- 1PetroChina Hangzhou Research Institute of Geology, Hangzhou, China
- 2Chinnery Assets Limited, Yangon, Myanmar
Channel–levee systems (CLSs) and mass transport complexes (MTCs) are prevalent in deep-water basins, yet their interplay remains enigmatic. This study uses high-resolution 3D seismic data to investigate the architecture of CLSs and MTCs, aiming to explore how CLSs influence the distribution of MTCs in the Rakhine Basin, Bay of Bengal. Two models are built to illustrate the interaction between CLSs and MTCs. In the first model, large-scale aggradational CLSs created unfilled relief in their overbank environments, which acted as spatially confined topography for subsequent mass-wasting deposits. In this model, the interaction between large-scale CLSs and confined MTCs controls the distribution of confined MTCs. In the second model, laterally migrating CLSs do not create such prominent topographic relief in their overbank environments. This characteristic renders them inconsequential in influencing the distribution of subsequent mass-wasting processes. Consequently, spatially extensive MTCs develop within unconfined settings. This configuration gives rise to lithological traps, which might be common and represent potential drilling targets on continental margins. This study could contribute to a better understanding of the interplay between CLSs and MTCs and have some enlightenment on looking to provide some insight into the search for lithological traps in the Rakhine Basin.
1 Introduction
Submarine channels and their flanking levees are commonly referred to as channel–levee systems (CLSs) (Flood and Damuth, 1987), while mass transport complexes (MTCs) are the deposits of subaqueous mass movements such as debris flows, slides, and slumps (Prior et al., 1984; Mulder and Cochonat, 1996; Piper et al., 1997; Sawyer et al., 2009). Both are significant components of the stratigraphic record in ancient and modern deep-water basins worldwide (Flood and Damuth, 1987; Mulder and Cochonat, 1996; Piper et al., 1997). CLSs are usually formed by turbidity currents, with sand-rich channels and mud-rich levees, but both can serve as reservoirs. MTCs are characterized by disordered sediments and dominated by mud, and they could be transported downslope from topographic highs to areas of lower gradient, where they can cover hundreds or thousands of square kilometers; therefore, they are important to act as seals in hydrocarbon exploration (Armitage et al., 2009; Omeru, 2014). However, small MTCs can also occur within large channels or canyons (Sun et al., 2011).
The Bengal Fan, as the world largest submarine fan system, develops the world-class CLSs and MTCs. The initiation and development of the Bengal Fan correspond to the India–Asia collision and subsequent Himalayan uplift and denudation since the Eocene (Curray and Moore, 1971; Cochran, 1990). A regional exploration of the Bengal Fan’s morphology, sedimentology, stratigraphy, and evolutionary history has been comprehensively studied by Curray and Moore (1971), Curray et al. (2003), Cochran (1990), and Alam et al. (2003). Further insights into how detrital sediments from the Himalayas are transported and contribute to forming the world’s largest fan systems in the Bay of Bengal have been provided by Stow and Cochran (1989), Curray (1994), and Galy et al. (1996). Moreover, the sedimentology and stratigraphy of deep-water systems in the Rakhine Basin, offshore Myanmar, have been documented by Sun et al. (2011), Ma et al. (2011), Yang and Kim (2014), and Harrowfield (2015). These studies collectively reveal that CLSs and MTCs are two of the most prominent depositional features in the deep-water areas of the Rakhine Basin, occurring concurrently with similar features worldwide (Posamentier and Kolla, 2003; Moscardelli et al., 2006; Moscardelli and Wood, 2008; Jackson and Johnson, 2009; Sylvester et al., 2011; Gong et al., 2014; Gong et al., 2015).
MTCs can affect seafloor morphology and may control the post-MTC sedimentation, such as submarine channel routes and lobe location (von Huene et al., 2004; Hühnerbach and Masson, 2004; Hühnerbach et al., 2005; Nwoko et al., 2020). Turbidite distribution, affected by high-relief topography formed by MTCs, is characterized by irregular pinch-outs, which present a significant risk for stratigraphic trapping, whereas low-relief topography may form overlying sedimentary bodies that are more extensive (Nwoko et al., 2020). However, the influence of CLSs on the distribution of MTCs remains elusive. This paper provides an excellent opportunity to understand the interaction between CLSs and MTCs through seismically well-imaged examples in the northeast of the Bengal Fan.
2 Geological setting
The study area is located in the Rakhine Basin, Bay of Bengal (Figures 1, 2A), facing the Indian craton to the west, the Shillong massif and the Himalayan belt to the north, and the Indo-Burman ranges to the east (Curray, 1991; Curray and Munasinghe, 1991; Uddin and Lundberg, 1998). Within the Bay of Bengal, three tectonic units are delineated from west to east: passive continental margins, the ocean basin, and the Indo-Myanmar Range (Figure 2B). The Bay of Bengal and the associated Ganges–Brahmaputra–Bengal sediment-routing systems have caught much attention because of their relation to the world’s largest orogenic system (i.e., the Himalayan Range), the world’s largest fluvio-deltaic system (i.e., the Bengal Delta), and the world’s largest submarine fan system (i.e., the Bengal Deep Sea Fan) (Curray, 1991; Curray and Munasinghe, 1991; Alam et al., 2003).
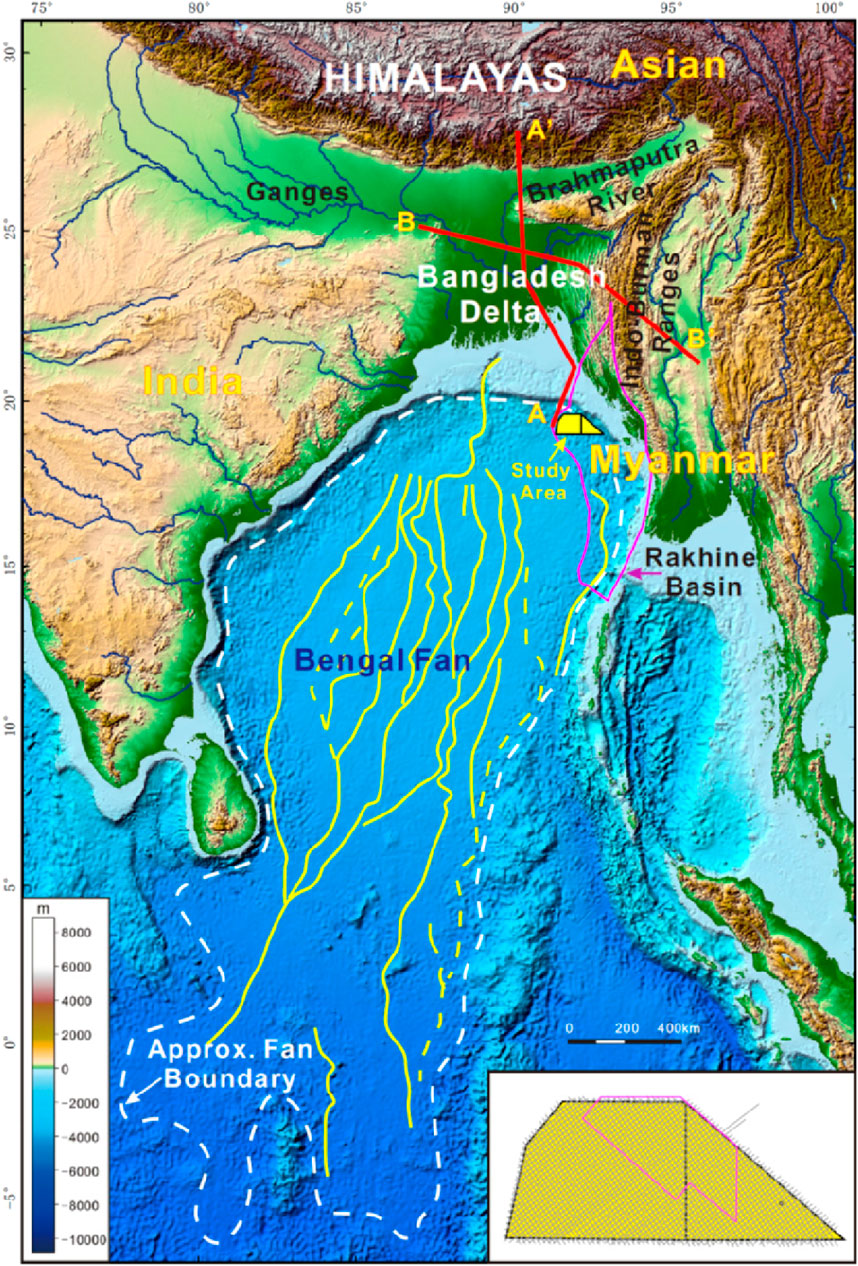
Figure 1. Topobathymetric map of the Bay of Bengal and adjacent regions showing the geographic context of the study area (yellow box) in the Rakhine Basin (pink boundary). The boundaries of the Bengal Fan, locations of geological profiles in Figure 2, and seismic data used are shown (pink boundary in the insert map represents the survey of 3D seismic data, and the gray lines represent the 2D seismic lines).
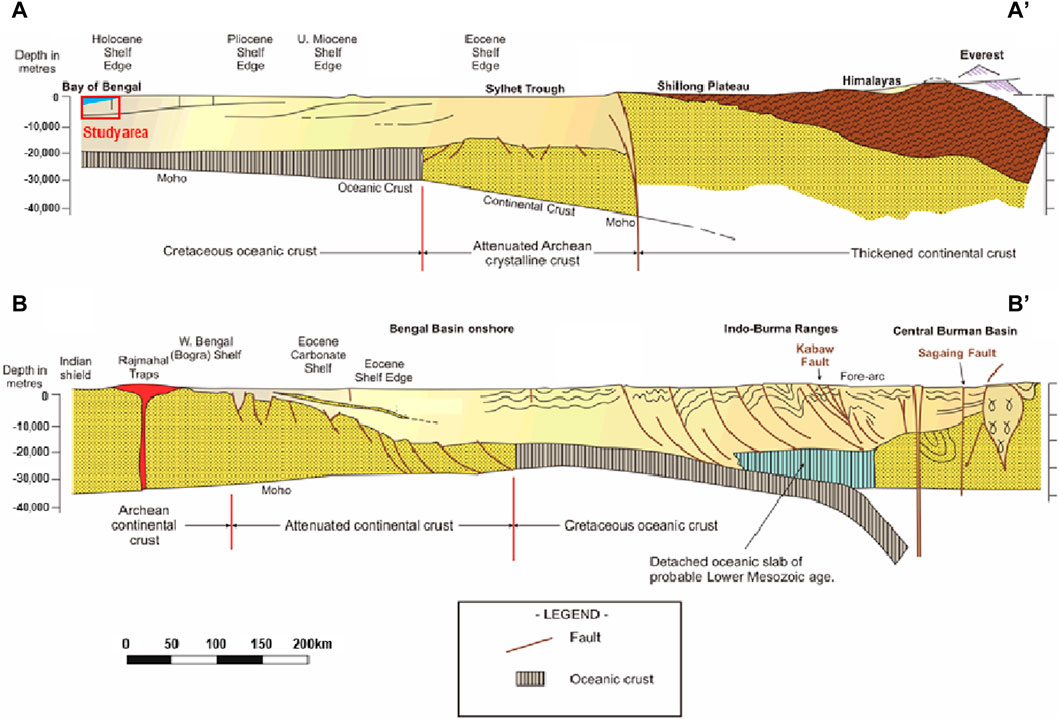
Figure 2. Regional geological profiles [modified from the work of Alam et al. (2003)] showing the geological context of the Bay of Bengal and adjacent regions. The study area is projected in the A–A′ profile.
The Bengal Basin was created by the collision of the Indian Plate with the Eurasian Plate and the uplift of the Himalayas and the Tibetan Plateau following the breakup of Gondwana (Alam et al., 2003; Curray et al., 2003). The tectonic convergence between the Indian Plate and Eurasian Plate experienced two main stages (Cochran, 1990; Beck et al., 1995). The initial India–Asia collision, known as “soft” collision, was proposed to have taken place in the early Eocene (Sclater and Fisher, 1974), middle Eocene (Sahni and Kumar, 1974; Dewey et al., 1988), or late Eocene (Honegger et al., 1982; Petterson and Windley, 1985). The soft India–Asia collision, in turn, led to the transition from marine deposits to non-marine deposits in the Indus Group in the Molasse basins, the southern Indus–Tsangpo suture, and the first appearance of red beds rich in ophiolitic debris in the Tibetan Zone (Sclater and Fisher, 1974; Hodges, 2000). The subsequent India–Asia collision known as “hard” collision occurred after the Middle Miocene (Alam et al., 2003). This hard India–Asia collision, in turn, led to the widespread reorganization of major plates in the eastern Indian Ocean and the tectonic extrusion between the Burma and Sibumasu blocks and Indochina block (Tapponnier et al., 1982; Tapponnier et al., 1986; Alam et al., 2003).
The India–Asia collision and the uplift of the Himalayas and Tibetan plateaus have facilitated the availability of abundant clastic materials, which were transported and delivered into the Bengal Basin, mainly by the Ganges and Brahmaputra rivers, and contributed to the world’s largest submarine fan, spanning approximately 3,000 km in length, approximately 1,000 km in width, and reaching a maximum thickness of up to 16.5 km (Curray et al., 2003; Blum et al., 2018). Two basin-wide unconformities have been recognized in the stratigraphy of the Bengal Fan, i.e., early Eocene and Late Miocene unconformities (Cochran, 1990; Curray et al., 2003; Kneller et al., 2016). The early Eocene unconformity marks the initiation of Bengal Fan growth, with shelf margins migrating consistently southward from the Eocene to the Holocene (Figure 2A) (Cochran, 1990; Alam et al., 2003; Curray et al., 2003; Kneller et al., 2016). The Late Miocene unconformity marks the onset of diffuse plate edge or intra-plate deformation in the southern or lower fan (Cochran, 1990; Curray et al., 2003; Kneller et al., 2016).
The Bengal Fan comprises enormous CLSs from the Pliocene to the Holocene, which were built on top of the pre-existing fan surface during lowered sea level by very large turbidity currents (Prior et al., 1984). The currently active CLS was formed about 12,000 years ago, which extended approximately 3,000 km from the Swatch of No Ground canyon. MTCs have occurred frequently since the Miocene hard collision (Tapponnier et al., 1982; Tapponnier et al., 1986). The deposits of MTCs can reach the thickness of a few hundred meters, while the coverage area is approximately hundreds to thousands of square kilometers. CLSs and MTCs stand out as two of the most prominent element building blocks of the Bengal Fan, which are the focus of this study.
3 Data and methods
The primary datasets used in this study consist of 2D and 3D seismic reflection data. The 2D seismic data were acquired in 2007 by BPG, featuring a dense grid with a spacing of 2 km × 2 km. The acquisition system for 2D seismic data was MSX 24-bit recording instruments. It was performed by the “In-Sea electronics,” remotely powered from the recording room by a 306 VDC power voltage. The 3D seismic data were acquired in 2012, covering an area of 2,000 km2, and were processed using the Kirchhoff pre-stack time migration (PSTM) method. The dominant frequency of the 3D seismic data is approximately 30 Hz, with a bin size spacing of 25 m (in-line) by 12.5 m (cross-line) and a sampling rate of 2 ms. The vertical resolution is approximately 10 m using the interval velocity in a target interval of 2,500 m/s. Both the 2D and 3D seismic data were visualized using SEG negative standard polarity, where a positive reflection coefficient corresponds to an increase in acoustic impedance and is represented by a positive reflection event. Seismic profiles were displayed using a white–black color bar. The low-impedance reservoir top is presented as the peak, denoted by the black color.
The 2D seismic data were used to tie 3D seismic data to the offset well which was drilled to the Miocene in 2015, providing paleontological analysis (such as calcareous nannofossils, foraminifera, and sporopollen) for establishing the stratigraphic framework of the study interval and determining the age of the documented Bengal Fan elements. Seismic attributes, such as variance attributes and root mean square (RMS) amplitude, were extracted along the selected horizons and used to analyze sediment systems. All the seismic data interpretation and attribute extraction are carried out using Petrel software, Schlumberger TM.
4 Results
4.1 Seismic stratigraphy and geomorphology
CLSs and MTCs represent two of most prominent depositional features from the Pliocene to the Quaternary in the Bengal Fan.
4.1.1 Pliocene–Lower Pleistocene large aggradational CLSs
The Pliocene–Lower Pleistocene succession in the study area contains well-imaged large aggradational CLSs (Figure 3). CLSs are typified by a “gull-wing” morphology bounded by a flat seismic horizon at their base (Figure 4). The central parts of CLSs are V-shaped, characterized by high-amplitude, discontinuous reflection, which represent sand-rich channel deposits. The channels exhibit distinctive L-shaped growth trajectories and present a highly aggradational pattern (Figure 4). The single channel is approximately few hundreds of meters wide and tens of meters thick. The flanks of CLSs are wedge-shaped levees, typified by low-amplitude and continuous reflection (Figure 4). The levees are generally symmetric, with a height of 300 ms and a maximum gradient of 6°.
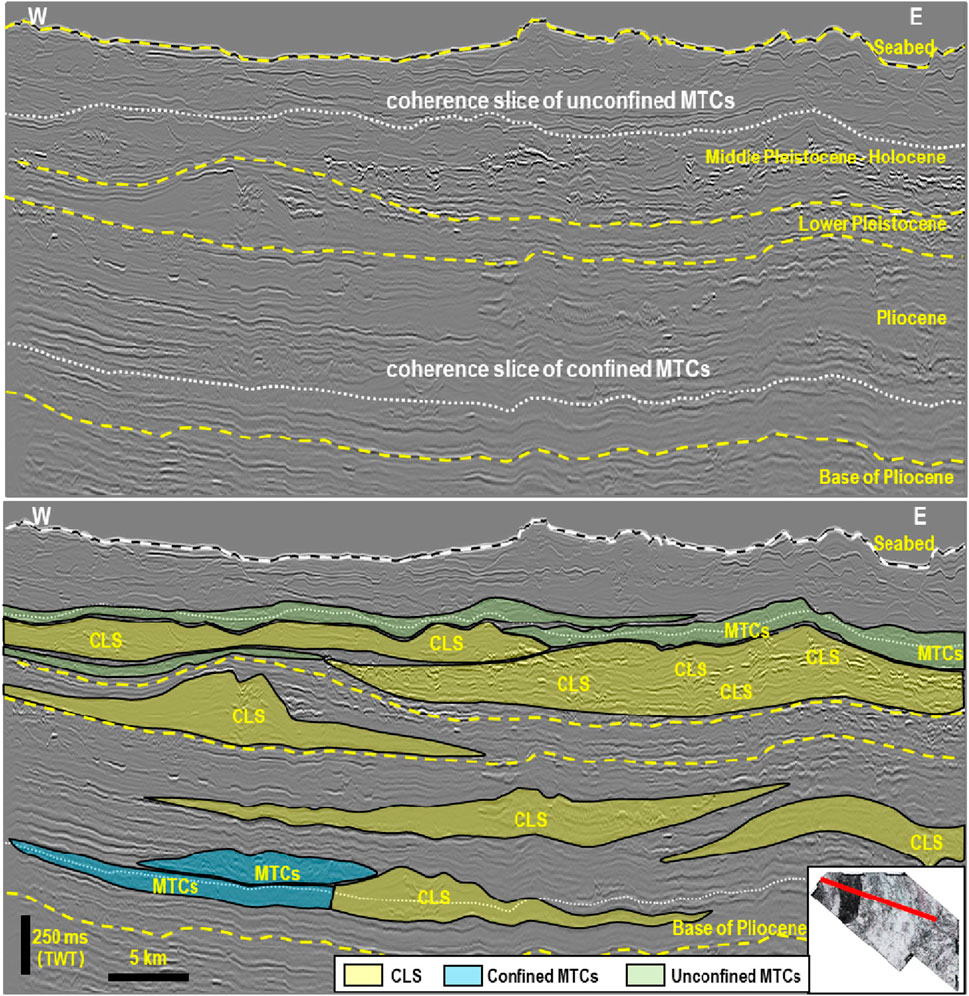
Figure 3. Seismic profile showing stratigraphic architecture of the Pliocene–Quaternary Bengal Fan. It should be noted that the Pliocene–Quaternary succession of the Bengal Fan is dominated by channel–levee systems (CLSs) and mass transport complexes (MTCs). The yellow dashed lines represent the chronostratigraphic layers, while the white dashed lines represent the location of the coherence slice in Figure 6 and Figure 11.
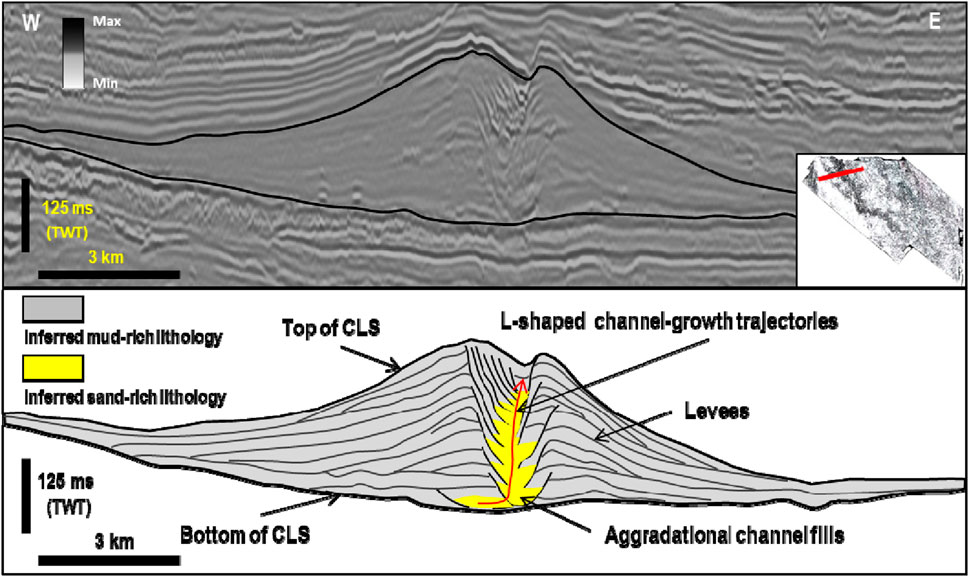
Figure 4. Strike view seismic profile and accompanying line drawings (upper and lower panels, respectively) illustrating the stratigraphic architecture of large aggradational CLSs displaying L-shaped channel-growth trajectories.
CLSs are approximately 10–30 km wide, which is the width between levee terminations, occupying an area larger than 1,000 km2; the channels are long and sinuous (i.e., sinuosity of approximately 2.0–2.5) courses in coherence attribute maps (Figure 5). The strong amplitude lobes located outside the bend of channels are the crevasse splays, which are developed above levees with an area of approximately few hundreds of square kilometers (Figure 5).
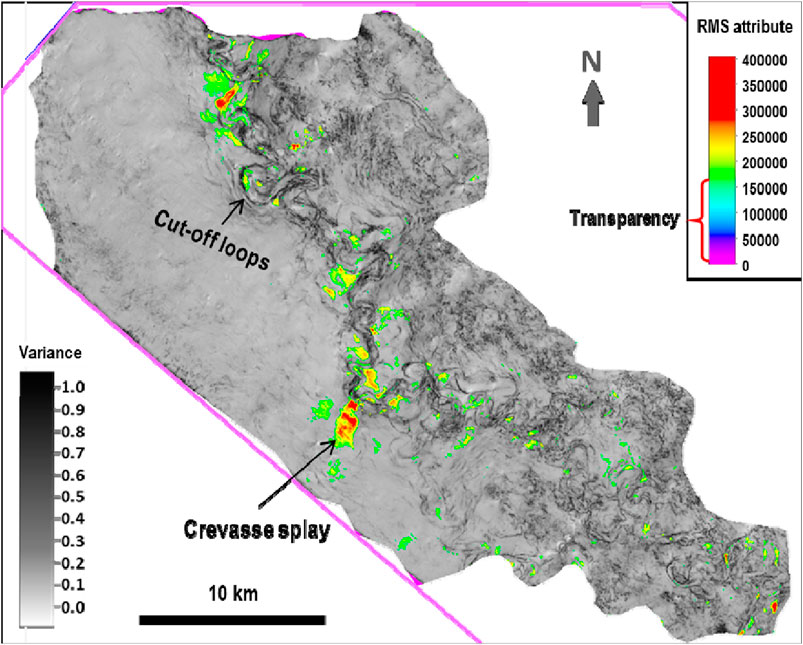
Figure 5. Coherence attribute map merged with RMS attributes showing plan view geomorphological manifestations of large-scale CLSs and their accompanying depositional elements (cut-off loops) and crevasse splays.
4.1.2 Pliocene–Lower Pleistocene confined MTCs
Within the Pliocene–Lower Pleistocene succession of the documented Bengal Fan, we observe the development of irregularly shaped chaotic seismic facies, denoted as MTCs (confined MTC in Figure 3). In plan view, MTCs display an irregular morphology and cover an area of 100 km2 (Figure 6). In flattened horizontal coherence slice, MTCs are imaged as amorphous dark-colored zones and are located at the west of sinuous channel belts. MTCs were transported from north to south, and the erosion boundary is neat and some thrust faults can be seen near the southern boundary (Figure 6). In seismic cross sections, they are characterized by chaotic, low-amplitude reflections with variable continuity and are bounded at their base and topped by undulating seismic horizons (Figure 7). They are approximately 20–100 m and exhibit irregularly shaped chaotic seismic facies and small faults (Figure 7).
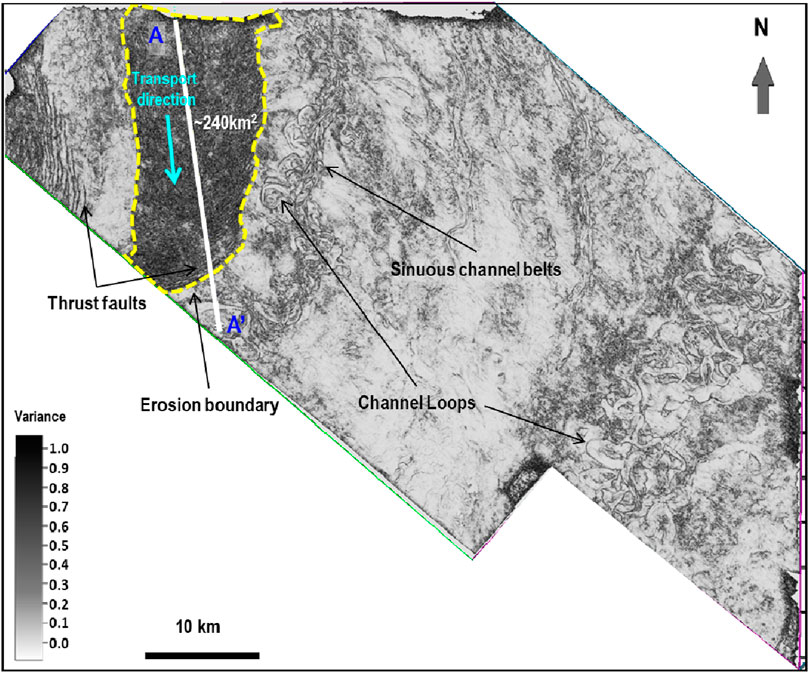
Figure 6. Flattened horizontal coherence slice showing the plan view geomorphological expression of confined MTCs (irregularly shaped chaotic seismic facies). The location of the coherence slice is shown in Figure 3. It should be noted that confined MTCs are localized and developed on channel overbank environments. Line A–A′ indicates the plan view location of the seismic profile shown in Figure 7.
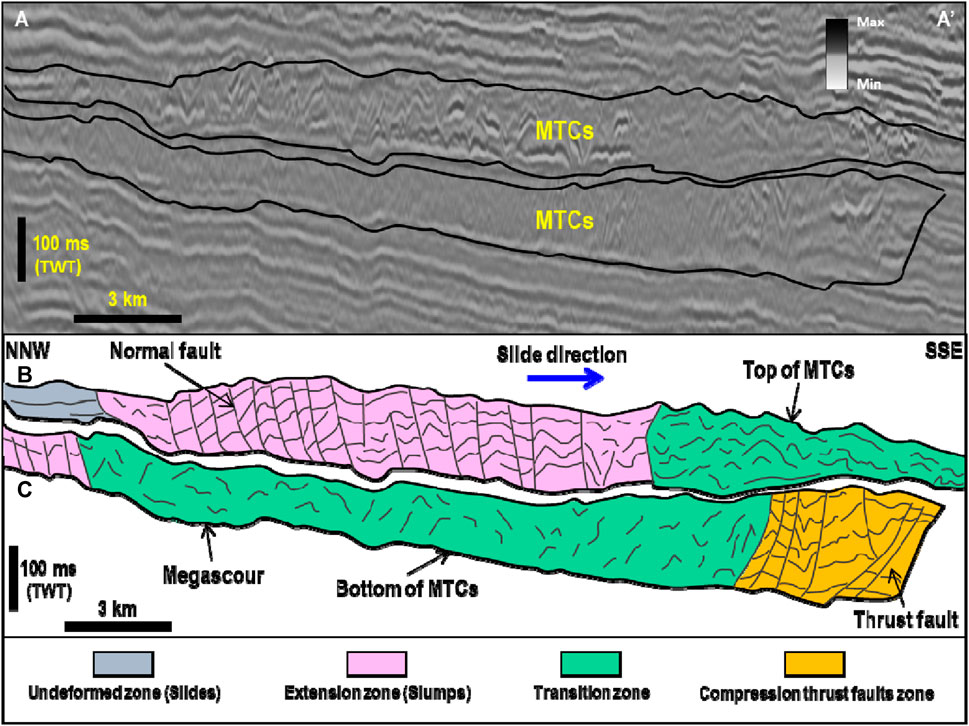
Figure 7. (A) Strike view seismic profile and interpretative line drawings [(B, C), respectively] illustrating confined MTCs as chaotic seismic reflections with an erosional base and irregular top surface. The location of the seismic section is shown in Figure 6.
Similar to blanket chaotic seismic facies, irregularly shaped chaotic seismic facies exhibit many of the recognition criteria (i.e., chaotic, low-amplitude reflections with variable seismic reflection continuity, and irregular-shaped cross-sectional geometries) typically used to recognize MTCs on seismic reflection (Sahni and Kumar, 1974; Honegger et al., 1982; Dewey et al., 1988; Yang and Kim, 2014). MTCs are restricted, completely confined within topographic lows, and can be considered confined MTCs. (Figures 6, 7).
4.1.3 Middle Pleistocene–Holocene migrating CLSs
Migrating CLSs are common within the Middle Pleistocene–Holocene sequence of the documented fan in the Bay of Bengal. In plan view, there are many more sinuous CLSs than in Pliocene systems (Figure 8). The individual channels shown in this figure are smaller than those shown in Figure 6. In strike view, the channels are also V- to U-shaped and are composed of high-amplitude, discontinuous reflections (Figure 9). The lateral shifts of CLSs are common, and they do not exhibit a clear gull-wing morphology. No large CLSs were formed during this interval. The channels were flanked by wedge-shaped, low-amplitude levees with continuous reflectors (Figures 3, 9). Individual CLSs are several hundreds to few kilometers wide and 100–200 m thick and occupy an area of <1,000 km2, which is smaller than the Pliocene–Lower Pleistocene CLSs.
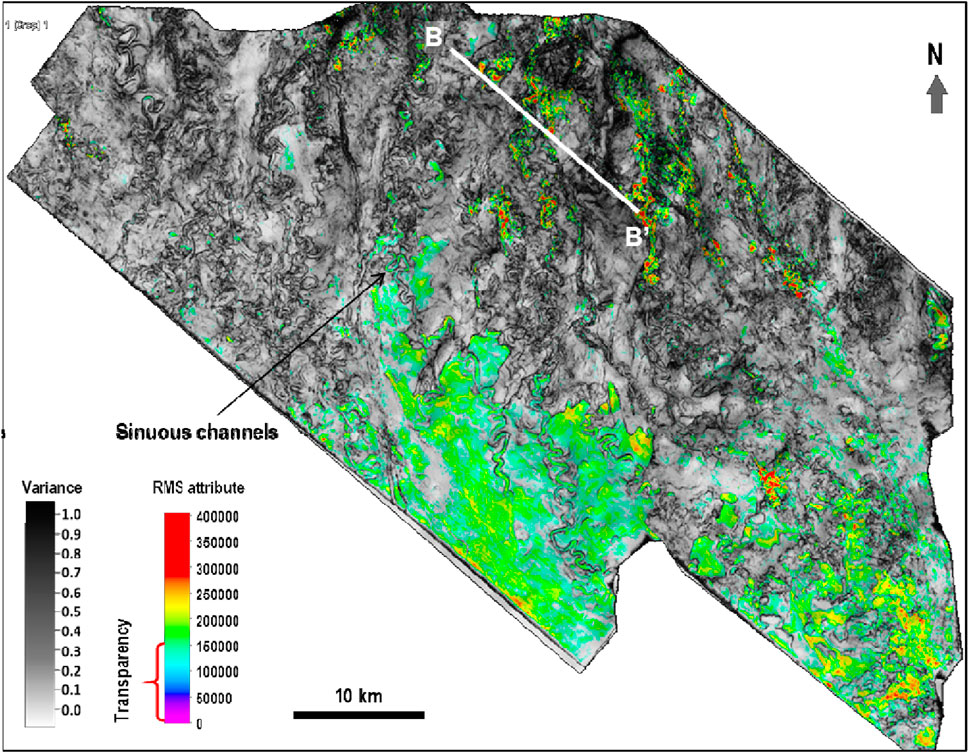
Figure 8. Coherence attribute map merged with RMS attributes showing the plan view geomorphological appearance of migrating CLSs. White dashed line in Figure 9 shows the location of this slice.
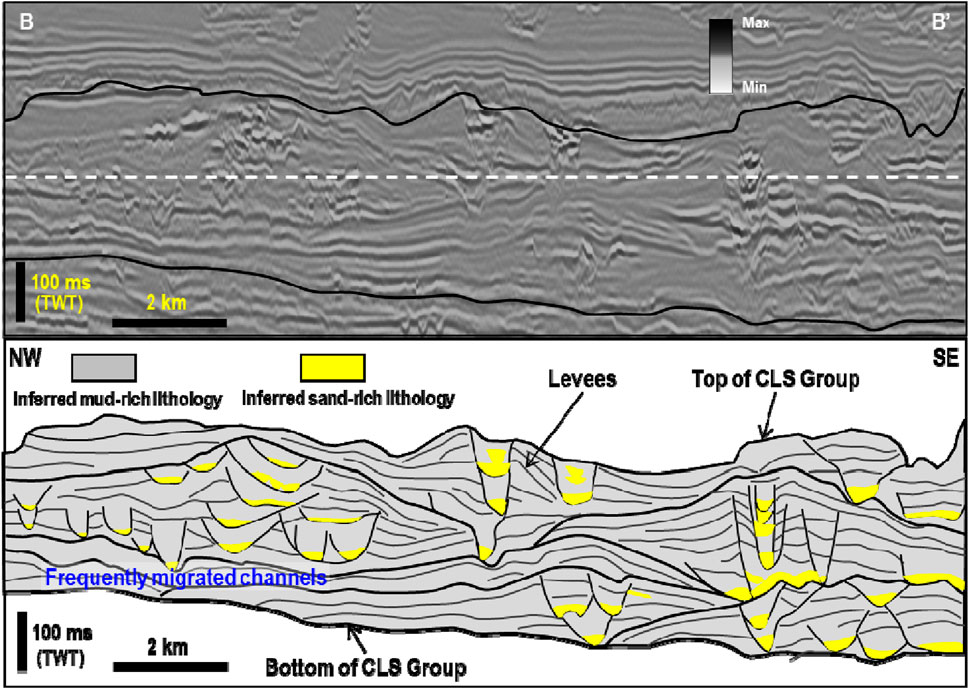
Figure 9. Strike-oriented seismic profile and accompanying interpretative line drawings (upper and lower panels, respectively) illustrating the stratigraphic architecture of small-scale CLSs (i.e., small-scale gull-wing seismic facies). It should be noted that migrated and stacked CLSs do not create obvious topographic relief. The location of this profile is shown in Figure 8.
The discontinuous, high-amplitude reflections confined within their central parts are typically related to thalweg channel fills made up of coarse channel-lag materials (Pirmez et al., 1997; Janocko et al., 2013; Gong et al., 2015; Morelli et al., 2022).
4.1.4 Middle Pleistocene–Holocene unconfined MTCs
The Middle Pleistocene–Holocene succession of the Bengal Fan is also dominated by blanket-like chaotic seismic facies (unconfined MTCs in Figure 3). In cross-sectional view, the unconfined MTCs are characterized by chaotic, low-amplitude, and semitransparent reflectors, covering the laterally migrating CLSs (Figures 4, 10). In plan view, blanket-like chaotic seismic facies are seismically imaged as dark-colored sheets (i.e., high-coherence attributes) and cover an area of 100–1,000 km2 (Figure 11).
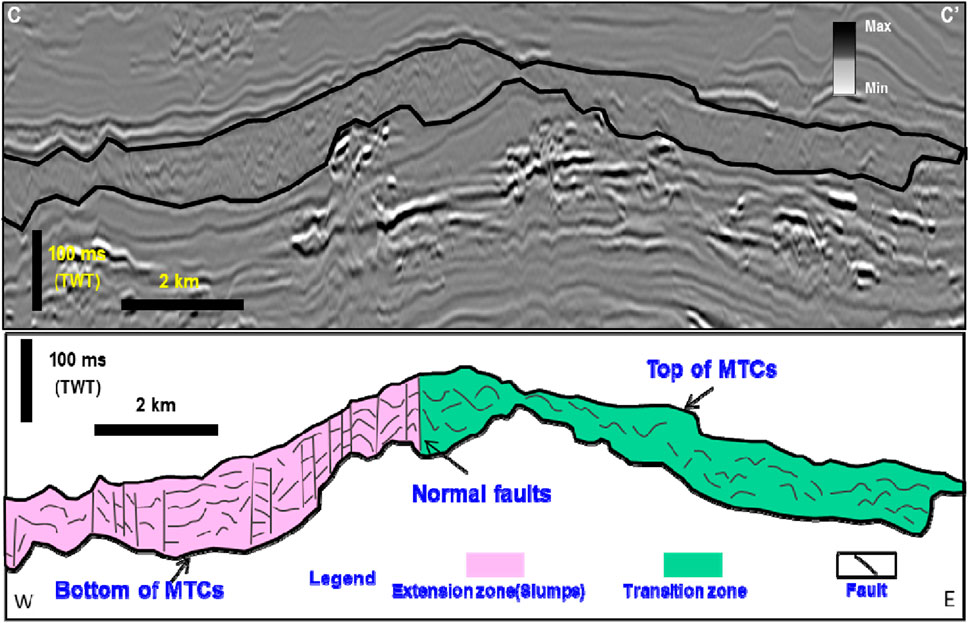
Figure 10. Strike-oriented seismic profile and line drawings (upper and lower panels, respectively) showing the stratigraphic architecture of unconfined MTCs seen as blanket-like chaotic seismic facies. The location of the seismic section shown in the upper panel of this figure is shown in Figure 11.
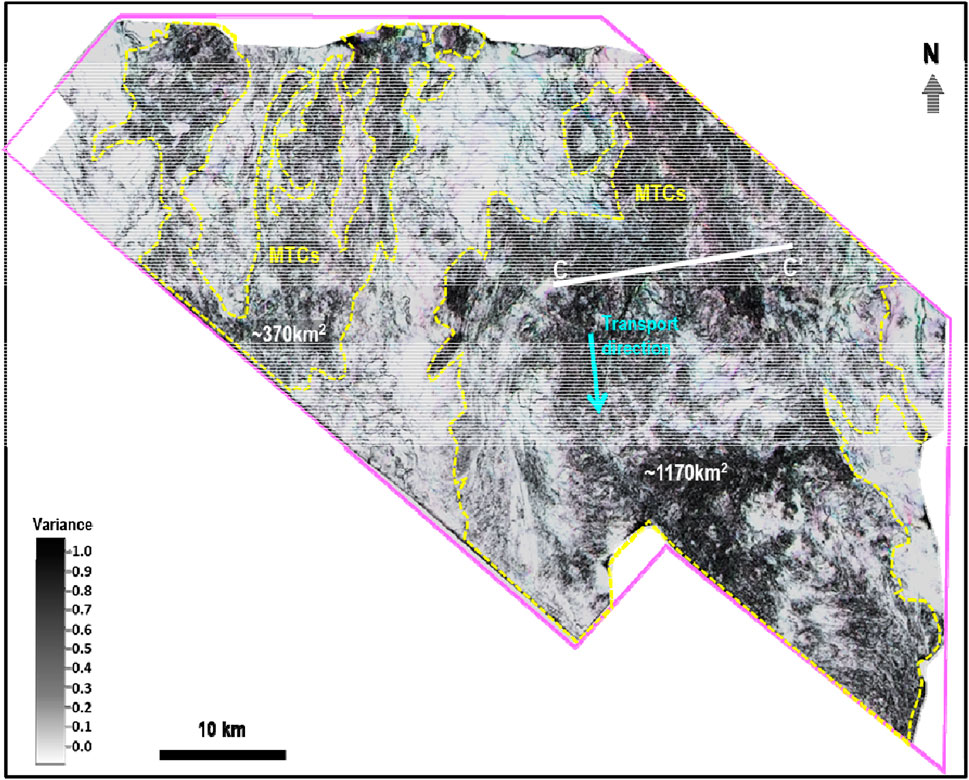
Figure 11. Flattened horizontal coherence slice showing plan view geomorphological manifestations of unconfined MTCs (sheet-like chaotic seismic facies). Line C–C′ indicates the location of the seismic profile shown in Figure 10.
The overall seismic reflection patterns of blanket-like chaotic seismic facies (i.e., chaotic, low-amplitude, and semitransparent) align entirely with the established recognition criteria for MTCs on seismic reflection data (Moscardelli et al., 2006; Moscardelli and Wood, 2008; Jackson and Johnson, 2009; Gong et al., 2014). Similar chaotic seismic facies described in other locations, such as in the Columbus Basin and Santos Basin, have been interpreted as the accumulations of mud-rich sediments (Moscardelli et al., 2006; Jackson and Johnson, 2009). The blanket-like MTCs documented herein are regionally extensive, occurring within unconfined settings, and are interpreted as unconfined MTCs (Figure 10).
4.2 The complex interplay of Pliocene–Quaternary CLSs and MTCs
Our results suggest that the studied CLSs and MTCs intensely interacted with each other, which is regionally manifested in two main ways.
4.2.1 Interaction between Pliocene large aggradational CLSs and MTCs
The Pliocene large aggradational CLSs are 10∼30 km wide and 200 ∼ 350 m thick (Figures 4, 12), creating remarkable topographic relief on the deep sea environment. MTCs are restricted within the topographic low formed by large-scale CLSs and cut deeply into preceding levees (Figures 4, 12, 13). MTCs show a transition to a compressional area from the distal to the proximal levee, and thrust faults can be seen in a compressional area from coherence slice (Figure 6). Such a relationship between large CLSs and MTCs suggests that the obvious topographic relief created by the large-scale CLSs acted as the accommodation space for the subsequent MTCs, and the topographic high created by aggradational channels limited the distribution of MTCs (Figures 4, 12, 13). This interaction model is illustrated graphically in Figure 13.
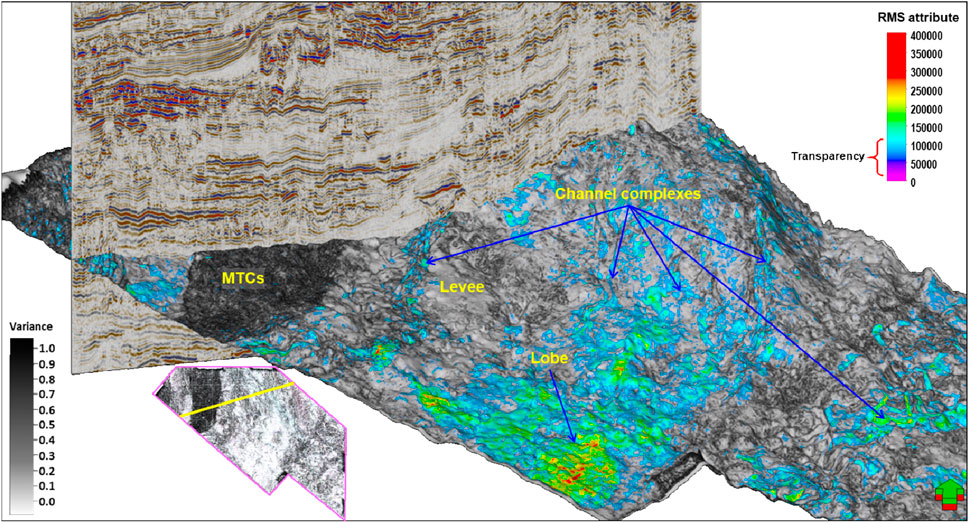
Figure 12. 3D perspective view map showing Pliocene MTCs completely confined by large aggradational CLSs.
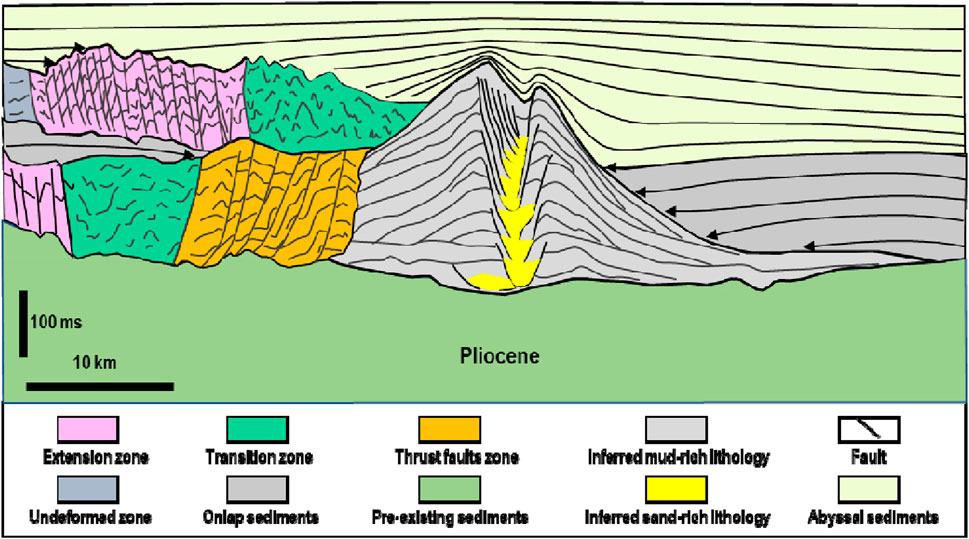
Figure 13. Cartoon model of the interaction between Pliocene channels and MTCs showing that large aggradational CLSs could control the distribution of MTCs. It should be noted that the lower MTCs were blocked by the CLS, forming a local compression environment, resulting in thrust faults, while the upper MTCs do not have an obvious compression environment.
4.2.2 Interaction between Pleistocene–Holocene laterally migrating CLSs and MTCs
CLSs above the Lower Pleistocene are obviously different from Pliocene CLSs due to the frequent migration of channels. CLSs are smaller than Pliocene CLSs, and they are stacked; thereby, no large topographic relief is created by these CLSs (Figures 4, 12). Therefore, subsequent MTCs can completely overlay these CLSs, being, therefore, unconfined as shown in Figure 14. Moreover, unconfined MTCs do not cut deeply into the preceding levees (Figures 10, 14). Such a stratigraphic relationship between smaller and stacked CLSs and unconfined MTCs suggests that Pleistocene–Holocene CLSs had a limited influence on controlling the distribution of overlying MTCs. This interaction model is illustrated graphically in Figure 14.
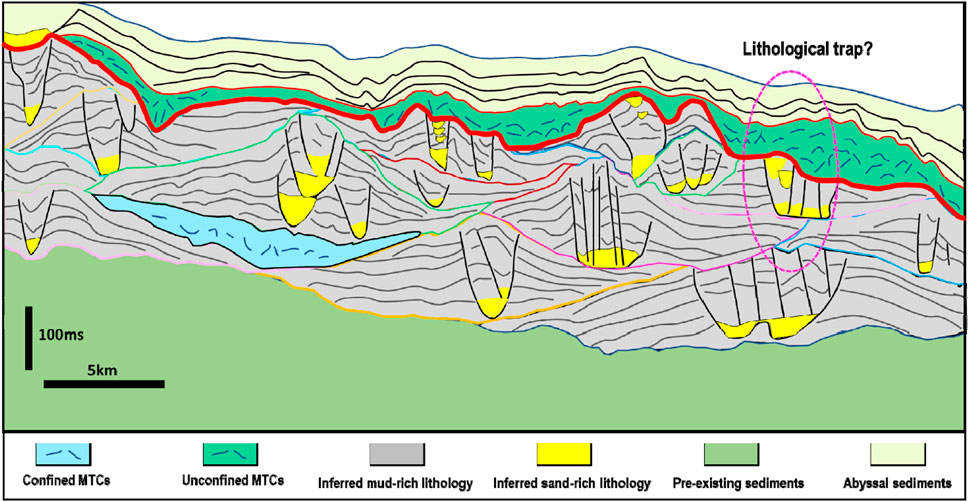
Figure 14. Cartoon model of the interaction between migrating CLSs and unconfined MTCs. This model of channel–MTC interaction shows that migrating CLSs are wholly capped by unconfined MTCs.
5 Discussion
5.1 Implications for understanding the interaction between two prominent deep-water elements
CLSs and MTCs are two of the most prominent depositional elements on continental margins worldwide (Posamentier and Kolla, 2003; Moscardelli et al., 2006; Moscardelli and Wood, 2008; Jackson and Johnson, 2009; Sylvester et al., 2011; Gong et al., 2014; Gong et al., 2015). They co-exist in deep-water settings, leading to extensive interactions. Various studies have documented their interaction, using outcrop analogs, seismic data, bathymetric data, well and core data, and numerical models (Piper and Normark, 2001; Jackson and Johnson, 2009; Bernhardt et al., 2012; Stright et al., 2013; Masalimova et al., 2015; Corella et al., 2016; Casalbore et al., 2019). While previous researches have primarily focused on the role of MTC-related topography in influencing the distribution of deep-water channels and how MTC-related topography usually determined the position of submarine channels (Hansen et al., 2013; Kneller et al., 2016; Qin et al., 2017), the influence of CLSs on the distribution of MTCs remains elusive. Our results suggest that the interaction between CLSs and MTCs on the Pliocene to Quaternary Bengal Fan is regionally manifested in two ways.
First, large-scale Pliocene aggradational CLSs formed a significant topographic relief (approximately 300 m); when MTCs occurred later, MTCs will be confined by this huge topographic high; thus, CLSs played a pivotal role in determining the distribution of MTCs (Figure 13). The topographic lows served as pathways for the movement of subsequent mass-wasting processes, resulting in confined MTCs (Figures 7, 12, 13). However, this topographic relief cannot be formed everywhere, it requires a stable and large supply of fine-grained sediment, and no major tectonic activity is also necessary. Usually, only huge submarine fan systems meet these conditions, like the Bengal and the Amazon fans (Flood and Damuth, 1987; Manley et al., 1997; Curray et al., 2003). Second, laterally migrating Pleistocene–Holocene CLSs create a topography with limited relief, which cannot restrict the movement of subsequent mass-wasting processes. Therefore, MTCs were transported on an unconfined setting and covered the whole area of CLSs (Figures 11, 14, 15).
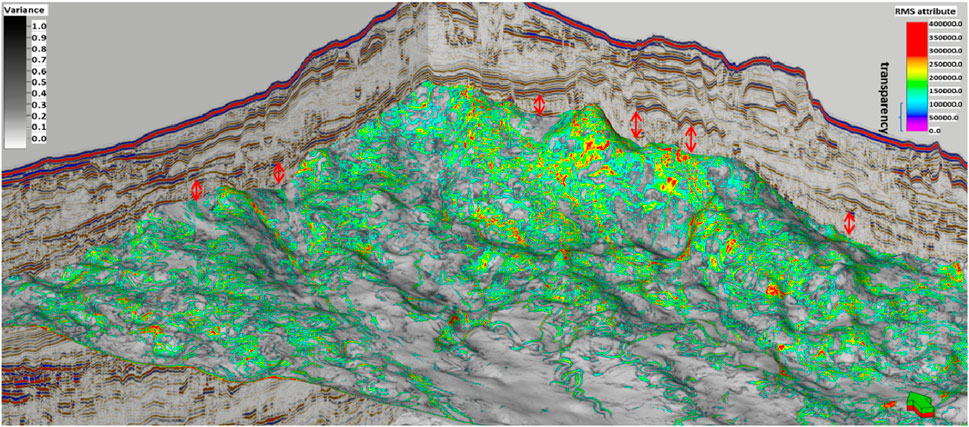
Figure 15. 3D perspective view map showing laterally migrating CLSs of late Pleistocene–Holocene age wholly covered by unconfined MTCs.
5.2 Lithological traps created by CLSs and MTCs
Thalweg high-amplitude reflections [“HARs” (Flood et al., 1991)] are observed within V- to U-shaped channel cross sections, featuring distinct high-amplitude reflector terminations (Figures 4, 8, 13, 14). In plan view, they are expressed as amorphous high-RMS accumulations (Figures 4, 8, 13, 14). In locations where similar HAR seismic facies have been calibrated with borehole data, such as in the Amazon fans, they have been proven to be made up of coarse sand-rich turbidites in the channel, i.e., reservoirs (Mayall et al., 2006). In contrast, both confined and unconfined MTCs are generally interpreted as poor reservoirs but can be excellent seals (Moscardelli et al., 2006; Jackson and Johnson, 2009; Gong et al., 2014). Although there are few examples where MTCs can act as reservoirs (Arfai et al., 2016; Cardona et al., 2020), in those cases, the reservoirs are sliding sandy blocks that have not undergone significant deformation; however, in the study area, there are no obvious sliding blocks in MTCs. In addition, in the locations where similar seismic facies have been identified, such as the Columbus Basin along offshore Trinidad, the Santos Basin along the offshore Brazil, and in the Qiongdongnan Basin along the northern South China Sea margin, they have been collectively related to the accumulation of mud-rich materials (Moscardelli et al., 2006; Jackson and Johnson, 2009; Gong et al., 2014). Considering the location of MTCs in this study, they may originate from the continental slope rather than from the CLS itself.
The confined MTCs are entirely restricted within the topographic lows formed by large-scale CLSs. Therefore, they are surrounded by mud-prone levee sediments and covered by successive MTCs or CLSs. This configuration makes it challenging to form good reservoir-seal assemblages (Figure 13). The unconfined MTCs completely cap the underlying CLS, which could form lithological traps (Figure 14). These lithological traps are expressed in a cross-sectional view as channel-fill sands blanketed and overlain by low-permeability and low-porosity MTCs (Figure 14). They appear in plan view as channel-fill sandy turbidites that are surrounded by the contemporary muddy levee and blanketed by subsequent unconfined MTCs (Figures 11, 14). The interplay between CLSs and unconfined MTCs can be a common phenomenon on continental margins worldwide, suggesting that lithological traps created by this interaction might be common and represent potential drilling targets on other continental margins.
6 Conclusion
(1) Two main types of CLSs have been recognized on the Pliocene–Quaternary Bengal Fan. Pliocene–Early Pleistocene large aggradational CLSs are 10∼ 30 km wide and 200∼ 350 m thick and acted to create huge topographic relief on the sea floor environments. Laterally migrating Late Pleistocene–Holocene CLSs , in contrast, are 0.2 ∼ 1 km wide and 100–200 m thick and do not generate prominent topographic relief.
(2) Two main types of MTCs have been identified in the Pliocene–Quaternary succession of the Bengal Fan. Confined Pliocene–Early Pleistocene MTCs are 100 km2 in area and are seen to fill topographic depressions created by large aggradational CLSs. Unconfined Pleistocene–Holocene MTCs are 100–1,000 km2 in area and are seen to cap and overlie the whole underlying laterally migrating CLSs.
(3) The confined MTCs are blocked by large aggradational CLSs and cannot cover sandy channels at the top of CLSs. Lithological traps for hydrocarbon accumulation are difficult to form. The laterally migrating CLSs do not form much topography relief; when large MTCs happen, MTCs will cover the entire CLSs, forming effective sealing for the channel-filled sandy sediments, creating favorable conditions for reservoir-seal assemblages and lithological traps for hydrocarbon accumulation.
Data availability statement
The original contributions presented in the study are included in the article/Supplementary Material; further inquiries can be directed to the corresponding author.
Author contributions
DS: conceptualization, formal analysis, methodology, project administration, supervision, validation, writing–original draft, and writing–review and editing. GF: conceptualization, formal analysis, methodology, project administration, writing–original draft, and writing–review and editing. HM: conceptualization, data curation, formal analysis, software, writing–original draft, and writing–review and editing. HW: conceptualization, formal analysis, methodology, software, validation, and writing–original draft. LD: data curation, formal analysis, investigation, software, and writing–original draft. GZ: conceptualization, data curation, formal analysis, resources, software, and writing–original draft. YL: formal analysis, investigation, software, and writing–original draft. XX: data curation, investigation, resources, software, and writing–original draft.
Funding
The author(s) declare financial support was received for the research, authorship, and/or publication of this article. This work was funded by the Science and technology project of CNPC, China (Grant No. 2021DJ24).
Acknowledgments
The authors thank Buqing Shi for his guidance and review that improved the focus of the manuscript. They acknowledge the support of the Quantitative Clastics Laboratory, Bureau of Economic Geology, the University of Texas at Austin. They also benefited greatly from the stimulating conversations with QCL folks at UT Austin (Jacob A. Covault, Dallas B. Dunlap, Zoltan Sylvester, Peter Flaig, and Jinyu Zhang).
Conflict of interest
Author HW was employed by Chinnery Assets Limited.
The remaining authors declare that the research was conducted in the absence of any commercial or financial relationships that could be construed as a potential conflict of interest.
The authors declare that this study received funding from CNPC. The funder had the following involvement in the study: data collection and analysis.
Publisher’s note
All claims expressed in this article are solely those of the authors and do not necessarily represent those of their affiliated organizations, or those of the publisher, the editors, and the reviewers. Any product that may be evaluated in this article, or claim that may be made by its manufacturer, is not guaranteed or endorsed by the publisher.
References
Alam, M., Alam, M. M., Curray, J. R., Chowdhury, M. L. R., and Gani, M. R. (2003). An overview of the sedimentary geology of the Bengal Basin in relation to the regional tectonic framework and basin-fill history. Sediment. Geol. 155, 179–208. doi:10.1016/s0037-0738(02)00180-x
Arfai, J., Lutz, R., Franke, D., Gaedicke, C., and Kley, J. (2016). Mass-transport deposits and reservoir quality of upper cretaceous chalk within the German central Graben, North Sea. Int. J. Earth Sci. 105, 797–818. doi:10.1007/s00531-015-1194-y
Armitage, D. A., Romans, B. W., Covault, J. A., and Graham, S. A. (2009). The influence of mass-transport-deposit surface topography on the evolution of turbidite architecture: the Sierra Contreras, Tres Pasos Formation (Cretaceous), southern Chile. J. Sediment. Res. 79 5, 287–301. doi:10.2110/jsr.2009.035
Beck, R. A., Burbank, D. W., Sercombe, W. J., Olson, T. L., and Khan, A. M. (1995). Organic carbon exhumation and global warming during the early Himalayan collision. Geology 23, 387–390. doi:10.1130/0091-7613(1995)023<0387:oceagw>2.3.co;2
Bernhardt, A., Stright, L., and Lowe, D. R. (2012). Channelized debris-flow deposits and their impact on turbidity currents: the Puchkirchen axial channel belt in the Austrian Molasse Basin. Sedimentology 59, 2042–2070. doi:10.1111/j.1365-3091.2012.01334.x
Blum, M., Rogers, K., Gleason, J., Najman, Y, Cruz, J., and Fox, L. (2018). Allogenic and Autogenic signals in the stratigraphic record of the deep-sea Bengal Fan. Sci. Rep. 8, 7973. doi:10.1038/s41598-018-25819-5
Cardona, S., Wood, L., Moscardelli, L., and Dunlap, D. (2020). Cannibalization and sealing of deepwater reservoirs by mass-transport complexes—the Jubilee field, Gulf of Mexico. Interpret. 8 4, SV17–SV30. doi:10.1190/int-2019-0274.1
Casalbore, D., Martorelli, E., Bosman, A., Morelli, E., and Latino Chiocci, F. (2019). Failure dynamics of landslide scars on the lower continental slope of the Tyrrhenian Calabrian margin: insights from an integrated morpho-bathymetric and seismic analysis. Geol. Soc. Lond. Spec. Publ. 477 (1), 389–397. doi:10.1144/sp477.16
Cochran, J. R. (1990). “Himalayan uplift, sea level, and the record of Bengal Fan sedimentation at the ODP leg 116 sites,” in Proceedings of the OceanDrilling Program, Scientific Results, 397–414.
Corella, J. P., Loizeau, J. L., Kremer, K., Hilbe, M., Gerard, J., Le Dantec, N., et al. (2016). The role of mass-transport deposits and turbidites in shaping modern lacustrine deepwater channels. Mar. Petroleum Geol. 77, 515–525. doi:10.1016/j.marpetgeo.2016.07.004
Curray, J. R. (1991). Possiblegreenschist metamorphism at the base of a 22-km sedimentary section, Bay of Bengal. Geology 19, 1097–1100. doi:10.1130/0091-7613(1991)019<1097:pgmatb>2.3.co;2
Curray, J. R. (1994). Sediment volume and mass beneath the Bay of bengal. Earth Planet. Sci. Lett. 125, 371–383. doi:10.1016/0012-821x(94)90227-5
Curray, J. R., Emmel, F. J., and Moore, D. G. (2003). The Bengal Fan: morphology, geometry, stratigraphy, history and processes. Mar. Petroleum Geol. 19, 1191–1223. doi:10.1016/s0264-8172(03)00035-7
Curray, J. R., and Moore, D. G. (1971). Growth of the Bengal deep-sea fan and denudation in the Himalayas. Geol. Soc. Am. Bull. 82, 563–572. doi:10.1130/0016-7606(1971)82[563:gotbdf]2.0.co;2
Curray, J. R., and Munasinghe, T. (1991). Origin of the rajmahal traps and the 85°E ridge: preliminary reconstructions of the trace of the crozet hotspot. Geology 19, 1237–1240. doi:10.1130/0091-7613(1991)019<1237:ootrta>2.3.co;2
Dewey, J. F., Shackleton, R. M., Chengfa, C, and Yiyin, S. (1988). The tectonic evolution of the Tibetan Plateau. Philosophical Transactions of the Royal Society of London. Series A, Mathematical and Physical Sciences 327, 379–413. doi:10.1098/rsta.1988.0135
Flood, R. D., and Damuth, J. E. (1987). Quantitative characteristics of sinuous distributary channels on the Amazon deep-sea fan. Geol. Soc. Am. Bull. 98 6, 728–738. doi:10.1130/0016-7606(1987)98<728:qcosdc>2.0.co;2
Flood, R. D., Manley, P. L., Kowsmann, R. O., Appi, C. J., and Pirmez, C. (1991). in Seismic facies and late quaternary growth of Amazon submarine fan. Seismic Facies and sedimentary Processes of submarine Fans and turbidite systems. Editors P. Weimer, and M. H. Link, 415–433.
Galy, A., France-Lanord, C., and Derry, L. A. (1996). The late oligocene-early Miocene himalayan belt constraints deduced from isotopic compositions of early Miocene turbidites in the bengal fan. Tectonophysics 260, 109–118. doi:10.1016/0040-1951(96)00079-0
Gong, C., Wang, Y., Hodgson, D. M., Zhu, W., Li, W., Xu, Q., et al. (2014). Origin and anatomy of two different types of mass-transport complexes: a 3D seismic case study from the northern South China Sea margin. Mar. Petroleum Geol. 54, 198–215. doi:10.1016/j.marpetgeo.2014.03.006
Gong, C., Wang, Y., Pyles, D. R., Steel, R. J., Xu, S., Xu, Q., et al. (2015). Shelf-edge trajectories and stratal stacking patterns: their sequence-stratigraphic significance and relation to stylesof deep-water sedimentation and amount of deep-water sandstone. AAPG Bull. 99, 1211–1243. doi:10.1306/01311513229
Hansen, L., L’Heureux, J. S., Sauvin, G., Polom, G., Lecomte, I., Vaneste, M., et al. (2013). Effects of mass-wasting on the stratigraphic architecture of a fjord-valley fill: correlation of onshore, shear-wave seismic and marine seismic data at Trondheim, Norway. Sediment. Geol. 289, 1–18. doi:10.1016/j.sedgeo.2013.02.002
Harrowfield, G. (2015). “Mass transport complexes of the Rakhine Basin, Myanmar – deepwater examples from recent 3D seismic data,” in SEAPEX Exploration Conference. (Abstract).
Hodges, K. V. (2000). Tectonics of the Himalaya and southern Tibet from two perspectives. GSA Bull. 112, 324–350. doi:10.1130/0016-7606(2000)112<324:tothas>2.0.co;2
Honegger, K., Dietrich, V., Frank, W., Gansser, A., Thoni, M., and Trommsdorff, V. (1982). Magmatism and metamorphism in the ladakh Himalayas (the indus-tsangpo suture zone). Earth Planet. Sci. Lett. 60, 253–292. doi:10.1016/0012-821x(82)90007-3
Hühnerbach, V., and Masson, D. G. (2004). Landslides in the North Atlantic and its adjacent seas: an analysis of their morphology, setting and behaviour. Mar. Geol. 213 1-4, 343–362. doi:10.1016/j.margeo.2004.10.013
Hühnerbach, V., Masson, D. G., Bohrmann, G., Bull, J. M., and Weinrebe, W. (2005). Deformation and submarine landsliding caused by seamount subduction beneath the Costa Rica continental margin—new insights from high-resolution sidescan sonar data. Geol. Soc. Lond. Spec. Publ. 244 1, 195–205. doi:10.1144/gsl.sp.2005.244.01.12
Jackson, C. A. L., and Johnson, H. D. (2009). Sustained turbidity currents and their interaction with debrite-related topography; Labuan Island, offshore NW Borneo, Malaysia. Sediment. Geol. 219, 77–96. doi:10.1016/j.sedgeo.2009.04.008
Janocko, M., Nemec, W., Henriksen, S., and Warcho, M. (2013). The diversity of deep-water sinuous channel belts and slope valley-fill complexes. Mar. Petroleum Geol. 41, 7–34. doi:10.1016/j.marpetgeo.2012.06.012
Kneller, B., Dykstra, M., Fairweather, L., and Milana, J. P. (2016). Mass-transport and slope accommodation: implications for turbidite sandstone reservoirs. AAPG Bull. 100, 213–235. doi:10.1306/09011514210
Ma, H. X., Lyu, F. L., Fan, G. Z., Sun, H., and Gao, C. X. (2011). Seismic responses and geological characteristics of mass-transport deposits in theRakhine Basin, Offshore Myanmar. Oil Gas Geol. 32, 751–759. doi:10.11743/ogg20110514
Manley, P. L., Pirmez, C., Busch, W., and Cramp, A. (1997). “Grain-size characterization of Amazon Fan deposits and comparison to seismic facies units,”. Proceedings of the ocean drilling program. Editors R. D. Flood, D. J. W. Piper, A. Klaus, and L. C. Peterson (Scientific Results), 155, 35–52.
Masalimova, L., Lowe, D. R., Mchargue, T., and Derksen, R. (2015). Interplay between an axial channel belt, slope gullies and overbank deposition in the Puchkirchen Formation in the Molasse Basin, Austria. Sedimentology 62, 1717–1748. doi:10.1111/sed.12201
Mayall, M., Jones, E., and Casey, M. (2006). “Turbidite channel reservoirse key elements infacies prediction and effective development,”. Marine PetroleumGeology. Editor M. Casey, 23, 821–841.
Morelli, E., Martorelli, E., Casalbore, D., and Chiocci, F. L. (2022). Morpho-stratigraphic evolution of a tectonically controlled canyon-channel system in the gioia basin (southern tyrrhenian sea). Mar. Geol. 451, 106881–106915. doi:10.1016/j.margeo.2022.106881
Moscardelli, L., and Wood, L. (2008). New classification system for mass transport complexes in offshore Trinidad. Basin Res. 20, 73–98. doi:10.1111/j.1365-2117.2007.00340.x
Moscardelli, L., Wood, L., and Mann, P. (2006). Mass-transport complexes and associated processes in the offshore area of Trinidad and Venezuela. AAPG Bull. 90, 1059–1088. doi:10.1306/02210605052
Mulder, T., and Cochonat, P. (1996). Classification of offshore mass movements. J. Sediment. Res. 66 1, 43–57. doi:10.1306/D42682AC-2B26-11D7-8648000102C1865D
Nwoko, J., Kane, I., and Huuse, M. (2020). Mass transport deposit (MTD) relief as a control on post-MTD sedimentation: insights from the Taranaki Basin, offshore New Zealand. Mar. Petroleum Geol. 120, 104489. doi:10.1016/j.marpetgeo.2020.104489
Omeru, T. (2014). Mass transport deposits: implications for reservoir seals. Diss: Cardiff University.
Petterson, M. G., and Windley, B. F. (1985). Rb-Sr dating of the Kohistan arc-batholith in the Trans-Himalaya of north Pakistan, and tectonic implications. Earth Planet. Sci. Lett. 74, 45–57. doi:10.1016/0012-821x(85)90165-7
Piper, D. J. W., Pirmez, C., Manley, P. L., Long, D., Flood, R. D., Normark, W. R., et al. (1997). “Mass-transport deposits of the Amazon fan,” in Proceedings-Ocean Drilling Program Scientific Results (National Science Foundation). doi:10.2973/odp.proc.sr.155.212.1997
Piper, D. J. W., and Normark, W. R. (2001). Sandy fans—from Amazon to hueneme and beyond. AAPG Bull. 85, 1407–1438. doi:10.1306/8626cacd-173b-11d7-8645000102c1865d
Pirmez, C., Hiscott, R. N., and JrKronen, J. D. (1997). Sandy turbidite successions at the base of channel-levee systems of the Amazon fan revealed by FMS logs and cores: unravelling the facies architecture of large submarine fans. Proc. Ocean Drill. Program, Sci. Results 155, 7–33. doi:10.2973/odp.proc.sr.155.201.1997
Posamentier, H. W., and Kolla, V. (2003). Seismic geomorphology and stratigraphy ofdepositional elements in deep-water settings. J. Sediment. Res. 73, 367–388. doi:10.1306/111302730367
Prior, D. B., Bornhold, B. D., and Johns, M. W. (1984). Depositional characteristics of a submarine debris flow. J. Geol. 92 6, 707–727. doi:10.1086/628907
Qin, Y. P., Alves, T. M., Constantine, J., and Gamboa, D. (2017). The role of mass wasting in the progressive development of submarine channels (espírito santo basin, Se Brazil). J. Sediment. Res. 87, 500–516. doi:10.2110/jsr.2017.18
Sahni, A., and Kumar, V. (1974). Paleogene paleobiogeography of the Indian subcontinent. Palaeogeogr. Palaeoclimatol. Palaeoecol. 15, 09–226. doi:10.1016/0031-0182(74)90016-9
Sawyer, D. E., Flemings, P. B., Dugan, B., and Germaine, J. T. (2009). Retrogressive failures recorded in mass transport deposits in the Ursa Basin, Northern Gulf of Mexico. J. Geophys. Res. Solid Earth 114, B10. doi:10.1029/2008jb006159
Sclater, J. G., and Fisher, R. L. (1974). Evolution of the east central Indian ocean, with emphasis on the tectonic setting of the Ninetyeast ridge, 85. Geological Society of America Bulletin, 683–702. doi:10.1130/0016-7606(1974)85<683:EOTECI>2.0.CO;2
Stow, D. A. V., and Cochran, J. R. (1989). The Bengal Fan: some preliminary results from ODP drilling. Geo-Marine Lett. 9, 1–10. doi:10.1007/bf02262812
Stright, L., Bernhardt, A., and Boucher, A. (2013). DFTopoSim: modeling topographically-controlled deposition of subseismic scale sandstone packages within a mass transport dominated deep-water channel belt. Math. Geosci. 45, 277–296. doi:10.1007/s11004-013-9444-7
Sun, H., Fan, G. Z., Lyu, F. L., Xu, Z. C., and Ma, H. X. (2011). Sedimentary characteristics of pliocene slope channel complexes in the Rakhine Basin. offshore Myanmar. actasedimentologicasinica. 29, 695–703.
Sylvester, Z., Pirmez, C., and Cantelli, A. (2011). A model of submarine channel-levee evolution based on channel trajectories: implications for stratigraphic architecture. Mar. Petroleum Geol. 28, 716–727. doi:10.1016/j.marpetgeo.2010.05.012
Tapponnier, P., Peltzer, G., and Armijo, R. (1986). On the mechanics of the collision between India and Asia, 19. Geological Society Special Publication, 15–157. doi:10.1144/GSL.SP.1986.019.01.07
Tapponnier, P., Peltzer, G., Le Dain, A. Y., Armijo, R., and Cobbold, P. (1982). Propagating extrusion tectonics in Asia: new insights from simple experiments with plasticine. Geology 10, 611–616. doi:10.1130/0091-7613(1982)10<611:petian>2.0.co;2
Uddin, A., and Lundberg, N. (1998). Cenozoic history of the Himalayan-Bengal system: sand composition in the Bengal basin, Bangladesh. GSA Bull. 110, 497–511. doi:10.1130/0016-7606(1998)110<0497:chothb>2.3.co;2
von Huene, R., Ranero, C. R., and Watts, P. (2004). Tsunamigenic slope failure along the Middle America Trench in two tectonic settings. Mar. Geol. 203 3-4, 303–317. doi:10.1016/s0025-3227(03)00312-8
Keywords: channel–levee systems, mass transport complexes, interaction, Rakhine Basin, offshore Myanmar
Citation: Shao D, Fan G, Ma H, Wang H, Ding L, Zuo G, Lu Y and Xu X (2024) The complex interaction between channel–levee systems and mass transport complexes in the Pliocene–Quaternary Rakhine Basin, offshore Myanmar. Front. Earth Sci. 12:1286229. doi: 10.3389/feart.2024.1286229
Received: 31 August 2023; Accepted: 26 February 2024;
Published: 18 March 2024.
Edited by:
Ángel Puga-Bernabéu, University of Granada, SpainReviewed by:
Daneiele Casalbore, Sapienza University of Rome, ItalyDawei Wang, Chinese Academy of Sciences (CAS), China
Copyright © 2024 Shao, Fan, Ma, Wang, Ding, Zuo, Lu and Xu. This is an open-access article distributed under the terms of the Creative Commons Attribution License (CC BY). The use, distribution or reproduction in other forums is permitted, provided the original author(s) and the copyright owner(s) are credited and that the original publication in this journal is cited, in accordance with accepted academic practice. No use, distribution or reproduction is permitted which does not comply with these terms.
*Correspondence: Dali Shao, c2hhb2RsX2h6QDE2My5jb20=