims to bridge the gap between agricultural productivity and environmental sustainability by exploring design scenarios that reconcile these goals. By applying advanced numerical modeling techniques, the study attempts to propose optimized approaches for the design of drainage systems. This includes the consideration of groundwater dynamics to maximize the efficiency of the system while minimizing negative impacts on the environment. The results could contribute to policy decisions and technical practices aimed at sustainable water management in Egypt and similar regions.
2 Materials and methods
2.1 Hooghoudt’s approach
The head between two drains is calculated using Equation 1 and is presented in Figure 2. The schematic of the subsurface drainage system indicating input model parameters includes (R) the irrigation rates, (Di) depth from the surface to the impermeable layer, (Dd) depth of drain from the surface, (Dm) water-table depth from the surface, (h) height of water-table above the water level in drain, (Ka) saturated hydraulic conductivity above the drain, (Kb) saturated hydraulic conductivity below the drain, (d) drain diameter and (L) the spacing between drains (Ellafi et al., 2021).
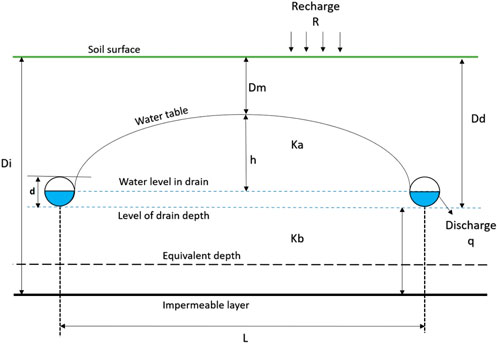
Figure 2. Vertical cross-section of a drainage system showing pipe placement, after Ellafi et al. (2021).
A drainage equation describes the relationship between the depth and spacing of parallel subsurface drainages, the depth of the water table, and the depth and hydraulic conductivity of the soil. It is used in the design of drainage systems. Hooghoudt’s distance equation is a well-known equation for steady-state drainage. It was first published in Dutch (Hooghoudt, 1940) and was popularized in 1957 by Van Schilfgaarde in the United States and formulated as follows (Equation 2):
where: L is the drain spacing (L); his groundwater height from the drain level (L); Kb is the hydraulic conductivity of the layer bottom drain level (LT−1); Ka is the hydraulic conductivity of the layer above the drain level (LT−1); q is the drainage rates (LT−1).
2.2 GM model
The GMS model was used to study and simulate the groundwater flow in steady state and transit flows for the Mashtul Pilot Area (MPA), Egypt. The partial differential equation for groundwater flow is used in the GMS model (McDonald and Harbaugh, 1998; Lv et al., 2022).
where: Kxx, Kyy, and Kzz are the hydraulic conductivity values along the x, y, and z coordinate axes (LT−1); h is the potentiometric head (L); Ss is the specific storage of the porous material (L−1); t is time (T); and Txx, Tyy, and Tzz are transmissivity values along the x, y, and z axes, respectively, equal to K * b in an unconfined aquifer. T = K * D* in a confined aquifer, q is the volumetric flux per unit volume representing source/sink terms. With q < 0.0 for discharge from the aquifer system and q > 0.0 for inflow (T−1), qs is volumetric flow per unit area representing source/sink terms. Ss is the specific storage coefficient defined as the volume of water released from storage per unit change in head per unit volume of porous material.
2.3 Field study area
To evaluate the impact of subsurface drainage projects, an experimental site representing the Nile Delta of Egypt was selected. The chosen experimental area of Mashtul Pilot Area (MPA) was constructed by the Drainage Research Institute (DRI) in 1980 in the south-east of the Nile Delta as an experimental field. The MPA is situated 70 km northeast of Cairo in a flat area (Figure 3) and is approximately 109.20 ha (260 feddans) in area.
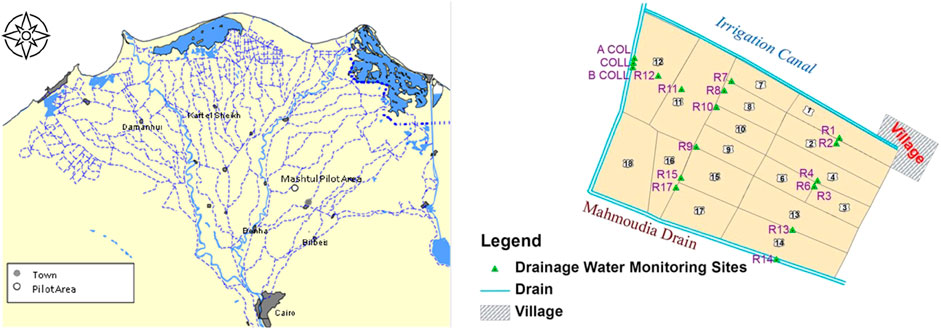
Figure 3. Location of Mashtul Pilot Area (DRI, 2004; El-Sadek et al., 2013).
The area has a subsurface drainage system consisting of parallel PVC lateral drains which discharge into buried concrete collector drains through a manhole as an experimental area. The design of the subsurface drainage system was made according to the standard criteria of the Egyptian Public Authority for Drainage Projects (EPADP). Two types of criteria can be distinguished: agricultural and technical. The agricultural criteria are a 1 m average depth of the groundwater table midway between the drains and an average drainage rate of 1.0 mm/day to permit sufficient leaching. The technical criteria are a design discharge rate for determining drain-pipe capacity of 4 mm/day for rice areas and 3 mm/day for non-rice areas and a safety factor of 25% in the design of the collector drains to account for sedimentation, misalignment, and changes in diameter. The selected drain depths are 1.20, 1.50, and 1.70 m for laterals and 2.5 m for collectors. Each unit was cultivated with a single crop for each unit each cropping season. Berseem and wheat were cultivated as winter crops, and cotton, rice, and maize as summer crops (DRI, 1987).
2.3.1 Climate and hydrological data
In the coastal regions, the annual temperature varies 14–37°C. Temperatures in the deserts vary from 6°C at night to 46°C during the day (Zaki and Swelam, 2017; Al-Gamal SEl-Sayed and Atta, 2018). Rainfall intensity in the study area is very limited because it is located in an arid climatic condition. The minimum average annual precipitation in Cairo is 25 mm/yr, and the maximum average annual rainfall in coastal areas is 133 mm/yr. Rainfall increases from south to north, with only a limited impact on groundwater recharge, apart from the Ismailia region (Eltarabily and Negm, 2017).
Three sources were considered for the recharge of groundwater in the study area. The first is rain, with an average rainfall of 25 mm/year (Mohamed, 2020). The second source is downward leakage due to excess irrigation water (originating from the River Nile); canal infiltration into the aquifer ranges 0.25–0.80 mm per day−1, depending on the soil type, irrigation, and drainage practices. The third source is the inter-aquifer flow of groundwater (Abdelhafez et al., 2020). Abstraction rates increased between 1980 and 2010 (Mohamed, 2020). Abstraction from the Nile Delta is linearly about 0.1 × 109 from 1980, except for 2003–2010 when the rate increased dramatically to 0.2 × 109 year−1 (Ding et al., 2020).
2.3.2 Model geometry, boundary conditions, and hydraulic parameters
A numerical model for the MPA was developed using MODFLOW software. The model is divided into 108 rows with 60 columns and five layers, with each cell dimension equaling (Δx * Δz) 25 m *25 m. The depth of the first layer, which presents the clay cap, was 10–20 m, while the second to fifth layers were divided into equal thicknesses of 20–267 m. The boundary conditions for the head were set in the model for the left side, in which the Mahmoudia drain forms the western boundary. The eastern boundary is formed by tertiary irrigation canals (Figure 4).
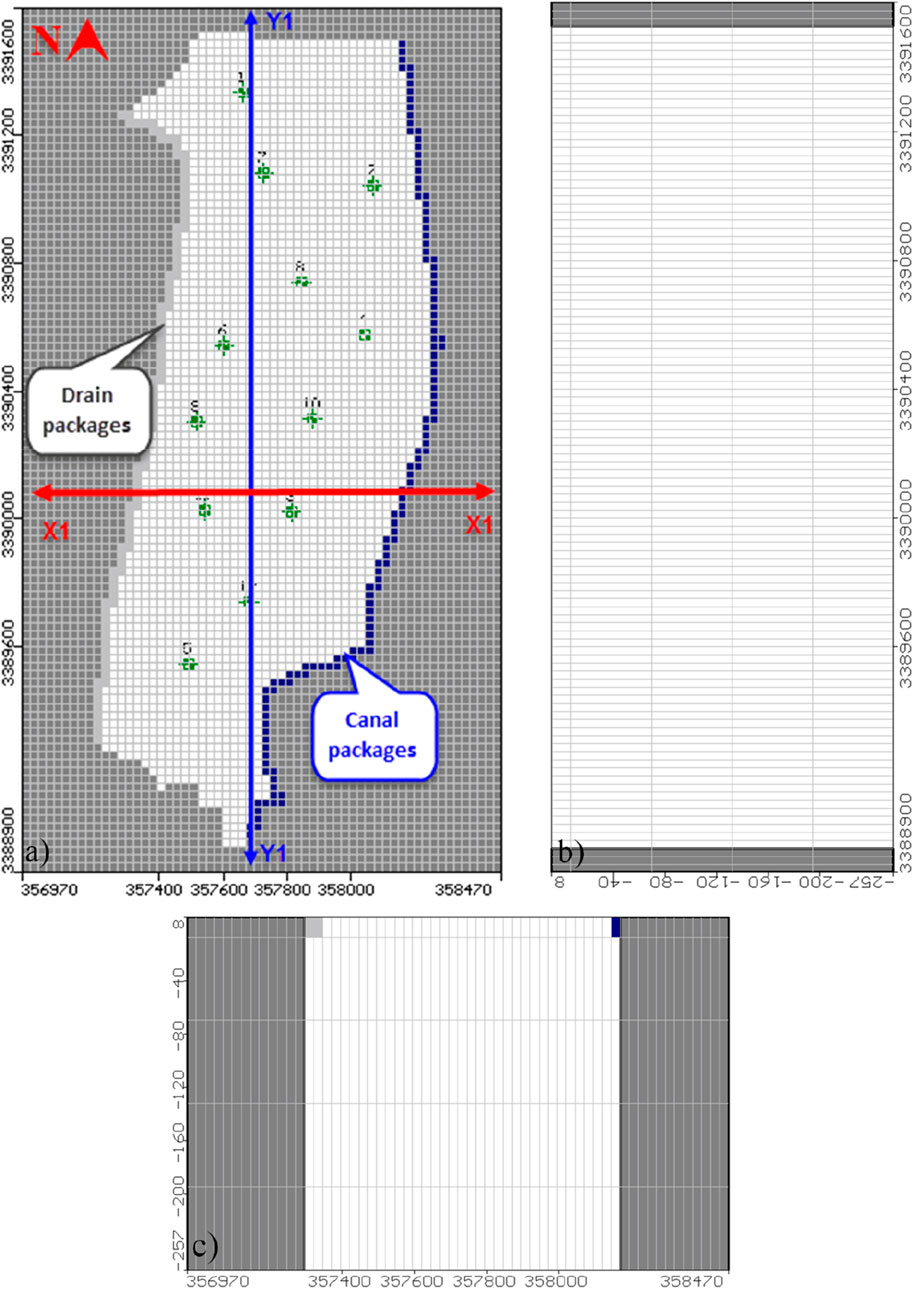
Figure 4. Model setup and boundary conditions for MPA: (A) top view, (B) vertical section X1-X1, and (C) vertical section X1-X1.
Morsy (2009) presented the hydraulic parameter values for the Nile Delta, including hydraulic conductivity in the horizontal (Kh) and vertical directions (Kv), specific yield (Sy), total porosity (n), and specific storage (Ss). Hydraulic parameters values were used in the numerical model (Table 1).
2.3.3 Model calibration
Under the above boundary conditions, the MODFLOW numerical model was applied to this case study of the MPA to simulate the groundwater flow system and flow direction. Figure 5A shows a realistic representation of the groundwater heads in the MPA case. The groundwater flow direction for the base case is also shown in Figure 5B.
For model calibration, the results for fall heights are compared with calculated fall heights at specific points in the study area. The calculated drop heights are assigned to the model at 12 observation wells.
The model was run under the same values of hydrological systems and observed groundwater heads, and the final results were from a steady state. The difference between calculated heads and observed ones. The figure indicates the residual range between −0.006 and −0.001 m with root mean square (RMS) at 0.004 m and normalization root mean square is 19.731%. Good agreement was found between the numerical model results and the calculated drop height.
2.4 Scenarios
A field case study of the MPA was used to investigate the effects of groundwater flow direction on the design of the drainage network. There were three scenarios to illustrate the best direction for the design of drains in relation to the movement of groundwater, for the orientations 45°, 90°, and 135°.
I. The drainage parameter for the recharge was decreased by 25% from the baseline case as a result of climate change.
II. The equivalent depth increased by 20 cm compared with the baseline case.
III. The drainage spacing was changed by changing the lateral distances to 120 m compared with 60 m at the base case.
3 Results
3.1 Field case simulation
The study investigates the influence of groundwater flow direction on drainage network design in the Mashtul Pilot Area (MPA) through detailed case studies. Three scenarios were developed to illustrate the direction of drainage design related to groundwater movement in the directions 45°, 90°, or 135° (Figures 6A–C). The results showed that the average groundwater level reached 5.63, 5.61, and 5.63 m (a.m.s.l), respectively, in which the optimal alignment of the drainage system was 90° (Figure 6E) compared to 135o (Figure 6F) and 45° (Figure 6D), (see Figure 10A).
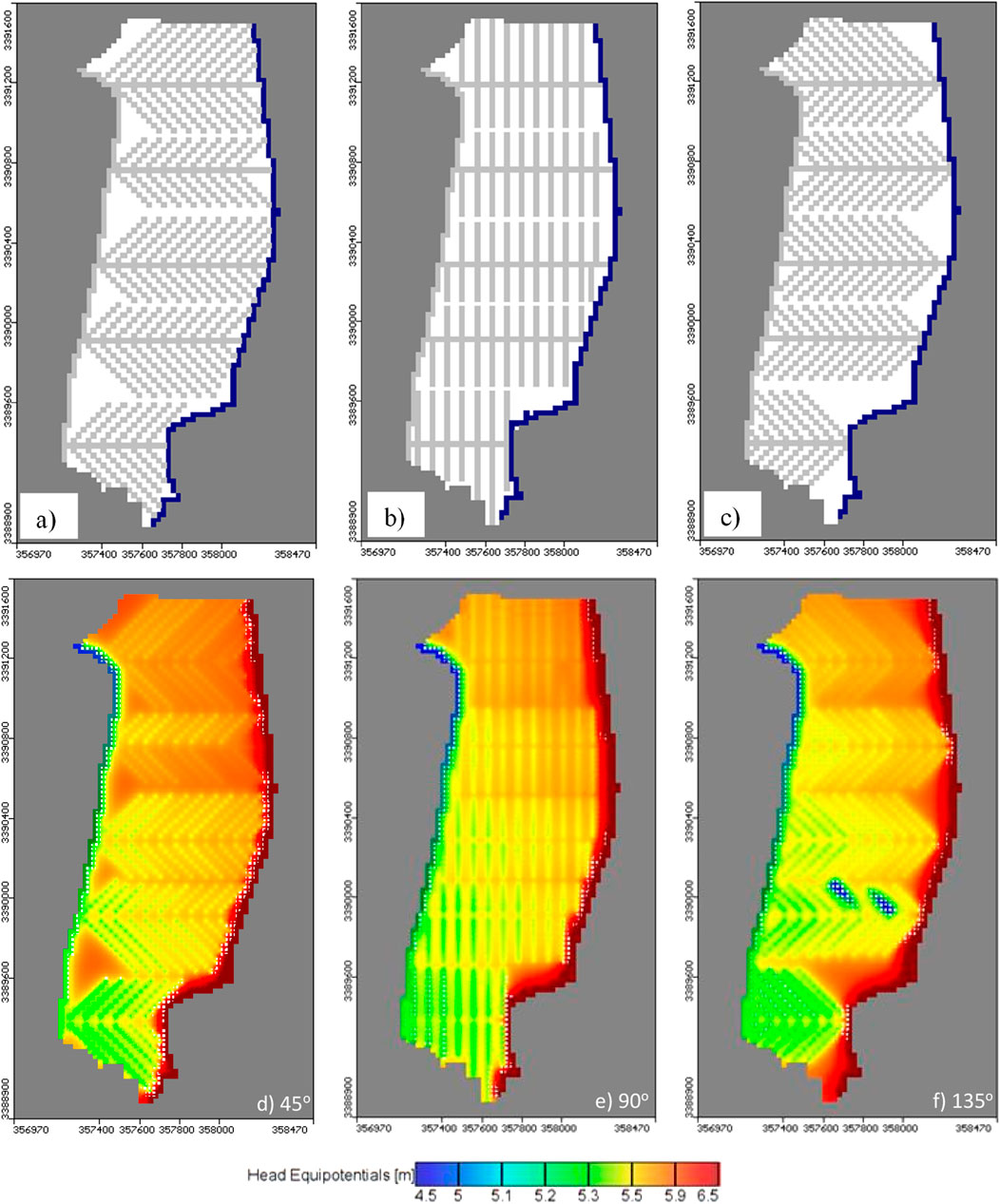
Figure 6. Relation between drain alignment and GWL in MPA. (A–C) show different alignment configurations, while (D–F) show the water head equipotentials for each alignment configuration.
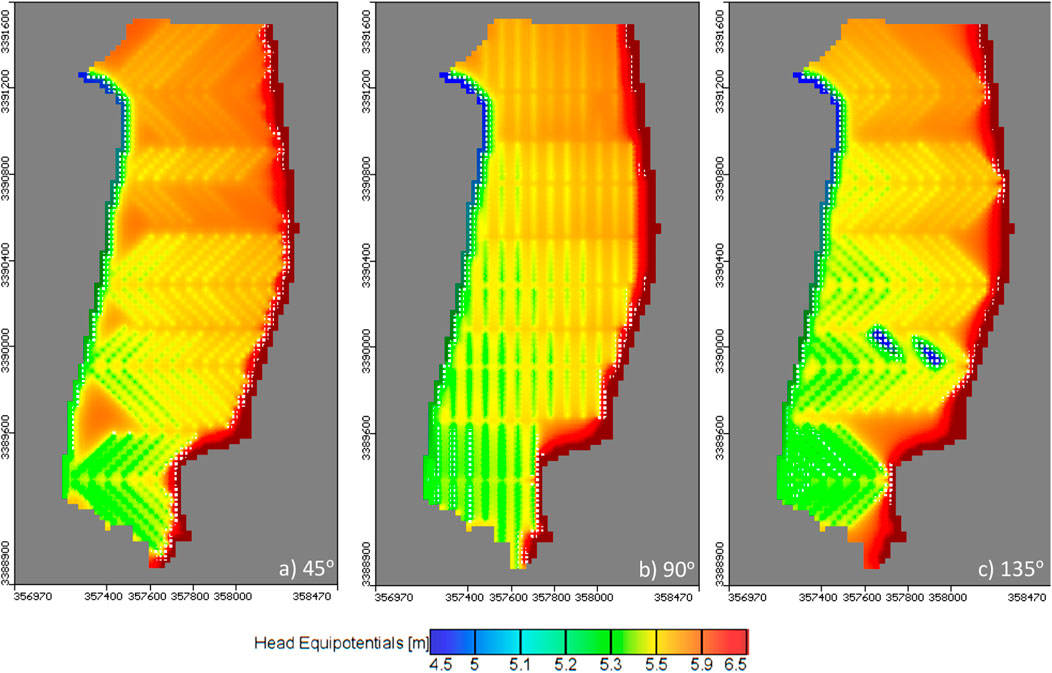
Figure 7. GWL for decreasing drainage rates by 25% in MPA for different alignment configurations. (A) 45°, (B) 90°, (C) 135°.
3.2 Impact of drain recharge
According to Li et al. (2016), boundary conditions greatly influence the direction of water flow and thus affect the design of discharges. As a result, Q is equal to 2.25 mm day−1 compared to 3 mm day−1 in the baseline case.
The results showed that the average groundwater level reached 5.57, 5.26, and 5.51 m (a.m.s.l) in the case of the 45°, 90°, and 135° alignments, respectively. Lateral alignment 90° was the best compared to 135° and 45° (Figure 7); the decrease in recharge resulted in a decrease in GWL (Figure 10B).
3.3 Impact of equivalent drain depth
The equivalent depth is determined by the drainage radius and drainage distance (L) (Van der Molen and Wesseling, 1991). Figure 8 shows the relationship between the groundwater levels and the 45°, 90°, and 135° alignments for drainage equivalent depth increased by 20 cm below the ground level.
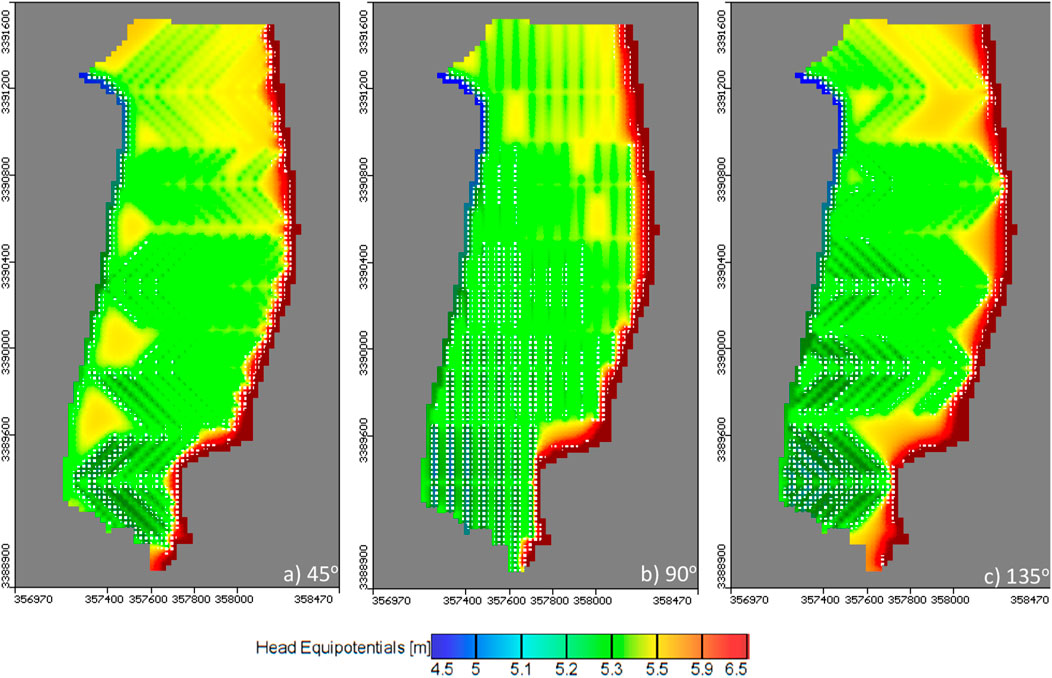
Figure 8. GWL for increasing equivalent drain depth by 20 cm in MPA for different alignment configurations. (A) 45°, (B) 90°, (C) 135°.
For example, when de was increased by 20 cm, the average groundwater level was 5.47, 5.15, and 5.35 m (a.m.s.l) in the respective 45°, 90°, and 135° orientations. In addition, the results indicate that the GWL decreases with increasing equivalent depth (Figure 10C).
3.4 Impact of drain spacing
The design drainage distance (L) was investigated in this study by changing the lateral distances to 120 m compared with 60 m at the baseline case. The results of the groundwater level reached 7.31, 6.88, and 7.14 m (a.m.s.l) at 45°, 90°, 135° orientations, respectively (Figure 9). In addition, the results indicate that the GWL increases with the increase in lateral drainage spacing (Figure 10D).
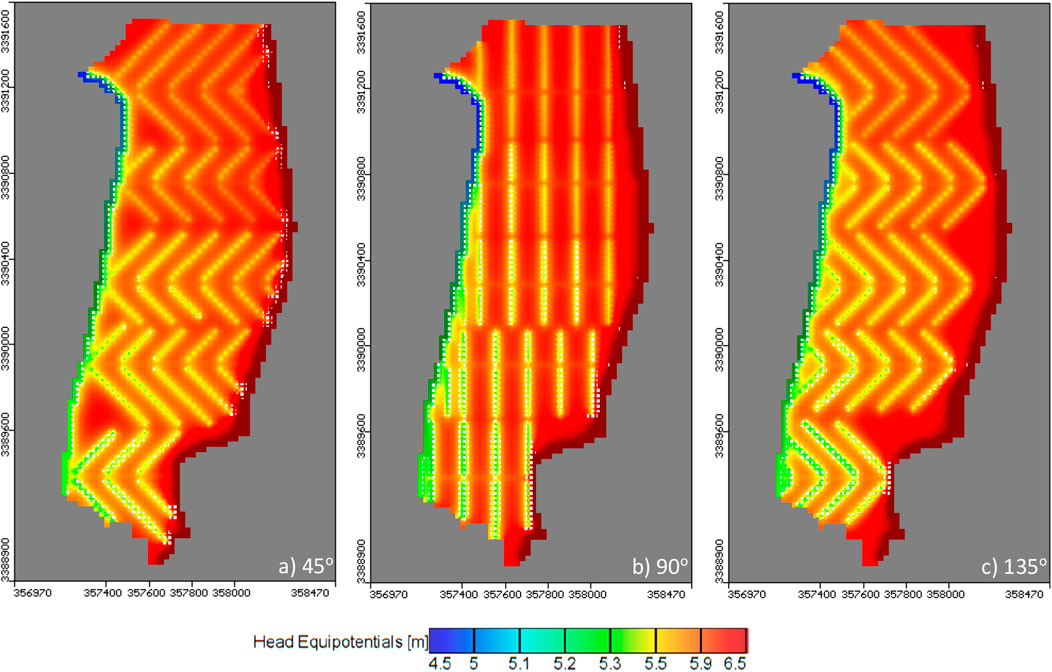
Figure 9. GWL for increasing drainage spacing to 120 m in MPA for different alignment configurations. (A) 45°, (B) 90°, (C) 135°.
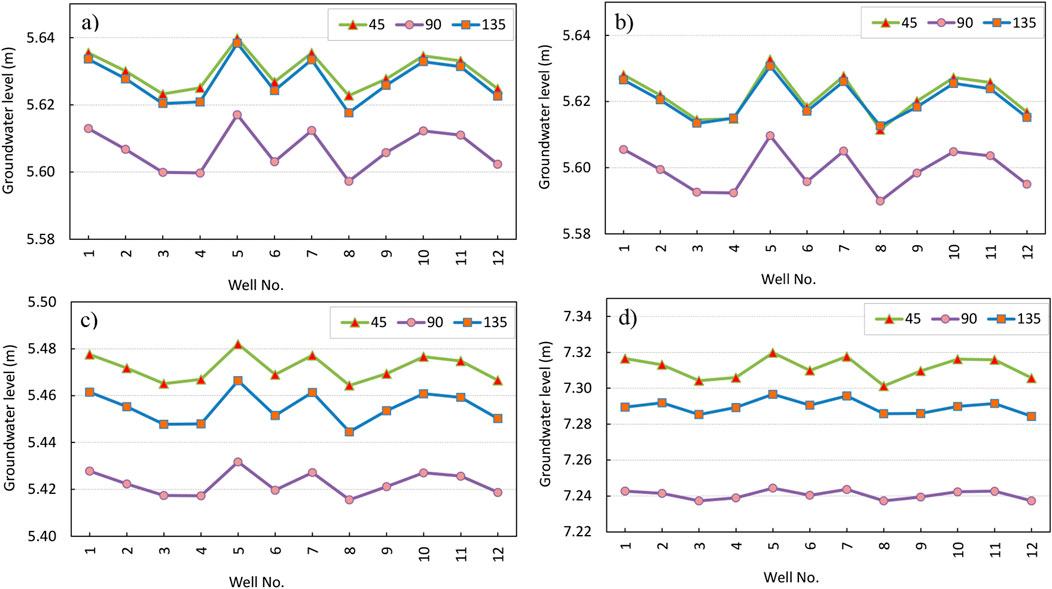
Figure 10. Relation between GWL and drainage alignment for MPA for (A) base case, (B) drain recharge rate, (C) equivalent drain depth, and (D) drain spacing.
4 Discussion
The study demonstrates the intricate relationship between subsurface drainage design and groundwater flow dynamics in the Mashtul Pilot Area (MPA), Egypt. By using Hooghoudt’s distance equation and MODFLOW software, the study provides a comprehensive analysis of different scenarios affecting groundwater level (GWL) and flow direction.
4.1 Influence of drain alignment on groundwater levels
Examination of the different drainage orientations—45°, 90°, and 135°—reveals that the 90° orientation consistently resulted in the lowest groundwater levels of the three orientations. In particular, it was found that the average groundwater level for the 90° orientation was 5.61 m, slightly lower than the 5.63 m observed for both 45° and 135°. This finding is consistent with the principle that a perpendicular orientation to the natural groundwater flow direction increases the efficiency of dewatering by reducing the resistance to flow and thus lowering the water table more effectively (Ellafi et al., 2021). These results suggest that aligning drainage at a 90° angle to the prevailing groundwater flow direction optimizes dewatering performance and water table management. Figure 10 presents the relation between the groundwater level and the observation well number. The GWL reached 5.63, 5.61, and 5.63 m (a.m.s.l) in the base case for the respective drain 45°, 90°, and 135° alignments (Figure 10A).
After studying these factors and in accordance with the relevant Egyptian code, the Ministry of Water Resources and Irrigation (MWRI) and the Drainage Research Institute (DRI) took into account the direction of groundwater movement with regard to the design of the drains. The DRI also modified parts of the layout in this area of MPA, in accordance with the results presented in Figure 11, which agree with our study findings.
The drainage system’s orientation depends on the soil’s topography and the direction of groundwater movement (Savenije, 2010). Moreover, the MPA results show that the best alignments of the subsurface drainage were, in order, at 90°, 135°, and 45° (Figure 11), where the groundwater level declined by an alignment of 90° more than in other cases. The current study’s conclusion that decreasing the number of surface water sources in the irrigation practice due to the future impact of climate change resulted in a decreased GWL and agreed with Youssef et al. (2018), in which low recharge rates led to a decrease in the distance between drains.
4.2 Impact of recharge reduction on drainage efficiency
The scenario considering a 25% reduction in recharge due to climate change showed a significant impact on groundwater levels. The results showed that lower recharge leads to lower groundwater levels at all orientations, with the 90° orientation again showing the most favorable results. The average groundwater levels were 5.57, 5.26, and 5.51 m for the 45°, 90°, and 135° orientations, respectively. This decrease in groundwater levels emphasizes the importance of considering future climate scenarios when designing and managing subsurface drainage systems (Abd-Elaty et al., 2019). The results show that a reduction in groundwater recharge can increase the effectiveness of subsurface drainage, further strengthening the 90° alignment as optimal under varying groundwater recharge conditions.
In addition, the GWL reached 5.57, 5.26, and 5.51 m (a.m.s.l) for the decrease of the drain recharge by 25% to be 2.25 mm day−1 compared to 3 mm day−1 (Figure 10B). Figure 10C shows the impact of increasing the drainage equivalent depth by 20 cm below the ground level; the groundwater level was 5.47, 5.15, and 5.35 m (a.m.s.l). Finally, Figure 10D presents the results of drain spacing of 120 m compared with 60 m at the baseline case, where the GWL reached 7.31, 6.88, and 7.14 m.
4.3 Effect of equivalent drain depth on groundwater levels
Increasing the equivalent discharge depth by 20 cm showed a noticeable effect on the water table, with the 90° alignment again showing the lowest values at 5.15 m compared to 5.47 m for 45° and 5.35 m for 135°. This scenario highlights the sensitivity of the water table to changes in discharge depth, as deeper discharges allow for greater groundwater abstraction. These results are consistent with previous studies (Van der Molen and Wesseling, 1991) which suggest that an increase in discharge depth increases the ability of the water table to lower, especially in areas with significant subsurface flow. Our findings for drain depths agree with Han et al. (2023), who showed that when subsurface drainage pipe spacing is constant, increasing the drainage outlet depth of subsurface drainage pipes can lower the groundwater level and effectively decrease soil salt accumulation.
4.4 Influence of drain spacing on groundwater levels
The analysis of the drainage distances, in which lateral distances of 120 m were compared with the baseline of 60 m, showed that increasing the distances leads to higher groundwater levels. The average groundwater levels for the 45°, 90°, and 135° alignments were 7.31, 6.88, and 7.14 m, respectively. This result indicates that a greater distance reduces the efficiency of the drainage system, resulting in higher groundwater levels (Han et al., 2023). The 90° alignment was also found to be the most effective at greater spacing, highlighting the importance of optimal alignment combined with adequate spacing between drains to maintain the desired water table. Moreover, the results for drain spacing agree with Kladivko et al. (2004), who showed that reducing drainage spacing creates a denser drainage network, enabling a more uniform distribution of the drainage system throughout the soil. This helps minimize the uneven distribution of salt in the soil, preventing the accumulation of salt in specific areas and facilitating the balanced removal of soil salt.
4.5 Practical implications and recommendations
The results of this study have significant implications for the design and management of subsurface drainage systems in similar arid and semi-arid regions. The optimal orientation of drainage systems at 90° to the natural groundwater flow direction, combined with considerations of future recharge scenarios and appropriate discharge depths and spacing, can significantly improve the efficiency of groundwater management (Han et al., 2023; Abd-Elaty et al., 2019). Furthermore, these results highlight the need for dynamic and adaptable drainage concepts that can respond to changing climatic conditions and varying hydrological parameters.
This study provides a detailed analysis of the influence of various factors on the performance of underground drainage systems. It also has limitations that should be considered in future designs and simulations. Thus, future research should focus on the long-term monitoring and evaluation of these drainage configurations to validate the results and further refine the design recommendations. Furthermore, this topic needs further research to better understand the relationship between soil properties and the performance of subsurface drainage systems. The effective operation of drainage systems is essential for efficient water management and flood prevention. The cost feasibility of changing drainage system layout should also be considered. Finally, the knowledge gained can guide future drainage projects to achieve optimal groundwater management and ensure agricultural productivity and sustainability in regions with similar hydrogeological conditions.
5 Conclusion
This study investigated the effects of different hydraulic parameters on subsurface drainage systems, focusing on the net recharge rate, the equivalent discharge depth, and the distance between discharges. The orientation of the drainage system plays a crucial role in lowering the water table and controlling waterlogging in the soil. Numerical analysis was used to simulate the water table and study the effects of different drainage system alignment configurations. The main results of this study are as follows.
• Effects of drainage orientation: The study shows that a 90° orientation of drainage systems consistently resulted in the lowest groundwater levels compared to other orientations. This orientation was found to be the most effective in lowering the water table as it optimized the drainage process and reduced flow resistance.
• Drainage recharge rates: Lower recharge rates, as simulated under future climate change scenarios, led to a decline in groundwater levels in all orientations, with the 90° orientation showing the most significant decline. This result underlines the importance of considering future climatic influences when planning drainage.
• Equivalent drainage depth: Increasing the equivalent drainage depth by 20 cm further lowered the groundwater level, with the 90° orientation achieving the greatest decrease. This result underlines the sensitivity of the water table to changes in discharge depth.
• Drainage spacing: The analysis showed that wider spacing between drains leads to higher groundwater levels, with the 90° alignment again performing best. Closer spacing creates a denser drainage network, which increases the efficiency of groundwater management.
Overall, the study shows that the groundwater table reacts very sensitively to discharge parameters. The optimal orientation for drainage systems should be perpendicular (90°) to the natural groundwater flow direction. This study acknowledges limitations, including cost feasibility and the need for further field investigations to substantiate its findings. Despite these limitations, the results provide valuable insights for engineering practice that support the planning and design of subsurface drainage systems to control groundwater dynamics and effectively mitigate flood risks. These findings have particularly significant implications for the design of subsurface drainage in arid and semi-arid regions. Future research should focus on long-term monitoring and evaluation to validate these results and further refine planning recommendations. Furthermore, understanding the relationship between soil properties and drainage performance is crucial for effective water management and agricultural productivity.
Data availability statement
The original contributions presented in the study are included in the article/supplementary material; further inquiries can be directed to the corresponding authors.
Author contributions
WA-E: conceptualization, data curation, formal analysis, investigation, methodology, project administration, software, supervision, validation, writing–original draft, and writing–review and editing. AE: data curation, investigation, visualization, and writing–review and editing. GS: investigation, project administration, visualization, writing–original draft, and writing–review and editing. AK: supervision, validation, investigation, visualization, and writing–review and editing. AA: funding acquisition, supervision, validation, visualization, investigation, and writing–review and editing. IA-E: investigation, methodology, visualization, and writing–original draft.
Funding
The authors declare that no financial support was received for the research, authorship, and/or publication of this article.
Acknowledgments
The authors are thankful to the Department of Water and Water Structures Engineering, Faculty of Engineering, Zagazig University, Zagazig 44519, Egypt, for constant support during the study.
Conflict of interest
The authors declare that the research was conducted in the absence of any commercial or financial relationships that could be construed as a potential conflict of interest.
Publisher’s note
All claims expressed in this article are solely those of the authors and do not necessarily represent those of their affiliated organizations, or those of the publisher, the editors, and the reviewers. Any product that may be evaluated in this article, or claim that may be made by its manufacturer, is not guaranteed or endorsed by the publisher.
References
Abd-Elaty, I., Gehan, A. H. S., Abdelaal, G. M., and Eldin, O. W. (2010). Environmental impact assessment of subsurface drainage projects in Egypt, the Egyptian intentional. Journal. Of engineering. Sci. .and Technol. 13 (2), 411–418.
Abd-Elaty, I., Kuriqi, A., Ramadan, E. M., and Ahmed, A. (2024). Hazards of sea level rise and dams built on the River Nile on water budget and salinity of the Nile Delta aquifer. J. Hydrology Regional Stud. 51 (2024), 101600. 2214-5818. doi:10.1016/j.ejrh.2023.101600
Abd-Elaty, I., Negm, A. M., and Sallam, G. A. H. (2017). “Environmental impact assessment of subsurface drainage projects,” in Unconventional water resources and agriculture in Egypt. The handbook of environmental chemistry. Editor A. Negm (Cham: Springer), 75, 59–85. doi:10.1007/698_2017_123
Abd-Elaty, I., Pugliese, L., Bali, K. M., Grismer, M. E., and Eltarabily, M. G. (2022). Modelling the impact of lining and covering irrigation canals on underlying groundwater stores in the Nile Delta, Egypt. Hydrol. Process. 36, e14466. doi:10.1002/hyp.14466
Abd-Elaty, I., Sallam, G. A., Strafacec, S., and Scozzari, A. (2019). Effects of climate change on the design of subsurface drainage systems in coastal aquifers in arid/semi-arid regions: case study of the Nile delta. Sci. Total Environ. J. 672 (672), 283–295. doi:10.1016/j.scitotenv.2019.03.483
Abdel-Dayem, M. S. (1994). “Concepts of design methods,” in Refreshing course on land drainage in Egypt, 10–19.
Abdel-Dayem, S., Abdel-Gawad, S., and Fahmy, H. (2007). Drainage in Egypt: a story of determination, continuity, and success. Irrigation Drainage J. Int. Comm. Irrigation Drainage 56, S101–S111. doi:10.1002/ird.335
Abdelhafez, A. A., Metwalley, S. M., and Abbas, H. H. (2020). “Irrigation: water Resources, types and common problems in Egypt,” in Technological and modern irrigation environment in Egypt: best management practices and evaluation. Editors E.-S. E. Omran, and A. M. Negm (Cham: Springer International Publishing), 15.
Al-Gamal Sel-Sayed, S., and Atta, E. (2018). Exploring surface-and groundwater interactions in East Delta Aquifer using conventional and non-conventional techniques. J. Appl. Geol. Geophys. 6, 48–58. doi:10.9790/0990-06020248
Dadgar, M. A., Nakhaei, M., Porhemmat, J., Eliasi, B., and Biswas, A. (2020). Potential groundwater recharge from deep drainage of irrigation water. Sci. Total Environ. 716 (2020), 137105. 0048-9697. doi:10.1016/j.scitotenv.2020.137105
Ding, Z., Koriem, M. A., Ibrahim, S. M., Antar, A. S., Ewis, M. A., He, Z., et al. (2020). Seawater intrusion impacts on groundwater and soil quality in the northern part of the Nile Delta, Egypt. Egypt Environ. Earth Sci. 79, 313. doi:10.1007/s12665-020-09069-1
DRI (Drainage Research Institute) (1987). Mashtul pilot area, physical description. Technical report no. 57, pilot areas and drainage Technology project. Cairo: Drainage Research Institute.
DRI (Drainage Research Institute), (2004). Drainage water status in the nile delta year book 2001/2002, Technical Report No. 70, June.
El-Atfy, H. (2007). Integrated national water resources plan in Egypt. Ministry Water Resour. Irrigation Alexandria Governorate.
El Bedawy, R. (2014). Water resources management: alarming crisis for Egypt. J. Mgmt. and Sustain. 4, 108. doi:10.5539/jms.v4n3p108
Ellafi, M. A., Deeks, L. K., and Simmons, R. W. (2021). Application of artificial neural networks to the design of subsurface drainage systems in Libyan agricultural projects. J. Hydrology Regional Stud. 35 (2021), 100832. 2214-5818. doi:10.1016/j.ejrh.2021.100832
El-Ramady, H. R., El-Marsafawy, S. M., and Lewis, L. N. (2013). “Sustainable agriculture and climate changes in Egypt,”. Sustainable agriculture reviews. Editor E. Lichtfouse (Dordrecht: Springer Netherlands), 12, 41–95. doi:10.1007/978-94-007-5961-9_2
El-Sadek, A., and Sallam, G.M. (2013). Developing DRAINMOD-geostatistical Technology to predict nitrate leaching. J. Irrigation Drainage Eng. 139 (2), 158–164. doi:10.1061/(ASCE)IR.1943-4774.0000491
Eltarabily, M. G., and Negm, A. M. (2017). Groundwater management for sustainable development east of the nile delta aquifer. Groundw. Nile Delta 73, 687–708. doi:10.1007/698_2017_102
Essien, A. E., Guo, Y., Khafagy, M., and Dickson-Anderson, S. E. (2024). Design and hydrologic performance estimation of highway filter drains using a novel analytical probabilistic model. Sci. Rep. 14, 2350. doi:10.1038/s41598-024-52760-7
Gerba, C. P., and And Pepper, I. L. (2019). “Municipal wastewater treatment,” in Environmentaland pollution science (Academic Press), 393–418.
Gleick, P. H. (2000). A look at twenty-first century water resources development. Water Int. 25, 127–138. doi:10.1080/02508060008686804
Guo, C., Yao, C., Wu, J., Qin, S., Yang, H., Li, H., et al. (2024). Field and numerical experiments of subsurface drainage systems in saline and low-permeability interlayered fields in arid regions. Agric. Water Manag. 300 (2024), 108898. 0378-3774. doi:10.1016/j.agwat.2024.108898
Han, D., Chen, C., Wang, F., Li, W., Peng, H., Jin, Q., et al. (2023). Effects of subsurface pipe drainage spacing on soil salinity movement in jiangsu coastal reclamation area. Sustainability 15 (18), 13932. doi:10.3390/su151813932
Hooghoudt, S. B. (1940). Algemeene beschouwing van het probleem van de detailontwatering en de infiltratie door middel van parallel loopende drains, greppels, slooten en kanalen. Versl. Landbouwk. Onderz. 46, 515–707.
Kladivko, E. J., Frankenberger, J. R., Jaynes, D. B., Meek, D. W., Jenkinson, B. J., and Fausey, N. R. (2004). Nitrate leaching to subsurface drains as affected by drain spacing and changes in crop production system. J. Environ. Qual. 33, 1803–1813. doi:10.2134/jeq2004.1803
Lai, S. H., Chin, R. J., Soo, E. Z. X., Ling, L., and Ghadim, H. B. (2024). Optimal design of subsurface conveyance system based bio-ecological drainage system simulation. KSCE J. Civ. Eng. 28, 2564–2570. doi:10.1007/s12205-024-1475-8
Li, X., Hu, B. X., and Tong, J. (2016). Numerical study on tide-driven submarine groundwater discharge and seawater recirculation in heterogeneous aquifers. Stoch. Environ. Res. Risk Assess. 30, 1741–1755. doi:10.1007/s00477-015-1200-8
Lv, Y., Jiang, J., Chen, L., Hu, W., and Jiang, Y. (2022). Elaborate simulation and predication of the tunnel drainage effect on karst groundwater field and discharge based on Visual MODFLOW. J. Hydrology 612, 128023. doi:10.1016/j.jhydrol.2022.128023
McDonald, M., and Harbaugh, A. W. (1988). A Modular Three-Dimensional Finite Difference Ground-Water Flow Model. In: Techniques of Water-Resources Investigations, Book 6, U.S. Geological Survey, 588.
Morsy, W. S. (2009). Environmental management to groundwater resources for Nile Delta region,” in PhD thesis, Egypt: Faculty of Engineering, Cairo University.
Mishra, B. K., Kumar, P., Saraswat, C., Chakraborty, S., and Gautam, A. (2021). Water security in a changing environment: concept, challenges and solutions. Water 13, 490. doi:10.3390/w13040490
Mohamed, A. (2020). Gravity applications to groundwater storage variations of the Nile Delta Aquifer. J. Appl. Geophys 182, 104177. doi:10.1016/j.jappgeo.2020.104177
Negm, L. M., Youssef, M. A., and Jaynes, D. B. (2017). Evaluation of DRAINMOD-DSSAT simulated effects of controlled drainage on crop yield, water balance, and water quality for a corn-soybean cropping system in central Iowa. Agric. Water Manag. 187, 57–68. doi:10.1016/j.agwat.2017.03.010
Ritzema, H. P., Satyanarayana, T. V., Raman, S., and Boonstra, J. (2008). Subsurface drainage to combat waterlogging and salinity in irrigated lands in India: lessons learned in farmers’ fields. Agric. Water Manag. 95, 179–189. doi:10.1016/j.agwat.2007.09.012
Sallam, G., and Abd-Elaty, I. (2012). “Subsurface drainage impacts on the environment in Egypt,” in The 11th international drainage workshop on agricultural drainage needs and future priorities. Cairo, Egypt.
Sallam, G. A. H. (2019). “The assessment of Egypt’s subsurface drainage system,” in Unconventional water resources and agriculture in Egypt. Editor A. M. Negm (Cham: Springer International Publishing), 27–58.
Savenije, H. H. G. (2010). HESS Opinions Topography driven conceptual modelling (FLEX-Topo). Hydrol. Earth Syst. Sci. 14, 2681–2692. doi:10.5194/hess-14-2681-2010
Shahid, S. A., Zaman, M., and Heng, L. (2018). “Introduction to soil salinity, sodicity andDiagnostics techniques,” in Guideline for salinity assessment, mitigation and AdaptationUsing nuclear and related techniques (Cham: Springer), 1–42.
Taylor, R. G., Scanlon, B., Döll, P., Rodell, M., van Beek, R., Wada, Y., et al. (2013). Ground water and climate change. Nat. Clim. Change 3, 322–329. doi:10.1038/nclimate1744
Tewari, V. P., Arrawatia, M. L., and Kumar, V. S. K. (1997). The problem of soil salinity andWaterlogging in indira gandhi canal area of Rajasthan state. Ann. Biol. 13 (1), 7–13.
Toro, M., Ptak, T., Massmann, G., Sültenfuß, J., and Janssen, M., (2022). “Groundwater flow patterns in a coastal fen exposed to drainage, rewetting and interaction with the Baltic Sea”, J. Hydrology, 615, ISSN 0022–1694. doi:10.1016/j.jhydrol.2022.128726
Van der Molen, W. H., and Wesseling, J. (1991). A solution in closed form and a series solution to replace the tables for the thickness of the equivalent layer in Hooghoudt's drain spacing formula. Agric. Water Manag. 19, 1–16. doi:10.1016/0378-3774(91)90058-Q
Youssef, M. A., Abdelbaki, A. M., Negm, L. M., Skaggs, R. W., Thorp, K. R., and Jaynes, D. B. (2018). DRAINMOD-simulated performance of controlled drainage across the U.S. Midwest. Agric. Water Manag. 197, 54–66. doi:10.1016/j.agwat.2017.11.012
Keywords: drainage system, climate change, layout, groundwater, drainage rates
Citation: Abd-Elaziz W, Elsaiad A, Sallam GAH, Kuriqi A, Ahmed AA and Abd-Elaty I (2025) Performance of subsurface drainage systems considering groundwater flow directions and network alignment. Front. Earth Sci. 12:1299495. doi: 10.3389/feart.2024.1299495
Received: 22 September 2023; Accepted: 26 November 2024;
Published: 06 January 2025.
Edited by:
Alexandre S. Gagnon, Liverpool John Moores University, United KingdomReviewed by:
Moctar Diaw, Cheikh Anta Diop University, SenegalLiang Chen, China University of Mining and Technology, China
Copyright © 2025 Abd-Elaziz, Elsaiad, Sallam, Kuriqi, Ahmed and Abd-Elaty. This is an open-access article distributed under the terms of the Creative Commons Attribution License (CC BY). The use, distribution or reproduction in other forums is permitted, provided the original author(s) and the copyright owner(s) are credited and that the original publication in this journal is cited, in accordance with accepted academic practice. No use, distribution or reproduction is permitted which does not comply with these terms.
*Correspondence: Ismail Abd-Elaty, ZW5nX2FiZGVsYXR5MjAwNkB5YWhvby5jb20=; Ashraf A. Ahmed, YXNocmFmLmFobWVkQGJydW5lbC5hYy51aw==; Alban Kuriqi, YWxiYW4ua3VyaXFpQHRlY25pY28udWxpc2JvYS5wdA==