- Carl von Ossietzky Universität Oldenburg, Institute for Chemistry and Biology of the Marine Environment, Wilhelmshaven, Germany
Coastal erosion and the need for flood protection present globally significant challenges. To address these challenges, hard coastal protection structures, such as groynes, are employed worldwide to safeguard coastal areas and regulate currents. However, their specific effects on current dynamics and sediment properties, particularly within tidal inlets, remain inadequately investigated, especially in regions like the North Sea characterized by prevalent tidal currents. This study aims to address the knowledge gap by examining the long-term impacts of coastal protection measures on sedimentology, with a focus on the environment of a tidal inlet. The Southern North Sea coast is subject to mesotidal conditions. It presents a mixed-energy coast with an erosive eastward littoral drift, providing an ideal setting for this investigation. On the island of Wangerooge, a prominent groyne extends into the Harle inlet, significantly restricting the exchange area between the North Sea and the Wadden Sea. Consequently, the changes in flow dynamics and sediment transport resulting from the construction significantly affect sediment distribution and morphology within the inlet. Sedimentological analysis was employed to characterize surface sediment properties and statistical analysis identified seven distinct facies associated with three realms, which were shaped by the tidal currents affected by the groyne to a distinct pattern. Additionally, the integration of multibeam data from existing literature facilitated the creation of a comprehensive facies map. These findings suggest alterations in the morphology of the inlet. By comparing the results with an unaffected inlet, the Otzumer Balje, this study provides valuable insights into the complex interplay between coastal protection infrastructure and coastal sedimentology within a high-dynamic tidal inlet system.
1 Introduction
Coastal erosion and flood protection represent globally acknowledged challenges, particularly amid the escalating risks of climate change and rising sea levels (Linham and Nicholls, 2010; Masselink and Russell, 2013, Vousdoukas et al., 2018). Small islands and flat coastal areas exhibit heightened vulnerability due to their geographical characteristics, presenting challenges in terms of limited adaptation options. Additionally, regions with substantial natural and cultural heritage necessitate protection against these challenges, given that relocation is frequently impractical, and the very existence of these areas is imperiled. A spectrum of coastal protection have been developed ranging from hard structures like dikes, seawalls, breakwaters, and groynes to softer, more natural approaches such as beach nourishment, reef restoration, and the restoration of seagrass meadows and salt marshes. While natural methods tend to offer positive ecological impacts, the utilization of hard coastal protection measures may be indispensable for ensuring effective and resilient protection (De Ruig, 1998; Narayan et al., 2016; Morris et al., 2018). Typically, the implementation of hard coastal protection structures is considered in areas where there is a high infrastructure and asset risk or where relocation and adaptation options are unfeasible. Moreover, these structures offer distinct advantages in regions with high protection value, such as densely populated cities or heavily utilized waterways. The need for immediate and reliable protection is crucial, and hard structures are capable of providing such results (Schoonees et al., 2019). It is often intended for hard structures to undergo periodic renewal or modification during their protective period.
The North Sea stands out as an area where coastal protection measures are prevalent. The concept of “building with nature” has emerged as a scientific approach where conventional coastal protection methods are adapted and substituted with innovative strategies that encompass greater socio-economic functions (Van Slobbe et al., 2013). However, most areas along the North Sea coast rely on conventional structures like groynes, dykes, and seawalls for protection (Kunz, 1997). Characterized by a mesotidally influenced coastline with a flat mainland, fronted by islands, the North Sea coast features the East Frisian Islands, a chain of barrier islands located in the southern German North Sea. These islands formed during the Holocene transgression, driven by the rapid rise in global sea level resulting from the melting of global ice sheet. The transgression causes the coastline to shift landward, forming predominantly sandy islands. The process involved the gradual rise in sea level, reaching river valleys and transform the previously elevated Pleistocene sand into elongated spit bars through wave action. Over time, these spit bars migrated inland, developing into coastal dunes. As the tidal amplitude transitioned from microtidal to mesotidal, the spit bars underwent a transformative process, evolved into elongated detached barrier islands (Figure 1). The continuous widening of the tidal inlets, influenced by the intricate interplay of semidiurnal tides, wave action, and currents, has engendered a persistent littoral drift and consequential erosion force in the west-to-east direction (Streif, 1989; Niemeyer, 1995). Presently, the East Frisian Islands are subject to the enduring effects of semidiurnal tides, dynamic wave action, and strong currents within the tidal inlets, leading to an ongoing littoral drift and a prevailing erosion force in the west-to-east direction. This drift instigates a migration of the inlet channels, with erosion predominantly manifesting on the western side of the islands, while sediment deposition occurs on the eastern side. In the early 20th century, groynes were constructed to counteract this shift (Witte, 1970; Flemming and Davies, 1994; Niemeyer et al., 1996; Kunz, 1997). The general significance of barrier islands as a protective barrier for the mainland and wetlands, mitigating erosion and storm impacts, has been emphasized by Fritz et al. (2007) and Penland et al. (1988).
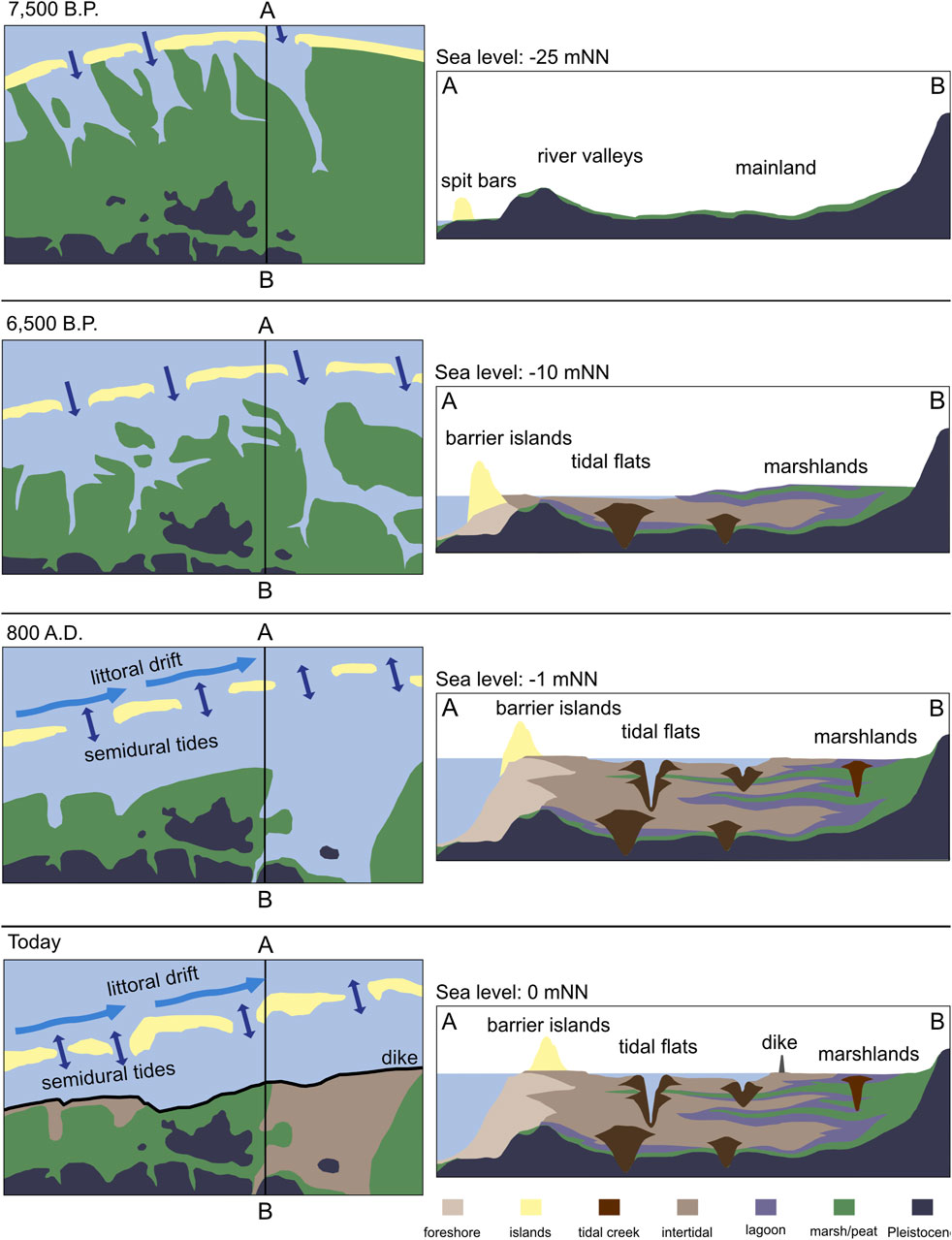
Figure 1. Schematic development of the coastline along the East Frisian Islands, modified after Flemming and Davies (1994), begins around 7,500 B.P. with a sea level of −25 mNN. This area is characterized by spit banks and primarily consists of marsh/peat sediments and Pleistocene sands. A cross-section along line AB illustrates the evolution of the subsurface in the region. As the sea level rose, the spit banks transformed into islands around 6,500 B.P. and migrated inland. The higher sea level resulted in tidal currents, giving rise to mudflats and marshes in the backshore area. By 800 AD, the development of semidiurnal tides and littoral drift was complete. With the implementation of today’s coastal protection measures, such as dikes, the landward migration of the islands was halted, and the littoral drift led to an eastward transport.
Initially, beach groynes were constructed as a measure to safeguard the northern beaches of the East Frisian Islands. However, due to their limited effectiveness in preventing sand erosion and detrimental generating of scours, the beach groynes were transformed into stream groynes through the addition of underwater extensions. This modification successfully prevented further erosion. Nevertheless, during storm events, the northern beaches are subject to significant damage, resulting in the removal of sand. To mitigate this, artificial beach nourishment is employed to replenish the eroded material and maintain existing structures. The construction of stream groynes serves to prevent further migration and facilitates the stabilization of the islands. Overall, the groyne protection measures on the East Frisian Islands aims to safeguard the island settlements, stabilize waterways and inlets, and provide protection against storm flood events (Eitner, 1996; Kunz, 1997).
The majority of the East Frisian Islands are protected by groynes to defend their northern beaches. However, specific inlets exhibited a significant tendency to migrate eastward and endanger the settlements. In part, this migration is so extensive that settlements that were located at the former eastern side of the island are nowadays located at its western side. Thus, further migration of the island threatens the very existence of the settlements (Lüders, 1952; Homeier, 1973; Fitzgerald and Penland, 1987). To address this, modifications were made to the western inlets of the islands Borkum, Norderney, Baltrum, and Wangerooge using groynes. Notably, the tidal inlet between Spiekeroog and Wangerooge (Harle inlet) is heavily influenced by a stream groyne. Conversely, the tidal inlet between Langeoog and Spiekeroog (Otzumer Balje) remains unprotected, with a groyne field developed solely along the northern beach of Spiekeroog.
The Spiekeroog Coastal Observatory (SCO; Zielinski et al., 2022) and its integrated Time Series Station (TSS; Badewien et al., 2009; Reuter et al., 2009) provides a robust scientific infrastructure for conducting various investigations in the Otzumer Balje. Through the utilization of this infrastructure, Son et al. (2011) discovered evidence of sediment recirculation within the ebb tidal delta. The transport of sediments in this area is primarily driven by the combined forces of tidal currents and wave action. Noormets et al. (2006) conducted research on local scouring within the tidal inlet, focusing on hydrodynamic flow changes due to the presence of the TSS pile and the interaction of currents with existing bedforms such as megaripples and compound dunes. Their study explored seasonal variations in ripple dimensions and the influence of neap-spring tidal cycles. Additionally, numerous numerical models have been employed to analyze the morphological, sedimentological, and hydrodynamic conditions of the Otzumer Balje (Wang et al., 1995; Bartholomä et al., 2009; Lettmann et al., 2009; Wang et al., 2012; Wang et al., 2014).
The aforementioned investigations along the Otzumer Balje inlet describe a high dynamic system with crucial interactions between tidal currents, waves, morphology and sediments. Interrupting the natural system in the Harle inlet by the construction of groynes leads on the one hand to the required termination of the natural shifting and migration of the inlet channels. On the other hand, the natural equilibrium is disrupted and depending on the varying condition the effects are various. To gain a better understanding of the interactions between environment and construction the Harle inlet between Spiekeroog and Wangerooge is investigated.
Mascioli et al. (2022) present sedimentological maps and hydroacoustic data obtained from the Harle inlet. The maps delineate four distinct sediment types within the inlet and describe its primary bedforms. The authors emphasize the erosive forces caused by the interaction between an anthropogenic element, a groyne, and tidal currents. However, their study lacks detailed sedimentological surface facies with component analysis, as well as information on alteration and deposition processes. The primary aim of our study is to investigate the impact of the groyne on sedimentology to comprehend the interactions of currents and transport within the Harle. Additionally, the study aims to interpret morphological alterations through notable features observed within the inlet, as reflected in maps and data.
2 Materials and methods
2.1 Study area
The barrier island system along the southern North Sea serves as a crucial protective barrier for the Wadden Sea, spanning the Dutch, German, and southern Danish coasts. This study focusses on the northwest coast of the German Wadden Sea, specifically the vicinity of the East Frisian Island of Spiekeroog within this barrier island system. The presence of tidal inlet systems between the islands facilitates the connection and exchange of water between the tidal flats and the open sea. In the case of Spiekeroog, the island is confined by two tidal inlet systems, namely, “Otzumer Balje” to the west, separating the islands Langeoog and Spiekeroog, and “Harle” to the east, separating Spiekeroog from Wangerooge (Figure 2).
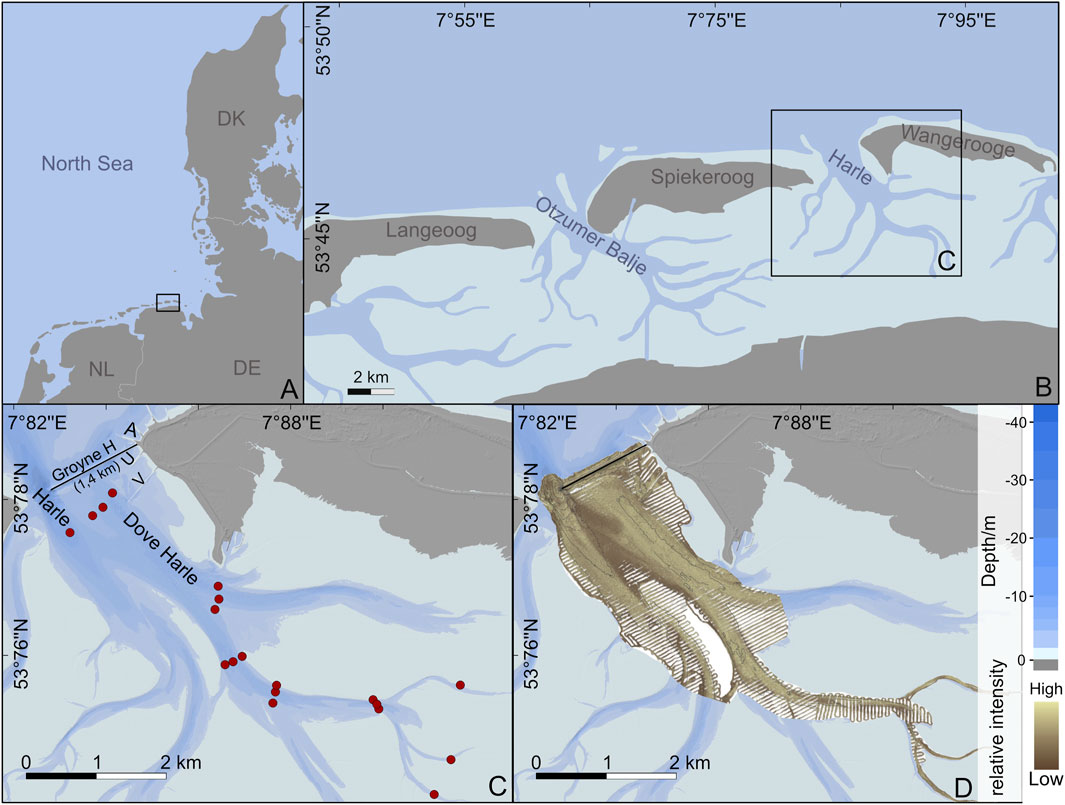
Figure 2. Overview map in (A) (DK: Denmark; DE: Germany; NL: Netherlands) of the working area along the German North Sea coast (WSV, 2021). (B) shows the islands Langeoog, Spiekeroog and Wangerooge and the tidal inlets “Otzumer Balje” and “Harle.” In (C) a detailed map of the sampling stations (red dots) along the Harle is shown with the marked groynes A, H, U, and V. The modified multibeam echo sounder backscatter data from Masciloi et al., 2022 are shown in (D). Dark colors indicate low backscatter intensity and light colors indicates high intensity.
2.1.1 Sedimentological situation
On the seaward side of the tidal inlets, sediment accumulation has resulted in the formation of ebb-tidal deltas northeast of the island (Hayes, 1980). This process is driven by a strong littoral drift, tidal currents, and waves action. Sediment bypassing along the ebb-tidal deltas forms swash-bar migration around the outer edge. Additionally, the hypothesis proposed by Burchard et al. (2008) suggests that density gradients contribute to the accumulation of Suspended Particular Matter (SPM) in the Wadden Sea. The prevailing understanding, as postulated by FitzGerald (1988) and FitzGerald et al. (1984, 2000), identifies longshore sediment movement as the primary driver of easterly sediment transport. This sediment transport exerts a considerable influence on the East Frisian Islands, prompting an eastward shift (Kunz, 1997).
The island of Wangerooge has historically borne the brunt of intense storm surges during the 19th century, resulting in severe consequences. The impact of these storm surges included the destruction of villages on Wangerooge and eroded the dunes along the western coast. Subsequent to dune breaching, a sand beach formed above the high-water level in the western part of Wangerooge. Notably, storm surge events caused the dune edge in the western part of Wangerooge to shift approximately 1,370 m eastward. Over the period from 1,650 to 2004, the west side of Wangerooge shifted eastward by 2010 m, while the east side extended by 3,030 m. A parallel eastward sediment transport from the neighbouring island Spiekeroog led to a reduction of the width of the Harle from 5.5 km to about 2 km. Simultaneously, the orientation of the Harle estuary shifted from north to northeast, forming the “Dove Harle.” The formation of the Dove Harle can be attributed to the connection of a tidal channel and a scour caused by the ebb current in the area of Groyne A (Figure 2). With the formation of the Dove Harle, the western beach of Wangerooge underwent erosion and shifted to the southwest (Lüders, 1952; Homeier, 1973; Ladage and Stephan, 2004).
To counteract further erosion on the west side of Wangerooge, the beach groyne, Groyne H, was constructed. Subsequent to its implementation, a limited redeposition of sands stabilized in the southwest region, although no re-sedimentation occurred in the west. The extension of Groyne H aimed to prevent the formation of Dove Harle and mitigate siltation, achieving partial success. Furthermore, the groyne contributed to stabilizing the course of the Harle estuary. Between 1950 and 2002, a bar formed, separating the channels of the Harle and the Dove Harle. This bar, aligned orthogonally to the Groyne H and parallel to the channel, is situated the tip of the Groyne H (Lüders, 1952; Homeier, 1973; Ladage and Stephan, 2004).
2.1.2 Hydrodynamic situation
The hydrodynamic conditions along the Harle inlet exhibit characteristics that are instrumental to the overall understanding of the study area. The tidal regime is predominantly semidiurnal, marked by a mean range of 2.9 m. Annual wave heights, as reported by Ladage et al. (2007), vary significantly within the range 0.7–1.0 m. This coastal area, as classified by Hayes (1979), can be characterized as a mesotidal, mixed-energy coast with tidal influence (Ladage et al., 2007). In this region, the ebb current emerges as the primary tidal current around the East Frisian Islands. The flow passes through the inlet channels, showcasing a strong north-westerly sediment transport that notably exceeds the strength of the south-easterly sediment transport during the flood flow. The flood current, as observed by Niemeyer (1990), endures a comparatively shorter duration, creating an asymmetry in the tidal patterns. The temporal dynamics of tidal currents, as highlighted by Stanev et al. (2007), indicate an early occurrence of the flood current, while the ebb current commences shortly before slack tide. This temporal asymmetry contributes to the overall tidal behaviour. Generally, the maximum tidal current is more than 1 ms−1, ushering dense North Sea water with higher salinity into the comparatively lighter brackish Wadden Sea water. A noteworthy phenomenon arises in the Harle due to the constrained discharge of the stream groyne on the downstream side during low tide of the Groyne H. This restriction gives rise to a counteracting dynamic eddy, resulting in a low-velocity zone at the centre of the eddy. The clockwise rotation of the eddy with a speed of 0.2 ms−1 is confined by the Groyne H and Groyne V (Albinus, 2021). This intricate interplay of tidal currents, sediment transport, and eddy formation plays a crucial role in shaping the hydrodynamic conditions of the Harle inlet, necessitating careful consideration in the broader context of the study.
2.2 Methods
Following the storm flood season in March 2022, sediment samples were collected in the Harle inlet with a 1 m2 Van-Veen grab sampler ar several stations along the tidal channel (Figure 2). The same sampling stations were approached again after the fair-weather season in September 2022. The deployment of the grab sampler from RV Senckenberg targeted subtidal stations, while RV Otzum was employed for shallower stations in both intertidal and subtidal areas during high water tide.
For comprehensive analysis bulk samples (250 mL) extracted from the grab sampler were used for grain size analysis after Udden and Wenthwoth scale, and semi-quantitative component analysis. Grain size analysis were involved wet sieving (>2000 μm; 2000–1,000 μm; 1,000–200 μm; 200–63 µm and <63 µm) followed by a drying process lasting 72 h in a drying oven at constant 38 °C. Subsequently, the weight percentages (wt%) of the five grain size fractions were determined gravimetrically. To conduct a semi-quantitative component analysis of the mass and types of siliciclastic, here quartz minerals and flint, as well as organic components the fractions >63 µm were analysed with a binocular of a scatter sample. These components were classified based on their abundances: present (1%–2%), rare (2%–5%), abundant (5%–10%), very abundant (10%–24%) and dominant (>24%). The combination of grain size distribution and component analysis, is utilized to determine the sedimentological facies (Reading, 1996; Miall, 2022). The facies along the Harle inlet are classified and categorized by statistical analysis into facies groups. The multibeam data presented by Mascioli et al. (2022), depict the seafloor characteristics within the study area. Bathymetric information facilitated the identification of morphological features such as ripples and slope angles, while the backscatter intensity could give more insights into sediment characteristics and distribution.
Hence, the correlation of that backscatter data with the sedimentological results of our study facilitated the precise mapping of facies on to distinct backscatter intensity zones, culminating in the development of a detailed facies map of the sample sites. This systematic approach ensures the most comprehensive coverage in characterizing the sedimentology of the Harle inlet and extended the work of Mascioli et al. (2022) by the detailed facies map to reveal the impact of the longstanding groyne.
To thoroughly investigate the long-term impacts of coastal protection measures on the Harle inlet, a comparative analysis is conducted with the Otzumer Balje inlet. The Otzumer Balje, situated between the islands of Langeoog and Spiekeroog, experiences minimal impact from coastal protection measures and is well described in the literature. Consequently, any significant deviations, particularly in morphology and sediment distribution, observed in the Harle may indicate the influence of groynes.
3 Results
The sedimentological characteristics of the sample locations along the inlet system of the Harle have been analyzed. Overall, seven facies groups have been identified: clayey sands, organic-rich clayey sands, very fine sands, bioclastic organic-rich fine sands, bioclastic fine sands, bioclastic middle sands, and shill sands (Figure 3). In combination with the backscatter intensity of the multibeam data from Mascioli et al. (2022), the facies of the sample locations have been expanded due to their associated backscatter intensity area and led to a facies map of the Harle (Figure 4).
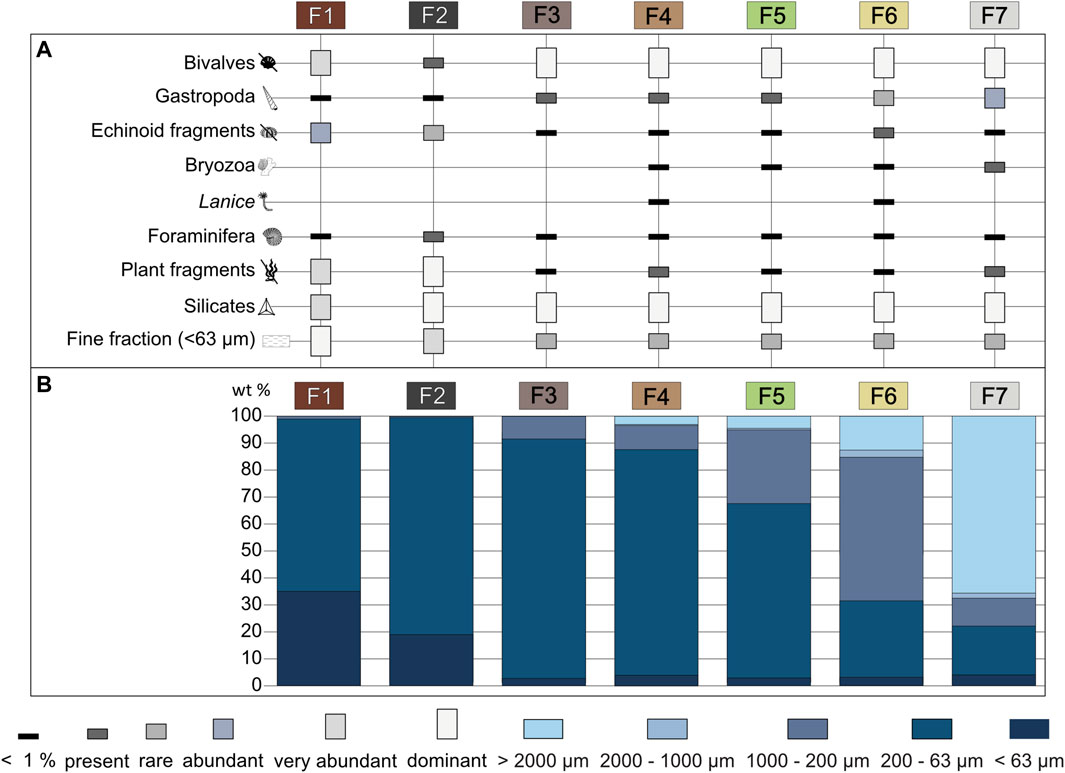
Figure 3. In (A) the relative abundance of the major components of the seven identified facies is shown. Silicates contain quartz minerals and flint stone. Abundance ranges from dominant (>24%) to very abundant (10%–24%), to abundant (5%–10%), to rare (2%–5%) to present (1%–2%), to under represent with <1%. The combination of the different sediment formers defines the facies. In (B) the mean grain size distribution is shown. Lighter blueish colors indicate a coarser material. Thus, the grain size increases from facies F1 to F7.
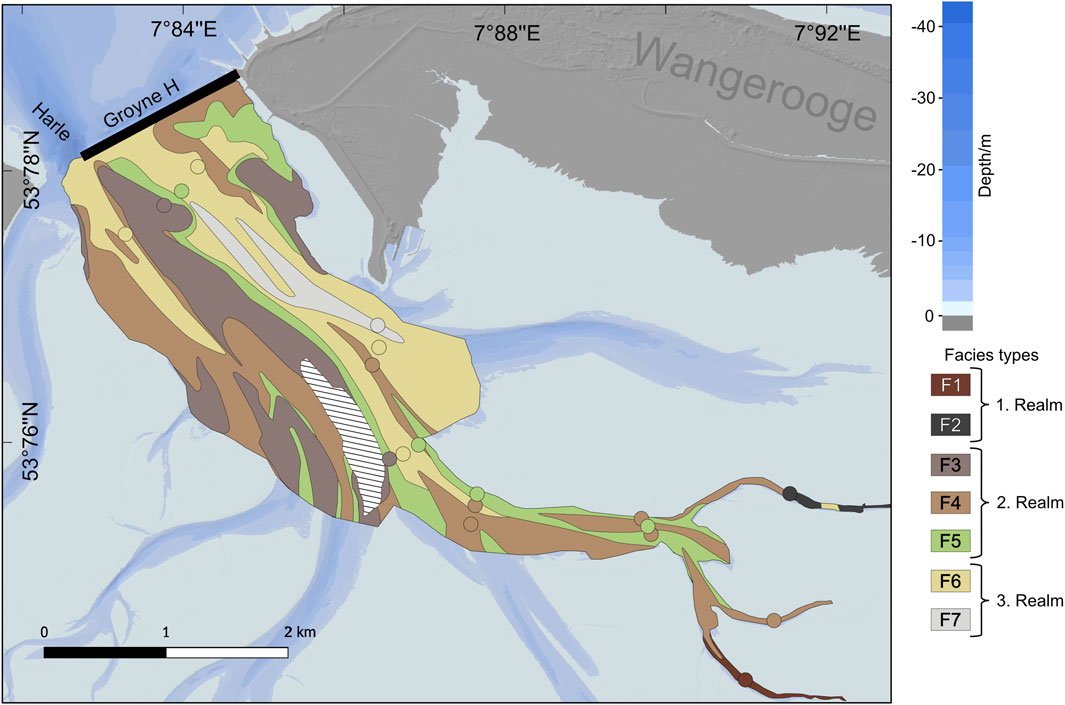
Figure 4. Facies map of the working area. Dark brownish colors represent the first realm of facies F1 and F2 in the channels. Brownish and greenish colors in the main channels and on the flanks represent facies F3, F4, and F5 of the second realm. Yellowish and grayish colors in the main channels represent the third realm of facies F6 and F7. The colored points show the sample station and the associated facies.
3.1 Facies F1: clayey sands
Facies F1, characterized by clayey sands, exhibits very fine sands with a fine fraction (<63 µm) content of 35 wt%. The grain size distribution displays a well sorted sand with the median (D50) of 79 µm. Within the sediment grains (>63 µm), a diverse composition includes silicates, bivalve shell debris and plant detritus. Minor occurrences of echinoid spines are observed, while gastropod remains and the benthic foraminifera Haynesina sp., Elphididae sp., and Ammonia sp. are comparatively less present.
The spatial distribution of clayey sands occur in the southernmost gully, situated closest to the mainland and farthest from the inlet. Utilizing multibeam backscatter data, the precise area of the facies has been identified along the end of the southernmost gully at water depth between 1 and 2 m.
3.2 Facies F2: organic-rich clayey sands
Dominated by a higher amount of plant detritus, the organic-rich clayey sands facies F2 consists of very fine sand with a fine fraction of 19 wt%, in contrast to F1. The D50 of the F2 facies is 83 µm and the sediment is well sorted. This facies is predominantly composed of plant fragments and silicates. F2 also contains of present echinoid fragments, mainly echinoid spines, the foraminifera Haynesina sp., Ammonia sp, and Elphididae sp, as well as bivalve fragments.
Located in the northern gully of the Harle inlet, close to Wangerooge, the facies F2 is in the multibeam data depicted as patches along the end of the norther gully. Water depths associated with this facies range between 1 and 2 m.
3.3 Facies F3: very fine sands
Comprising well sorted very fine sands with a D50 of 91 µm and a fine fraction of 3 wt%, facies F3 primarily consists of silicates and bivalve fragments. Gastropoda, plant fragments, echinoid fragments and the foraminifera Ammonia sp., Haynesina sp., and Elphidiae sp. are also present.
Facies F3 is located along the shallow flanks of the channels, with water depths ranging between 2 and 6 m. Additionally, the very fine sands of this facies occur also in the middle of the inlet area, approximately 730 m south of Groyne H, forming the sand bar between the Harle and the Dove Harle at a water depth of around 5 m.
3.4 Facies F4: bioclastic organic-rich fine sands
Characterized by a composition mainly of silicates and bivalve shell debris, the F4 bioclastic organic-rich fine sands exhibit a D50 of 96 µm and a minor fine fraction of 4 wt%. The grain size distribution varies from well to poor sorted, and additional sediment forming components include gastropods, plant fragments, with minor amounts of bryozoan, Lanice sp., tube fragments, echinoid fragments, and foraminifera.
Covering the flanks of the main channel and the transition zone between the Wadden area and the inlet system, facies F4 is present at water depths ranging between 2 and 7 m.
3.5 Facies F5: bioclastic sands
Distinguished by fine grained, poor sorted sands, facies F5 is composed of a coarser grain size with a D50 of 128 µm. Made primarily of silicates and bivalve shell debris, facies F5 contains a lesser amount of fine fraction, 3 wt%. Other components are gastropods, while echinoid fragments, foraminifera, and bryozoan are underrepresented, and plant fragments are absent.
Similar to facies F4, the coarser bioclastic sands of facies F5 occur along the main channels and in the transition zone between the intertidal zone and the subtidal zone, with water depths ranging between 2 and 8 m.
3.6 Facies F6: medium bioclastic sands
Facies F6 is composed of bivalve shell debris and silicate grains with present echinoid fragments and less present bryozoans, foraminifera, Lanice tubes, and plant fragments. The medium grained sands are poorly sorted with a D50 of 281 µm. The fine fraction content is 3 wt%, and gastropods are present in equal amounts.
Facies F6 is notably found in the main channels of the Harle and Dove Harle, with water depths exceeding 5 m.
3.7 Facies F7: shill sands
Comprised mainly of silicate grains and bivalve fragment shill, facies F7 is made of a poorly sorted coarse grained sand with a D50 of 820 µm. Gastropods are the abundant sediment former, and fragments of bryozoan occur. Less present are plant fragments, echinoid fragments and foraminifera. A distinctive feature is the preservation of bivalve shells, covered with encrusting bryozoans, with their calcific shells being micritic.
Distributed along the inner channel of the Dove Harle, the facies F7 is exclusive to this area, with water depths ranging between 5 and 10 m.
The sediment type changes along the morphology of the inlet system. In the shallow gullies of the Wadden shoals between the islands and the mainland, is mud, while at higher water depths on the flanks and surfaces of the narrower channels, are finer sands. As the water depths increase closer to the inlet and the exchange area between the North Sea and the Wadden Sea, the deep main channels are composed of medium to coarse-grained sands. The main channels are separated by a shallow sandbar, which interrupts the coarse facies with finer facies.
4 Discussion
The inlet system of the Harle provides a distinctive case study for investigating the impact of longstanding hard coastal protection measure in tidal environments. The implementation of such measures has resulted in significant alterations in flow dynamics and a constriction in the exchange between the North Sea and the adjacent Wadden Sea. Consequently, discernible perturbations can be observed in the inherent morphology of the inlet and the sedimentological facies characteristics.
4.1 Facies distribution
In a comprehensive classification, the sedimentary facies within the Harle inlet can be grouped into three distinct realms, encompassing a total of seven facies. The first realm, the Wadden realm, is represented by facies F1 and F2, predominantly occurring within the gullies of the intertidal shoals, exhibiting a high content of fine sediment and organic detritus. The second realm comprises facies F3, F4, and F5, constituting the channel beds and flanks. Within these subtidal areas, the sediment is predominantly fine-grained, with a minor portion of fine fraction. Silicate grains and bivalve shell debris are the primary constituents of these facies. A sandbar present towards the southern region of Groyne H, composed of facies F3, F4, and F5, shares sedimentological characteristics with the channel beds and flanks. The third realm is the main channel realm, characterized by facies F6 and F7. These facies exhibit a sediment composition of medium to coarse-grained sands, containing significant amounts of silicate grains and bivalve shell debris. This realm is distinguished by the largest water depths in the main channels, and the flow energy during tidal exchanges is most pronounced. Surface sediment structures are constituted of sand rippels and sand waves (Mascioli et al., 2022).
Mascioli et al. (2022) proposed a similar classification for the Harle, identifying an additional realm characterized by Holocene peat or clay outcrops. This realm consists of hard substrate from the Holocene, surrounded by sands, and is situated along the slope of the main Harle channel. However, due to the limited spatial extent of this realm and the lack of ground truthing, its presence is solely based on the interpretation of hydroacoustic data. Observations by Streif (2004) indicate the occurrence of a peat layer along the slopes of the tidal inlets representing the termination of Pleistocene morainic and glaciofluvial deposits overlain by Holocene marine sediments. This peat layer suggests the erosion forces exerted by the current flow and provides insights into the maximum depth of channel deepening and the reworking of underlying coarse sediments in the inlets.
Conspicuous features observed in Facies F7 include bivalve shells encrusted by bryozoans (Figure 5.). While bryozoans are present in Facies F4, F5, and F6, their abundance is less than 1%, indicating their underrepresentation. The primary occurrence of bryozoans is found in Facies F7 along the channel of the Dove Harle. Bryozoans, known to require hard substrates for larval settlement only on solid surfaces (Ward and Thorpe, 1989; Kuklinski and Barnes, 2005). In unconsolidated sediment, sessile organisms can utilize mollusk shells as hard substrates. Encrusting and boring bryozoans are particularly prevalent on disarticulated bivalve shells in diverse marine coastal environments (McKinney, 1996; Kuklinski et al., 2005; de Blauwe, 2006; 2020; Brett et al., 2011; Denisenko et al., 2017; Almeida A. C. S. et al., 2018; Almeida A. et al., 2018). The most stable position for shells in high-velocity regimes is with the convex side facing upwards (Allen, 1984). In intertidal regimes, encrusting bryozoans, as described by Almeida et al. (2015) and de Blauwe (2006), generally grow on disarticulated bivalve shells on their convex side. Amini et al. (2004) observed that areas with high sedimentation rates tend to have fewer bryozoans. Disturbances such as substrate movement and bioturbation can also decrease the bryozoan abundance (Kelly and Horowitz, 1987; Smith, 1995).
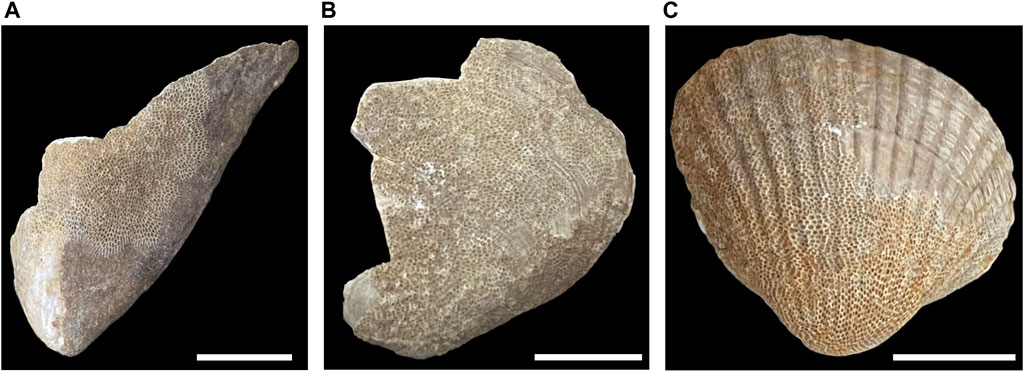
Figure 5. Disarticulated bivalve shells of Mythilidae (A), Mya (B) and Cardiidae (C) and with overgrown bryozoans. Scale bar shows 1 cm.
In Facies F7, bryozoans are predominantly found on the convex side of bivalve shells, suggesting a stable position. The presence of encrusting bryozoans implies a low sedimentation rate in this area, while the orientation indicates a stable substrate movement with low grain movement. Lüders (1952) described a sediment deposition process of an infill in the Dove Harle. Investigations by Homeier (1973) and Ladage and Stephan (2004) indicated a termination of the deposition, discussing the non-attainment of dynamic equilibrium. The occurrence of bryozoans on the bivalve shells also suggests restricted sedimentation within the Dove Harle. Currently, it is assumed that 66% of the ebb current flows through the Dove Harle (Ladage and Stephan, 2004). This indicate changes in the hydrographic conditions, which caused the termination of deposition in the Dove Harle channel.
4.2 Morphological structures
Groynes, in general, exert significant influence on various environmental factors, including changes in bathymetry through erosion and the formation of scours, alteration of flow patterns, velocity, and turbulence sediment transport, deposition and erosion processes, as well as impacting bed shear stress (Walker, 1991; Kristensen et al., 2016; Wu and Qin, 2020; Zhou et al., 2021). Ladage and Stephan (2004) documented a bisection of the main channel, resulting in the establishment of a dual channel system since 1998. The modification appears stable, accompanied by the development of a sand barrier between the main channels of the Harle and Dove Harle.
Scouring and sediment deposition are the primary effects of groynes on sediment dynamics. The sedimentation rate is influenced by the length, angle, and crest elevation of a groyne, lower crest heights resulting in higher erosion forces and a negative sedimentation rate (Henning and Hentschel, 2013). Several investigations have been conducted to examine altered flow characteristics along groynes and groyne fields (Sukhodolov et al., 2004; Henning and Hentschel, 2013; Biria et al., 2015; Higham et al., 2017). Overall, flow velocity is increased at the tip of the groyne. The specific characteristics of the groyne, whether emerged or submerged, lead to variations in flow patterns and resulting sedimentation patterns. On the downstream side of an emerged groyne, flow velocity decreases, resulting in the development of a mixed shear layer along the streamline and the formation of a recirculation eddy near the groyne. Depending on the topography, the number of recirculation eddys differs, with a single eddy occurring in deep flows and a counteracting second recirculation eddy occurring in shallow flows (Armaly et al., 1983; Talstra et al., 2005). Based on sediment composition, larger grains settle along the mixed shear layer due to decreased flow energy, while finer sediment is transported by the eddy into the groyne field and subsequently settles. Very fine sediments tend to be transported to the attached corner of the groyne. In the case of submerged groynes, a vertical eddy forms at the leeside, leading to erosion on the downstream side. Lower flow velocities within the groyne field result in sediment deposition in the center of the field (Carling et al., 1994; Henning and Hentschel, 2013).
In the specific case of the tidal inlet of the Harle and Groyne H, the main flow direction undergoes semidiurnal changes, with the groyne being submerged during flood and partially emerged during ebb, resulting in scouring north and south of the groyne during flood conditions (Figure 6). The intensity of the scours is greater in areas where the groyne crest is lower. South of Groyne H, a sand barrier composed of Facies F3 is formed orthogonally to the groyne and parallel to the channels, permanently separating the channels of the Harle and Dove Harle. The presence of groynes can lead to the formation of sandbars, which induce changes in flow behavior. Along the mixed shear layer, where flow velocity decreases, sediment load settles. It can be assumed that the distance of 700 m is determined by the flow dynamics of vertical circulation cell which occurs during the overflow of the groyne at flood. The shape of the sand barrier follows the flow edge, which can be observed as breaking water in aerial photographs. The facies distribution indicates the presence of finer grained sediments of facies F4 and F5 in the area between Groyne H, Groyne U, and Groyne V. The recirculation eddy may transport these fine-grained sediments to the corner, where they settle and deposit.
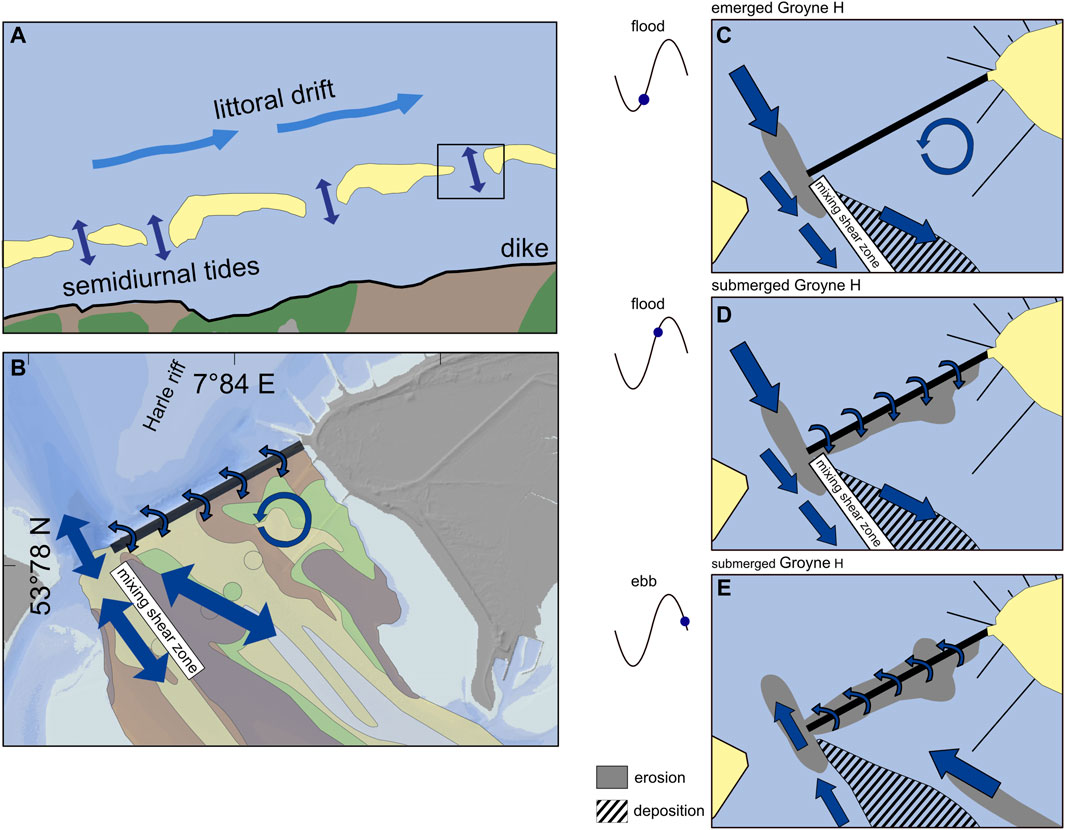
Figure 6. (A) shows an overview of the East Frisian Islands and the tidal directions and the direction of the littoral drift. (B) shows schematically the prevailing currents over the facies map, influenced by Groyne H (black line). During the onset of the flood current, the water flows into the inlet around the partially emerged Groyne H (C). An eddy forms in the downstream area. A strong current develops at the tip of the groyne, leading to erosion. Behind, a mixed shear zone develops where sediment is deposited. During high tide (D), the groyne is submerged and a vertical circulation cell develops, leading to scour erosion south of Groyne H. During the onset of the ebb current (E), the groyne is submerged and the vertical circulation cell leads to scour erosion north of the groyne. The strong current along the Dove Harle channel leads to erosion along the main channel.
Considering the tidal influences, it is reasonable to infer that sediment deposits and erosion areas are influenced by both submerged and emerged groyne characteristics of Groyne H. The scours observed during flood conditions are the outcome of the vertical eddy generated when the groyne is submerged. Conversely, the formation of the sand barrier and the presence of finer grained sands in the attached corner of the groyne field are the result of ebb conditions when the Groyne H is partially emerged, and the recirculation eddies and mixed shear layers are formed (Figure 6).
4.3 Comparison with a natural inlet
To assess the impacts caused by the longstanding coastal protection measures in the Harle, a comparison with a tidal inlet system minimally affected is necessary. The tidal inlet system between the islands Langeoog and Spiekeroog, respectively the Otzumer Balje, provides a suitable basis for such a comparison, being only protected by groynes along its northern coastline, leaving the inlet itself unaffected. Given the short distances between the Harle and Otzumer Balje, as well as the similar geographic and oceanographic conditions, a comparative analysis between these two systems can offer valuable insights into the extent of the impacts resulting from coastal protection measures. The sediment dynamics of the inlet channel in the Otzumer Balje are characterized by anticlockwise shifting due to sediment transport in the ebb delta, with recirculation of sediments occurring within the delta (Son et al., 2011; Valle-Levison et al., 2018). The tidal flats of the Otzumer Balje are dominated by a single main channel (Figure 7). In contrast to the Harle, where the main channel is divided into the Harle and the Dove Harle. The split-up of the main channel in the Harle was a semi natural process that has been influenced and restricted by human activities. However, the altered current energies and bedload capacities have led to the formation of a sandbar between the Harle and the Dove Harle. This sandbar has developed as a result of the modified flow patterns and sediment transport dynamics (Ladage and Stephan, 2004). In the Harle, the formation of the sand bar acts as a barrier, whereas a similar sand bar has not formed in the Otzumer Balje. Consequently, the current energy in the Otzumer Balje is not restricted.
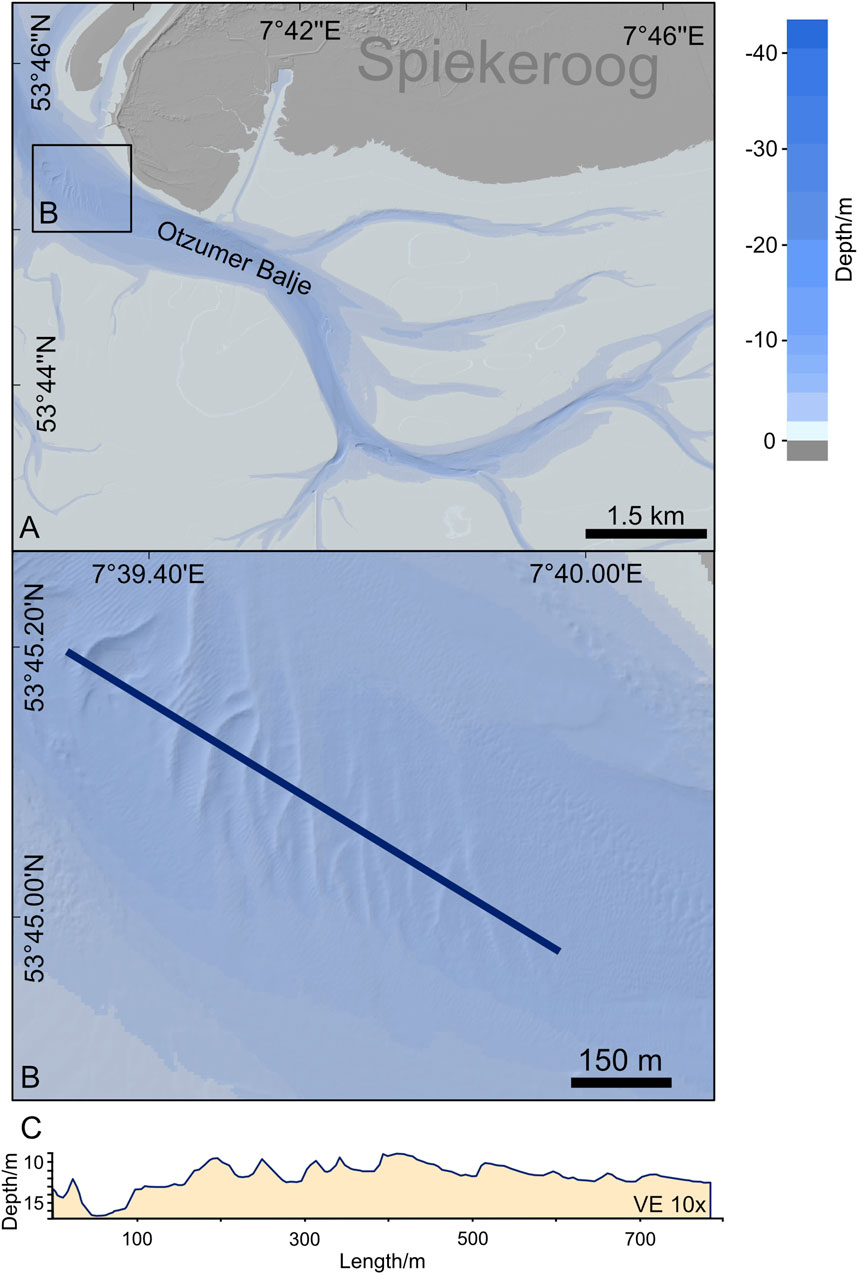
Figure 7. Overview map of the Otzumer Balje (A), with the subaqueous dune field in (B) and the marked cross section (blue line). Cross-section through the subaqueous dunes (C) with a 10x vertical exaggeration (WSV, 2021).
Digital Elevation Model data from the Waterways and Shipping Authority Germany (WSA) show the main channel of the Otzumer Balje. Here a subaquatic dune field has developed at the widest section. These compound subaquatic dune field reach a maximum height of 3.5 m and is extended to a length of 1,280 m (Figure 7). Literature states that subaquatic dunes typically form under high current velocity conditions ranging from 0.6 to 1.5 ms−1 (Reineck and Singh, 1980; Boothroyd, 1985; Ashley, 1990). Megaripples can be observed within the tidal flats along the area of the Time Series Station (Noormets et al., 2006). Subaquatic dunes are commonly found in tidal inlets, as seen in the inlet channel between islands Skallingen and Fanø, respectively, where they migrate at a rate of 0.07 m per day (Bartholomä et al., 2004). In contrast, the dominant bedforms in the Harle are ripples to megaripples, with subaquatic dunes being absent. The maximum currents in the Harle reach a speed of 1 ms−1 at the tip of the groyne, but on the downstream side, the flow velocity decreases to 0.2 ms−1 (Albinus, 2021). This results in sediment deposition along the mixed shear layer in the sand barrier. Large sand waves, rather than dunes, are observed by Mascioli et al. (2022) in the Harle, particularly on the sand barrier between the main channels. The height of these sand waves increases with distance from Groyne H. Factors such as current energy and water depth can influence the formation and height of subaquatic dunes (Allen, 1968; Flemming, 1978), and in the Otzumer Balje, the water depth along the dune catchment area ranges from 19 m to 25 m, whereas in the Harle, the water depth in the sand wave areas is <10 m.
The sediment grain size in the Otzumer Balje varies between 350 µm in the inlet channel and 88 µm in the back-barrier intertidal basin (Antia, 1994; Flemming and Nyandwi, 1994; Son et al., 2011). Notably, sediments corresponding to Facies F7 are absent in the literature about Otzumer Balje. Unlike the Harle, the Otzumer Balje does probably not exhibit shill sediments characterized by disarticulated shells overgrown by bryozoan colonies. As previously hypothesized, the channel of the Dove Harle is covered with this facies, indicating a low sediment bedload within the channel. However, despite these conditions, no distinct bedforms such as megaripples and sand waves are observed in the Dove Harle channel.
Data on the northern side of Groyne H, specifically regarding the “Harle Riff,” are currently lacking. However, it is reasonable to assume that an ebb stream recirculation eddy occurs in this area. This eddy would interact with the natural system, similar to the observed recirculation of sediments along the northern shoals in the Otzumer Balje and it may assumed that the sublittoral sediment transport is restricted to a minimum.
5 Conclusion
Overall the sedimentary facies within the Harle inlet can be classified into three realms:
- Wadden realm (Facies F1 and F2): Found in intertidal shoal gullies, this realm is characterized by fine sediment and organic detritus.
- Channel realm (Facies F3, F4, and F5): Predominantly consisting of fine-grained sediment with silicate grains and bivalve shell debris. This realm encompasses the channel beds and flanks
- Main channel realm (Facies F6 and F7): Characterized by medium to coarse grained sands with high flow energy and sand ripples. This realm is situated at the greatest depths within the main channels.
The construction of groynes in the Harle has led to various morphological changes, which are not observed in the natural neighboring tidal inlet, Otzumer Balje. Depending on the semidiurnal tides, resulting in the periodic emerge and submerge of the groyne, contribute to morphological alterations in the Harle erosion along the groyne flanks is likely induced by vertical circulation cells at high water tides. Furthermore, erosion is evident at the tip of the groyne, where increased flow exerts higher erosion forces. Conversely, specific deposition areas emerge, such as the occurring eddy during low water tides and in the mixing shear zone, where flow decreases, and sediment load is lower. These dynamics result in the separation of the two main channels, Harle and Dove Harle, in the Harle inlet. Additionally, notable differences in sediment bedforms are observed between the Harle and the natural system of the Otzumer Balje. In the natural system, bedforms are dominated by ripples and megaripples, with occasional subaquatic dunes. In contrast, the Harle exhibits only ripples and megaripples, subaquatic dunes are absent.
To enhance the comparison between the Harle and the Otzumer Balje, it is imperative to generate a facies map for the Otzumer Balje using the methodology outlined in this study. The existing literature on the Otzumer Balje referenced in this research provides a comprehensive overview of prior investigations, offering valuable insights into the general disparities between the tidal inlets. However, to enable a direct and robust comparison, the creation of a comprehensive facies map is essential. Such a map would illustrate variations in sediment distribution and surface sediment influences across a broad expanse for the Otzumer Balje, facilitating a more evident demonstration of the impacts of coastal protection measures on the Harle inlet. Unlike individual sampling points used as references, a facies map created with multibeam backscatter data provides a holistic depiction of the overall surface sediments, thereby offering a more nuanced understanding of the differences between the two inlet systems.
This study highlighted the combination of hydroacoustic backscatter data with sedimentological characteristics as grain size and component analysis to interpret morphological alterations, sedimentation and transport processes in relation to tidal currents and coastal protection structures, which could be easily applied at other study sites.
Data availability statement
The raw data supporting the conclusion of this article will be made available by the authors, without undue reservation.
Author contributions
A-LG: Data curation, Formal Analysis, Investigation, Methodology, Software, Validation, Visualization, Writing–original draft. JW: Conceptualization, Funding acquisition, Project administration, Supervision, Writing–review and editing. H-AG: Writing–review and editing. TB: Funding acquisition, Project administration, Supervision, Writing–review and editing.
Funding
The author(s) declare financial support was received for the research, authorship, and/or publication of this article. This study was conducted as part of the research project (Gute Küste Niedersachsen), which was funded by the Lower-Saxon Ministry of Research and Culture and the Volkswagen foundation (FKZ: 76251-17-5/19).
Acknowledgments
We would like to thank Michelle Albinus, Helmo Nicolai and the captains of the RV Otzum for their great work to gain the sediment sampley on the RV Otzum. And we thank the crew onboard the RV Senckenberg for supporting the deployments of the grab sampler. Acknowledgments are extended to the two reviewers whose comments on the initial submission of this paper were invaluable. Gratitude is also expressed to the Editors for their constructive feedback, which significantly contributed to the improvement of this paper.
Conflict of interest
The authors declare that the research was conducted in the absence of any commercial or financial relationships that could be construed as a potential conflict of interest.
Publisher’s note
All claims expressed in this article are solely those of the authors and do not necessarily represent those of their affiliated organizations, or those of the publisher, the editors and the reviewers. Any product that may be evaluated in this article, or claim that may be made by its manufacturer, is not guaranteed or endorsed by the publisher.
Supplementary material
The Supplementary Material for this article can be found online at: https://www.frontiersin.org/articles/10.3389/feart.2024.1292462/full#supplementary-material
References
Albinus, M. (2021). Circulation in the vicinity of a submerged stream groyne under mesotidal impact (East Frisian Islands, Southern North Sea). bachelor´s thesis. Oldenburg (Nds): Carl-von-Ossietzky-Universität Oldenburg.
Allen, J. R. L. (1968). The nature and origin of bed-form hierarchies. Sedimentology 10, 161–182. doi:10.1111/j.1365-3091.1968.tb01110.x
Allen, J. R. L. (1984). Experiments on the settling, overturning and entrainment of bivalve shells and related models. Sedimentology 31, 227–250. doi:10.1111/j.1365-3091.1984.tb01961.x
Almeida, A., Souza, F., Farias, J., Alves, O., and Vieira, L. (2018b). Bryozoa on disarticulated bivalve shells from Todos os Santos Bay, northeastern Brazil, with the description of two new species. Zootaxa 4434, 401–428. doi:10.11646/zootaxa.4434.3.1
Almeida, A. C. S., Souza, F. B. C., Sanner, J., and Vieira, L. M. (2015). Taxonomy of recent Adeonidae (Bryozoa, Cheilostomata) from Brazil, with the description of four new species. Zootaxa 4013, 348–368. doi:10.11646/zootaxa.4013.3.2
Almeida, A. C. S., Souza, F. B. C., and Vieira, L. M. (2018a). Malacostegine bryozoans (Bryozoa: cheilostomata) from Bahia State, northeast Brazil: taxonomy and non-indigenous species. Mar. Biodiv 48, 1463–1488. doi:10.1007/s12526-017-0639-x
Amini, Z. Z., Adabi, M. H., Burrett, C. F., and Quilty, P. G. (2004). Bryozoan distribution and growth form associations as a tool in environmental interpretation, Tasmania, Australia. Sediment. Geol. 167, 1–15. doi:10.1016/j.sedgeo.2004.01.010
Antia, E. E. (1994). The ebb-tidal delta model of shoreface ridge origin and evolution: appraisal and applicability along the southern North Sea barrier island coast?a discussion. Geo-Marine Lett. 14, 59–63. doi:10.1007/BF01204472
Armaly, B. F., Durst, F., Pereira, J. C. F., and Schönung, B. (1983). Experimental and theoretical investigation of backward-facing step flow. J. Fluid Mech. 127, 473. doi:10.1017/S0022112083002839
Ashley, G. M. (1990). Classification of large-scale subaqueous bedforms: a new look at an old problem-SEPM bedforms and bedding structures. SEPM JSR 60. doi:10.1306/212F9138-2B24-11D7-8648000102C1865D
Badewien, T. H., Zimmer, E., Bartholomä, A., and Reuter, R. (2009). Towards continuous long-term measurements of suspended particulate matter (SPM) in turbid coastal waters. Ocean. Dyn. 59, 227–238. doi:10.1007/s10236-009-0183-8
Bartholomä, A., Ernstsen, V. B., Flemming, B. W., and Bartholdy, J. (2004). Bedform dynamics and net sediment transport paths over a flood-ebb tidal cycle in the Grådyb channel (Denmark), determined by high-resolution multi-beam echosounding. Geografisk Tidsskrift-Danish J. Geogr. 104, 45–55. doi:10.1080/00167223.2004.10649503
Bartholomä, A., Kubicki, A., Badewien, T. H., and Flemming, B. W. (2009). Suspended sediment transport in the German Wadden Sea—seasonal variations and extreme events. Ocean. Dyn. 59, 213–225. doi:10.1007/s10236-009-0193-6
Biria, H. A., Neshaei, M. A. L., Ghabraei, A., and Mehrdad, M. A. (2015). Investigation of sediment transport pattern and beach morphology in the vicinity of submerged groyne (case study: dahane Sar Sefidrood). Front. Struct. Civ. Eng. 9, 82–90. doi:10.1007/s11709-014-0275-5
Boothroyd, J. C. (1985). “Tital inlets and tidal deltas,” in Coastal sedimentary environments. Editor R. A. Davies (New York, NY: Springer New York), 445–525. doi:10.1007/978-1-4612-5078-4
Brett, C. E., Parsons-Hubbard, K. M., Walker, S. E., Ferguson, C., Powell, E. N., Staff, G., et al. (2011). Gradients and patterns of sclerobionts on experimentally deployed bivalve shells: synopsis of bathymetric and temporal trends on a decadal time scale. Palaeogeogr. Palaeoclimatol. Palaeoecol. 312, 278–304. doi:10.1016/j.palaeo.2011.05.019
Burchard, H., Flöser, G., Staneva, J. V., Badewien, T. H., and Riethmüller, R. (2008). Impact of density gradients on net sediment transport into the Wadden Sea. J. Phys. Oceanogr. 38, 566–587. doi:10.1175/2007JPO3796.1
Carling, P. A., Orr, I. G., and Glaister, M. S. (1994). “Significance of dead zone flow structure for solute and fine particle dynamics,” in Mixing and transport in the environment. Editors K. J. Beven, P. C. Chatwin, and J. H. t. Millbank (John Wiley and Sons Ltd), 139–157.
De Blauwe, H. (2006). Bryozoa on shells from the kwintebank, southern bight of the North Sea (Belgium). Bull. Van Het K. Belg. Inst. Voor Natuurwetenschappen Biol. 76, 125–138.
De Blauwe, H. (2020). Bryozoans on disarticulated shells from pléneuf-val-andré, brittany, France. Australas. Paleontol. Memoirs 52, 57–65.
Denisenko, N. V., Thomsen, E., and Tendal, O. S. (2017). Bryozoan epifauna on brachiopods from the Faroe Islands (NE Atlantic). Frit, 96–113. doi:10.18602/fsj.v60i0.9
De Ruig, J. H. M. (1998). Seaward coastal defence: limitations and possibilities. J. Coast Conserv. 4, 71–78. doi:10.1007/BF02806492
Eitner, V. (1996). Geomorphological response of the East Frisian barrier islands to sea-level rise: an investigation of past and future evolution. Geomorphology 15, 57–65. doi:10.1016/0169-555X(95)00116-M
FitzGerald, D. M. (1988). “Shoreline erosional-depositional processes associated with tidal inlets,” in Lecture notes on coastal and estuarine studies. Editors D. G. Aubrey, and L. Weishar (Washington, D. C.: American Geophysical Union), 186–225. doi:10.1029/LN029p0186
FitzGerald, D. M., Kraus, N. C., and Hands, E. B. (2000). Natural mechanisms of sediment bypassing at tidal inlets.
Fitzgerald, D. M., and Penland, S. (1987). Backbarrier dynamics of the East Frisian islands. J. Sediment. petrology 57, 746–754. doi:10.1306/212F8BF7-2B24-11D7-8648000102C1865D
Fitzgerald, D. M., Penland, S., and Nummedal, D. (1984). Control of barrier island shape by inlet sediment bypassing: east Frisian Islands, West Germany. Mar. Geol. 60, 355–376. doi:10.1016/0025-3227(84)90157-9
Flemming, B. W. (1978). Underwater sand dunes along the southeast African continental margin — observations and implications. Mar. Geol. 26, 177–198. doi:10.1016/0025-3227(78)90059-2
Flemming, B. W., and Davies, R. A. (1994). Holocene evolution, morphodynamics and sedimentology of the Spiekeroog barrier island system (Southern North sea). Senckenberg. maritima 24, 117–155.
Flemming, B. W., and Nyandwi, N. (1994). Land reclamation as a cause of fine-grained sediment depletion in backbarrier tidal flats (Southern North Sea). Neth. J. Aquatic Ecol. 28, 299–307. doi:10.1007/BF02334198
Fritz, H. M., Blount, C., Sokoloski, R., Singleton, J., Fuggle, A., McAdoo, B. G., et al. (2007). Hurricane Katrina storm surge distribution and field observations on the Mississippi Barrier Islands. Estuar. Coast. Shelf Sci. 74, 12–20. doi:10.1016/j.ecss.2007.03.015
Hayes, M. O. (1979). Barrier island morphology as a function of tidal and wave regime. Academic Press, 28.
Hayes, M. O. (1980). General morphology and sediment patterns in tidal inlets. Sediment. Geol. 26, 139–156. doi:10.1016/0037-0738(80)90009-3
Henning, M., and Hentschel, B. (2013). Sedimentation and flow patterns induced by regular and modified groynes on the River Elbe, Germany: SEDIMENTATION AND FLOW PATTERNS INDUCED BY REGULAR AND MODIFIED GROYNES. Ecohydrol 6, 598–610. doi:10.1002/eco.1398
Higham, J. E., Brevis, W., Keylock, C. J., and Safarzadeh, A. (2017). Using modal decompositions to explain the sudden expansion of the mixing layer in the wake of a groyne in a shallow flow. Adv. Water Resour. 107, 451–459. doi:10.1016/j.advwatres.2017.05.010
Homeier, H. (1973). Die morphologische Entwicklung im Bereich der Harle und ihre Auswirkungen auf das Westende von Wangerooge. Foschungsstelle für Insel-und Küstenschutz.
Kelly, S. M., and Horowitz, A. S. (1987). “Growth-forms and paleoecology of Missisippian bryozoans: critical application of Stach´s 1936 model, eastern United States,” in Bryozoa: present and past. Editor J. R. P. Ross (Bellingham, Washington: Western Washington University), 137–144.
Kristensen, S. E., Drønen, N., Deigaard, R., and Fredsoe, J. (2016). Impact of groyne fields on the littoral drift: a hybrid morphological modelling study. Coast. Eng. 111, 13–22. doi:10.1016/j.coastaleng.2016.01.009
Kuklinski, P., and Barnes, D. (2005). Microhabitat diversity of svalbard bryozoa. J. Nat. Hist. 39, 539–554. doi:10.1080/00222930400001350
Kuklinski, P., Gulliksen, B., Lønne, O. J., and Weslawski, J. M. (2005). Composition of bryozoan assemblages related to depth in Svalbard fjords and sounds. Polar Biol. 28, 619–630. doi:10.1007/s00300-005-0726-5
Kunz, H. (1997). “Groynes on the East Frisian islands: history and experiences,” in Coastal engineering 1996 (Orlando, Florida, United States: American Society of Civil Engineers), 2128–2141. doi:10.1061/9780784402429.165
Ladage, F., and Stephan, H.-J. (2004). Morphologische Entwicklung im Seegat Harle und seinem Einzugsgebiet, 69.
Ladage, F., Stephan, H.-J., and Niemeyer, H. D. (2007). “Interactions of large-scale groyne and tidal inlet migration,” in Coastal dynamics 2005 (Barcelona, Spain: American Society of Civil Engineers), 1–14. doi:10.1061/40855(214)111
Lettmann, K. A., Wolff, J.-O., and Badewien, T. H. (2009). Modeling the impact of wind and waves on suspended particulate matter fluxes in the East Frisian Wadden Sea (southern North Sea). Ocean. Dyn. 59, 239–262. doi:10.1007/s10236-009-0194-5
Linham, M. M., and Nicholls, R. J. (2010). Technologies for climate change adaptation: coastal erosion and flooding. Denmark: UNEP Riso Centre on Energy, Climate and Sustainable Development.
Mascioli, F., Piattelli, V., Cerrone, F., Cinosi, J., Kunde, T., and Miccadei, E. (2022). Sediments and bedforms of the Harle tidal inlet (Wadden Sea, Germany). J. Maps 19, 2154175. doi:10.1080/17445647.2022.2154175
Masselink, G., and Russell, P. (2013). Impacts of climate change on coastal erosion. MCCIP Sci. Rev. 2013, 16. doi:10.14465/2013.ARC09.071-086
McKinney, F. K. (1996). Encrusting organisms on co-occurring disarticulated valves of two marine bivalves: comparison of living assemblages and skeletal residues. Paleobiology 22, 543–567. doi:10.1017/S0094837300016523
Miall, A. D. (2022). “Stratigraphy: the modern synthesis,” in Springer textbooks in Earth sciences, geography and environment (Springer), 528. doi:10.1007/978-3-030-87536-7_7
Morris, R. L., Konlechner, T. M., Ghisalberti, M., and Swearer, S. E. (2018). From grey to green: efficacy of eco-engineering solutions for nature-based coastal defence. Glob. Change Biol. 24, 1827–1842. doi:10.1111/gcb.14063
Narayan, S., Beck, M. W., Reguero, B. G., Losada, I. J., Van Wesenbeeck, B., Pontee, N., et al. (2016). The effectiveness, costs and coastal protection benefits of natural and nature-based defences. PLoS ONE 11, e0154735. doi:10.1371/journal.pone.0154735
Niemeyer, H. D. (1995). “Long-term morphodynamical development of the East Frisian islands and coast,” in Coastal engineering 1994 (Kobe, Japan: American Society of Civil Engineers), 2417–2433. doi:10.1061/9780784400890.176
Niemeyer, H. D., Eiben, H., and Rohde, H. (1996). “History and heritage of German coastal engineering,” in History and heritage of coastal engineering (Orlando, Florida: American Society of Civil Engineers), 169–213. doi:10.1061/9780784401965.005
Noormets, R., Ernstsen, V. B., Bartholomä, A., Flemming, B. W., and Hebbeln, D. (2006). Implications of bedform dimensions for the prediction of local scour in tidal inlets: a case study from the southern North Sea. Geo-Mar Lett. 26, 165–176. doi:10.1007/s00367-006-0029-z
Penland, S., Boyd, R., and Suter, J. R. (1988). Transgressive depositional systems of the Mississippi delta plain: a model for barrier shoreline and shelf sand development.
Reading, H. G. (1996). Sedimentary environments: processes, facies and stratigraphy. third edition Oxford: Blackwell Science, 615.
Reineck, H.-E., and Singh, I. B. (1980). Depositional sedimentary environments. Berlin, Heidelberg: Springer Berlin Heidelberg. doi:10.1007/978-3-642-81498-3
Reuter, R., Badewien, T. H., Bartholomä, A., Braun, A., Lübben, A., and Rullkötter, J. (2009). A hydrographic time series station in the Wadden Sea (southern North Sea). Ocean. Dyn. 59, 195–211. doi:10.1007/s10236-009-0196-3
Schoonees, T., Gijón Mancheño, A., Scheres, B., Bouma, T. J., Silva, R., Schlurmann, T., et al. (2019). Hard structures for coastal protection, towards greener designs. Estuaries Coasts 42, 1709–1729. doi:10.1007/s12237-019-00551-z
Smith, A. M. (1995). Palaeoenvironmental interpretation using bryozoans: a review. SP 83, 231–243. doi:10.1144/GSL.SP.1995.083.01.11
Son, C. S., Flemming, B. W., and Bartholomä, A. (2011). Evidence for sediment recirculation on an ebb-tidal delta of the East Frisian barrier-island system, southern North Sea. Geo-Mar Lett. 31, 87–100. doi:10.1007/s00367-010-0217-8
Stanev, E. V., Brink-Spalink, G., and Wolff, J.-O. (2007). Sediment dynamics in tidally dominated environments controlled by transport and turbulence: a case study for the East Frisian Wadden Sea. J. Geophys. Res. 112, C04018. doi:10.1029/2005JC003045
Streif, H. (1989). “Barrier islands, tidal flats, and coastal marshes resulting from a relative rise of sea level in East Frisia on the German North Sea coast,” in Coastal lowlands: geology and geotechnology. Editors W. J. M. Van Der Linden, S. A. P. L. Cloetingh, J. P. K. Kaasschieter, W. J. E. Van De Graaff, J. Vandenberghe, and J. A. M. Van Der Gun (Dordrecht: Springer Netherlands), 213–225. doi:10.1007/978-94-017-1064-0
Streif, H. (2004). Sedimentary record of Pleistocene and Holocene marine inundations along the North Sea coast of lower saxony, Germany. Quat. Int. 112, 3–28. doi:10.1016/S1040-6182(03)00062-4
Sukhodolov, A., Engelhardt, C., Krüger, A., and Bungartz, H. (2004). Case study: turbulent flow and sediment distributions in a groyne field. J. Hydraul. Eng. 130, 1–9. doi:10.1061/(ASCE)0733-9429(2004)130:1(1)
Talstra, H., Uijtteewall, W. S. J., and Stelling, G. S. (2005). in Research on river dynamics from geological to operational time scales. Editors H. J. T. Weerts, I. L. Ritsema, and A. G. van Os (Netherlands Centre for River Studies), 52–53.
Valle-Levison, A., Stanev, E., and Badewien, T. H. (2018). Tidal and subtidal exchange flows at an inlet of the Wadden Sea. Estuar. Coast. Shelf Sci. 202, 270–279. doi:10.1016/j.ecss.2018.01.013
van Slobbe, E., de Vriend, H. J., Aarninkhof, S., Lulofs, K., de Vries, M., and Dircke, P. (2013). Building with Nature: in search of resilient storm surge protection strategies. Nat. Hazards 65, 947–966. doi:10.1007/s11069-012-0342-y
Vousdoukas, M. I., Mentaschi, L., Voukouvalas, E., Bianchi, A., Dottori, F., and Feyen, L. (2018). Climatic and socioeconomic controls of future coastal flood risk in Europe. Nature. Clim. Change 8, 776–224. doi:10.1038/s41558-018-0260-4
Walker, D. J. (1991). Sediment transport near groynes in the nearshore zone. J. Coast. Res. 7. 1003–1011.
Wang, Y., Yu, Q., Gao, S., and Flemming, B. (2014). Modeling the effect of progressive grain-size sorting on the scale dependence of back-barrier tidal basin morphology. Cont. Shelf Res. 91, 26–36. doi:10.1016/j.csr.2014.09.006
Wang, Z. B., Hoekstra, P., Burchard, H., Ridderinkhof, H., De Swart, H. E., and Stive, M. J. F. (2012). Morphodynamics of the Wadden Sea and its barrier island system. Ocean Coast. Manag. 68, 39–57. doi:10.1016/j.ocecoaman.2011.12.022
Wang, Z. B., Louters, T., and de Vriend, H. J. (1995). Morphodynamic modelling for a tidal inlet in the Wadden Sea. Mar. Geol. 126, 289–300. doi:10.1016/0025-3227(95)00083-B
Ward, M. A., and Thorpe, J. P. (1989). Assessment of space utilisation in a subtidal temperate bryozoan community. Mar. Biol. 103, 215–224. doi:10.1007/BF00543350
Wasserstraßen- und Schiffahrtsverwaltung des Bundes (WSV) (2021). DGM-W 2012 außenweser/jade. Available at: https://www.kuestendaten.de (Accessed November 30, 2021).
Wu, T., and Qin, J. (2020). Influence of flow and sediment transport processes on sedimentation in groyne fields. J. Coast. Res. 95, 304. doi:10.2112/SI95-059.1
Zhou, Z., Ge, J., Van Maren, D. S., Wang, Z. B., Kuai, Y., and Ding, P. (2021). Study of sediment transport in a tidal channel-shoal system: lateral effects and slack-water dynamics. JGR Oceans 126, e2020JC016334. doi:10.1029/2020JC016334
Keywords: coastal erosion, coastal protection measures, tidal inlets, sediment distribution, sediment dynamics
Citation: Geßner A-L, Wollschläger J, Giebel H-A and Badewien TH (2024) Impact of a submerged stream groyne on morphology and sedimentology on a tidal inlet, Harle (Southern North Sea, Germany). Front. Earth Sci. 12:1292462. doi: 10.3389/feart.2024.1292462
Received: 11 September 2023; Accepted: 22 April 2024;
Published: 15 May 2024.
Edited by:
Andrés Payo, British Geological Survey—The Lyell Centre, United KingdomCopyright © 2024 Geßner, Wollschläger, Giebel and Badewien. This is an open-access article distributed under the terms of the Creative Commons Attribution License (CC BY). The use, distribution or reproduction in other forums is permitted, provided the original author(s) and the copyright owner(s) are credited and that the original publication in this journal is cited, in accordance with accepted academic practice. No use, distribution or reproduction is permitted which does not comply with these terms.
*Correspondence: Anna-Lena Geßner, YW5uYS1sZW5hLmdlc3NuZXJAdW5pLW9sZGVuYnVyZy5kZQ==