- State Key Laboratory of Earthquake Dynamics, Institute of Geology, China Earthquake Administration, Beijing, China
Various lab-scale friction tests and seismic observations have highlighted the role of fault gauges in earthquake initiation in geological faults. These fault gauges consist of particles accumulated over thousands of years due to surface wear caused by friction. Understanding their properties is crucial as they significantly influence both the frictional strength and sliding stability of faults. This study investigates the friction stability parameter (a-b) under loading rates of 0.2–25 μm/s using velocity step tests on gypsum fault gauges under a low normal stress condition (0.9 MPa) and steady-state velocity step tests on fault gauges composed of varying ratios of kaolinite/calcite mixture under an effective normal stress of 3 MPa. The conclusions drawn from this study are as follows: 1) The (a-b) values obtained from near steady-state velocity step tests on gypsum fault gauges and those reported in previous studies under similar conditions were both negative. However, our results show that the former values were one order of magnitude lower than the latter, indicating a higher susceptibility to velocity weakening. 2) Steady-statevelocity steptests on the kaolinite/calcite mixture fault gauges demonstrated positive (a-b) values for all mixtures with varying kaolinite contents. Moreover, the (a-b) values were proportional to the kaolinite content. We established a functional relationship between the (a-b) values of the mixture fault gauge and the mass fraction of kaolinite, providing valuable insights for future experiments and numerical simulations related to fault stability.
1 Introduction
Earthquakes are natural phenomena resulting from abrupt accelerated sliding on tectonic faults. This sliding releases energy through seismic waves and heat (Niemeijer et al., 2012). Seismic waves propagate through the earth’s crust, causing surface vibrations, and earthquakes with significant magnitudes can result in substantial loss of life and property. Therefore, comprehending earthquake formation processes and mechanisms has become a relentless pursuit for numerous scientific researchers (Scholz, 1989; He et al., 1998; Marone, 1998; Mair et al., 2002; West et al., 2005; BenDavid et al., 2010; Kaproth and Marone, 2013). Brace and Byerlee (1966) introduced one crucial understanding of earthquake mechanisms, who suggested that the stick-slip phenomenon observed during the relative sliding of two rocks in laboratory experiments might be a mechanism for shallow earthquakes.
Since then, extensive laboratory friction experiments have been conducted to investigate the sliding properties of rock-rock interfaces (Johnson and Scholz, 1976; Scholz and Engelder, 1976; Engelder, 1978; Dieterich, 1979; Lockner and Okubo, 1983; Weeks and Tullis, 1985; Tullis, 1988; Kato et al., 1992; Karner and Marone, 2000). Nevertheless, lab-scale friction tests and seismic observations revealed that fault gauges in geological faults may play a critical role in earthquake initiation. These fault gauges consist of particles accumulated through surface wear over thousands of years due to friction.
The properties of fault gauges have a decisive influence on both the frictional strength and sliding stability of faults (Brace and Byerlee, 1966; Johnson et al., 1973; Sammis and Biegel, 1989; Frye and Marone, 2002; Mair et al., 2002; Anthony and Marone, 2005; Marone et al., 2008; Ikari et al., 2015; Scuderi et al., 2015; Jiang et al., 2016; Lieou et al., 2017; Hedayat et al., 2018). However, acquiring natural deep fault gauges without compromising their micromorphs and loading history is nearly impossible. To study the sliding properties of fault gauges, some scholars have used fault gauge samples collected from outcrops of fault surfaces (He et al., 2007). Various granular materials have also been used as simulants for fault gauges, assembled into the sliding surface of simulated faults in laboratories. These include pulverized particles obtained by manually grinding various rocks (Byerlee, 1978; Engelder, 1978; Morrow and Byerlee, 1989; Moore et al., 1997; He et al., 2007; Togo et al., 2011; Lu and He, 2014), industrially produced granular materials like finely ground glass beads (Géminard et al., 1999; Albert et al., 2001; Adjemian and Evesque, 2004; Härtl and Ooi, 2008; Johnson et al., 2013; Lastakowski et al., 2015; Rivière et al., 2018), MgO nanoparticles (Han et al., 2011; Yao et al., 2016), and even kitchen flour (Shinbrot et al., 2012; Leeman et al., 2015).
Within the framework of the rate- and state-dependent friction criterion, velocity weakening is a necessary condition for earthquakes to occur, while velocity strengthening typically cannot cause earthquakes (Scholz, 1998). Recent studies have shown that on some naturally seismogenic faults, creep on the fault during the seismic gap is unevenly distributed across the fault (Freymueller et al., 2000; Chlieh et al., 2008). This means that while some areas inside the fault are self-locking and undergoing stress recovery to prepare for the next earthquake, others are slowly creeping. These observations suggest the simultaneous existence of speed enhancement and velocity weakening on the same fault. In laboratory settings, some scholars have explored the influence of material inhomogeneity on fault belts’ sliding properties (Buijze et al., 2021; Bedford et al., 2022). Consequently, investigating the friction stability of fault gauges with different attributes greatly aids related research on laboratory earthquakes. Prior studies have indicated that gypsum behaves as a speed-weakening material at room temperature (Buijze et al., 2021), meaning that (a-b) < 0. As such, (a-b) of gypsum is fitted through stick-slip experiments and cannot be obtained by steady-state tests of speed steps. Conversely, calcite and kaolinite are velocity-enhanced materials at room temperature (Buijze et al., 2021), signifying that (a-b) > 0. However, the friction properties of their mixtures have not been systematically reported to date. Given these reasons, it holds significant scientific importance to study the changes in friction stability parameters (a-b) throughvelocity steptests of gypsum fault gauges under low normal stress conditions and steady-state tests of speed steps of kaolinite/calcite mixture fault gauges with different proportions.
2 Experimental
The experiment was conducted at the Structural Physics Laboratory of the Institute of Geology, China Earthquake Administration. Gypsum, calcite, and kaolinite were purchased from Aladdin Company. We controlled the particle size of the gypsum fault gauge to 100–150 μm and that of kaolinite and calcite to 75–100 μm through grinding and sieving. To enhance the rigidity of the entire system and promote stable fault sliding, we used steel blocks for the surrounding rock of the fault. The steel blocks on both sides measured 100 × 50 × 50 mm³, while the middle steel block measured 150 × 50 × 50 mm³. The thickness of the fault gauge was 1.5 mm. Figure 1A shows the specific sample loading mode. Initially, we placed the assembled samples on the horizontal biaxial press, which had a maximum single-axis load capacity of 150 t and could be driven by either displacement or load control. We first loaded pressure in the Fx direction to the predetermined pressure, which was 0.9 MPa for the gypsum fault gauge experiment and 3 MPa for the kaolinite/calcite mixture experiment. Once the Fx direction reached the predetermined pressure, we controlled the Fy direction using displacement and performed shear experiments at speeds of 0.2, 1, 5, and 25 μm/s, allowing approximately 0.5 mm of slip under each speed condition until the sliding reached a steady state.
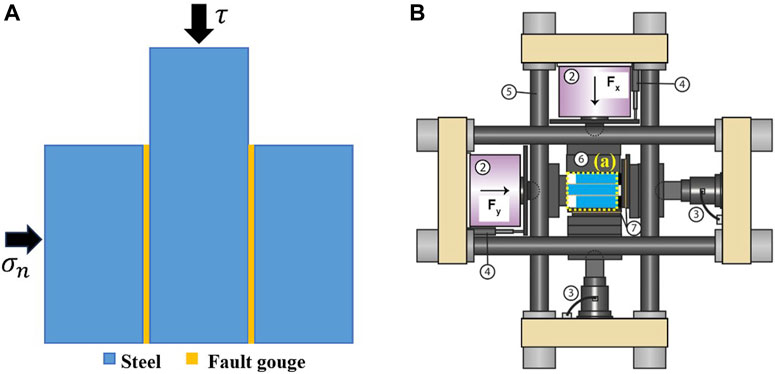
FIGURE 1. (A) Sample assembly diagram; (B) sample assembly and loading schema diagram, (1) Sample assembly; (2) Loading piston, (3) Fixed piston, (4) LVDT, (5) Loading frame, (6) Steel spacers, (7) Slide-bearing steel plates.
After completing the experiment, we determined the values of the friction stability parameters (a-b) based on the rate-state friction constitutive relation. Rate- and state-dependent friction is described using τ and refers to the conditions under which materials either strengthen or weaken with an imposed velocity step (Dieterich, 1979; Ruina, 1983). The Dieterich-Ruina formulation allows us to calculate τ as follows: τ
3 Results and discussion
Figure 2A illustrates the stable sliding behavior of different kaolinite/calcite mixtures under various loading rates (0.2–25 μm/s). As the proportion of kaolinite mass increases, the overall mixed fault gauge’s frictional coefficient gradually decreases. The frictional coefficient exhibits sudden changes during the experimental loading rate switching process, where an increase in loading rate results in a sudden increase in the frictional coefficient (Figure 2B), and a decrease in loading rate leads to a sudden decrease in the friction coefficient (Figure 2C).
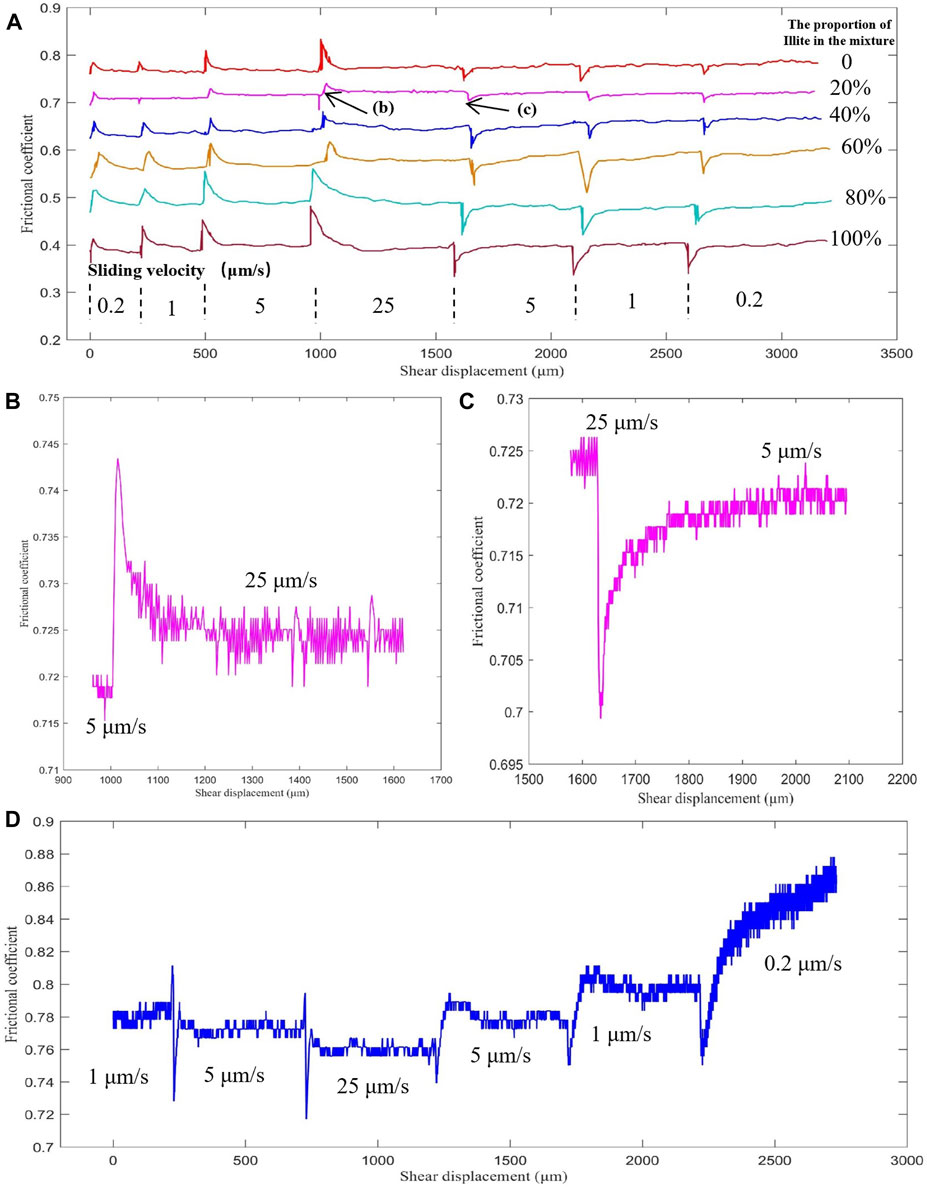
FIGURE 2. (A) Curves of the fault gauge frictional coefficient changing with displacements of different proportions of kaolinite/calcite mixture under the condition of room temperature and 3 MPa effective normal stress, (B) and (C) enlarged images of the black arrow in (A), (D) variation curve of gypsum fault gauge frictional coefficient with displacement under the condition of room temperature and 0.9 MPa effective normal stress.
Previous studies suggested that gypsum exhibits stick-slip behavior under different normal stress conditions at room temperature (Buijze et al., 2021). However, using steel blocks as the surrounding rock and a low effective normal stress of 0.9 MPa in our experimental design is not conducive to stick-slip in the gypsum fault gauge. Therefore, we observe a more stable sliding behavior in the gypsum fault gauge. The accuracy of the gypsum fault gauge’s (a-b) obtained through the steady-statevelocity steptest is superior to the value obtained by fitting the stick-slip data (Dieterich, 1979; Ruina, 1983). Additionally, the friction coefficient exhibits sudden changes during the experimental loading rate switching process, where an increase in loading rate leads to a sudden decrease in the friction coefficient, while a decrease in loading rate causes a sudden increase.
Based on the data, we derived the variation curves of different fault gauge frictional coefficients with loading rate, as shown in Figure 3A. Using the formula
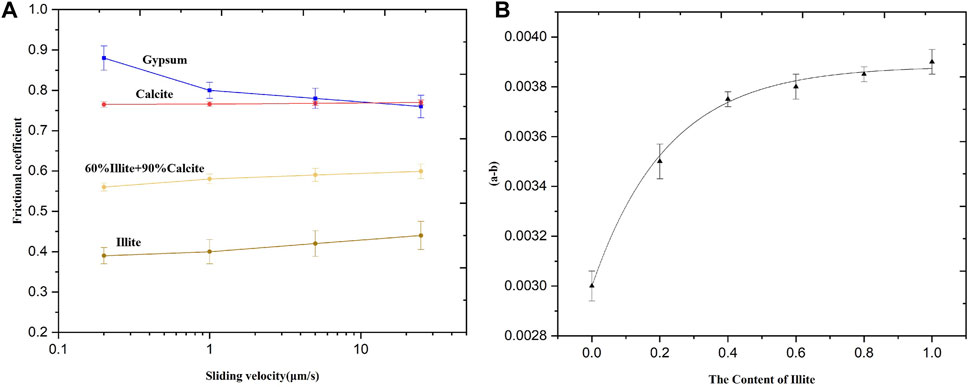
FIGURE 3. (A) Variation of frictional coefficient with loading rate for different fault gauge, (B) variation of kaolinite/calcite mixture fault gauge (A, B) with the mass fraction of kaolinite.
4 Conclusion
By designing a well-designed steady-state velocity step test, we successfully determined the values of (a-b) for both the gypsum fault gauge and the fault gauge consisting of different components of the kaolinite/calcite mixture. For the gypsum fault gauges, the (a-b) values obtained through near steady-statevelocity steptests and those reported in previous studies under similar conditions were negative. However, the (a-b) values obtained in our tests were one order of magnitude lower than those reported in previous studies, indicating that the gypsum fault gauge in our experimental setup exhibits a stronger tendency toward velocity weakening. Our steady-state velocity step tests on the kaolinite/calcite mixture fault gauges revealed that all mixtures, regardless of their kaolinite contents, exhibited positive (a-b) values. Furthermore, we observed a proportional relationship between (a-b) and the kaolinite content. As a result, we established a functional relationship between the (a-b) values of the mixture fault gauge and the mass fraction of kaolinite.
Author contributions
DR: Conceptualization, Funding acquisition, Writing–original draft, Writing–review and editing.
Funding
The author(s) declare financial support was received for the research, authorship, and/or publication of this article. This study is supported by the National Nonprofit Fundamental Research Grant of China, Institute of Geology, China Earthquake Administration (Grant No. IGCEA-22-19), the National Key R&D Program Project, Rock Physical Properties, Friction Parameters, and Mechanical Mechanisms of Fault Zones in the Sichuan Yunnan Region (Project No. 2021YFC3000603) and the National Natural Science Foundation of China (Project No. 42104176).
Conflict of interest
The author declares that the research was conducted in the absence of any commercial or financial relationships that could be construed as a potential conflict of interest.
Publisher’s note
All claims expressed in this article are solely those of the authors and do not necessarily represent those of their affiliated organizations, or those of the publisher, the editors and the reviewers. Any product that may be evaluated in this article, or claim that may be made by its manufacturer, is not guaranteed or endorsed by the publisher.
References
Adjemian, F., and Evesque, P. (2004). Experimental study of stick-slip behaviour. Int. J. Numer. Anal. Methods Geomechanics 28 (6), 501–530. doi:10.1002/nag.350
Albert, I., Tegzes, P., Albert, R., Sample, J. G., Barabási, A. L., Vicsek, T., et al. (2001). Stick-slip fluctuations in granular drag. Phys. Rev. E 64 (3), 031307. doi:10.1103/physreve.64.031307
Anthony, J. L., and Marone, C. (2005). Influence of particle characteristics on granular friction. J. Geophys. Res. Solid Earth 110 (B8). doi:10.1029/2004jb003399
Bedford, J., Faulkner, D., and Lapusta, N. (2022). Fault rock heterogeneity can produce fault weakness and reduce fault stability. Nat. Commun. 13, 326. doi:10.1038/s41467-022-27998-2
Bendavid, O., Cohen, G., and Fineberg, J. (2010). The dynamics of the onset of frictional slip. Science 330 (6001), 211–214. doi:10.1126/science.1194777
Brace, W. F., and Byerlee, J. D. (1966). Stick-slip as a mechanism for earthquakes. Science 153 (3739), 990–992. doi:10.1126/science.153.3739.990
Buijze, L., Guo, Y., Niemeijer, A. R., Ma, S., and Spiers, C. (2021). Effects of heterogeneous gouge segments on the slip behavior of experimental faults at dm scale. Earth Planet. Sci. Lett. 554, 116652. doi:10.1016/j.epsl.2020.116652
Byerlee, J. (1978). “Friction of rocks,” in Rock friction and earthquake prediction (Basel: Birkhäuser), 615–626.
Chlieh, M., Avouac, J. P., Sieh, K., Natawidjaja, D. H., and Galetzka, J. (2008). Heterogeneous coupling of the sumatran megathrust constrained by geodetic and paleogeodetic measurements. J. Geophys. Res. Solid Earth 113. doi:10.1029/2007jb004981
de Meer, S., and Spiers, C. J. (1997). Uniaxial compaction creep of wet gypsum aggregates. J.Geophys. Res. Solid Earth102 (B1) 102, 875–891. doi:10.1029/96jb02481
Dieterich, J. H. (1979). Modeling of rock friction: 1. Experimental results and constitutive equations. J. Geophys. Res. 84 (B5), 2161–2168. doi:10.1029/jb084ib05p02161
Engelder, T. (1978). Aspects of asperity-surface interaction and surface damage of rocks during experimental frictional sliding. pure Appl. Geophys. 116 (4-5), 705–716. doi:10.1007/bf00876533
Freymueller, J. T., Cohen, S. C., and Fletcher, H. J. (2000). Spatial variations in present-day deformation, kenai peninsula, Alaska, and their implications. J. Geophys. Res. 105 (B4), 8079–8101. doi:10.1029/1999jb900388
Frye, K. M., and Marone, C. (2002). The effect of particle dimensionality on granular friction in laboratory shear zones. Geophys. Res. Lett. 29 (19), 22. doi:10.1029/2002gl015709
Géminard, J. C., Losert, W., and Gollub, J. P. (1999). Frictional mechanics of wet granular material. Phys. Rev. E 59 (5), 5881–5890. doi:10.1103/physreve.59.5881
Han, R., Hirose, T., Shimamoto, T., Lee, Y., and Ando, J. i. (2011). Granular nanoparticles lubricate faults during seismic slip. Geology 39 (6), 599–602. doi:10.1130/g31842.1
Härtl, J., and Ooi, J. Y. (2008). Experiments and simulations of direct shear tests: porosity, contact friction and bulk friction. Granul. Matter 10 (4), 263–271. doi:10.1007/s10035-008-0085-3
He, C., Ma, S., and Huang, J. (1998). Transition between stable sliding and stick-slip due to variation in slip rate under variable normal stress condition. Geophys. Res. Lett. 25 (17), 3235–3238. doi:10.1029/98gl02518
He, C., Wang, Z., and Yao, W. (2007). Frictional sliding of gabbro gouge under hydrothermal conditions. Tectonophysics 445 (3-4), 353–362. doi:10.1016/j.tecto.2007.09.008
Hedayat, A., Haeri, H., Hinton, J., Masoumi, H., and Spagnoli, G. (2018). Geophysical signatures of shear-induced damage and frictional processes on rock joints. J. Geophys. Res. Solid Earth 123 (2), 1143–1160. doi:10.1002/2017jb014773
Ikari, M. J., Niemeijer, A. R., and Marone, C. (2015). Experimental investigation of incipient shear failure in foliated rock. J. Struct. Geol. 77, 82–91. doi:10.1016/j.jsg.2015.05.012
Jiang, Y., Wang, G., Kamai, T., and McSaveney, M. J. (2016). Effect of particle size and shear speed on frictional instability in sheared granular materials during large shear displacement. Eng. Geol. 210, 93–102. doi:10.1016/j.enggeo.2016.06.005
Johnson, P. A., Ferdowsi, B., Kaproth, B. M., Scuderi, M., Griffa, M., Carmeliet, J., et al. (2013). Acoustic emission and microslip precursors to stick-slip failure in sheared granular material. Geophys. Res. Lett. 40 (21), 5627–5631. doi:10.1002/2013gl057848
Johnson, T., Wu, F. T., and Scholz, C. H. (1973). Source parameters for stick-slip and for earthquakes. Science 179 (4070), 278–280. doi:10.1126/science.179.4070.278
Johnson, T. L., and Scholz, C. H. (1976). Dynamic properties of stick-slip friction of rock. J. Geophys. Res. 81 (5), 881–888. doi:10.1029/jb081i005p00881
Kaproth, B. M., and Marone, C. (2013). Slow earthquakes, preseismic velocity changes, and the origin of slow frictional stick-slip. Science 341 (6151), 1229–1232. doi:10.1126/science.1239577
Karner, S. L., and Marone, C. (2000). Effects of loading rate and normal stress on stress drop and stick-slip recurrence interval. Geophys. Monograph-American Geophys. Union 120, 187–198. doi:10.1029/GM120p0187
Kato, N., Yamamoto, K., Yamamoto, H., and Hirasawa, T. (1992). Strain-rate effect on frictional strength and the slip nucleation process. Tectonophysics 211 (1-4), 269–282. doi:10.1016/0040-1951(92)90064-d
Lastakowski, H., Géminard, J. C., and Vidal, V. (2015). Granular friction: triggering large events with small vibrations. Sci. Rep. 5, 13455. doi:10.1038/srep13455
Leeman, J., Scuderi, M. M., Marone, C., and Saffer, D. (2015). Stiffness evolution of granular layers and the origin of repetitive, slow, stick-slip frictional sliding. Granul. Matter 17 (4), 447–457. doi:10.1007/s10035-015-0565-1
Lieou, C. K. C., Daub, E. G., Guyer, R. A., Ecke, R. E., Marone, C., and Johnson, P. A. (2017). Simulating stick-slip failure in a sheared granular layer using a physics-based constitutive model. J. Geophys. Res. Solid Earth 122 (1), 295–307. doi:10.1002/2016jb013627
Lockner, D. A., and Okubo, P. G. (1983). Measurements of frictional heating in granite. J. Geophys. Res. Solid Earth 88 (B5), 4313–4320. doi:10.1029/jb088ib05p04313
Lu, Z., and He, C. (2014). Frictional behavior of simulated biotite fault gouge under hydrothermal conditions. Tectonophysics 622, 62–80. doi:10.1016/j.tecto.2014.03.002
Mair, K., Frye, K. M., and Marone, C. (2002). Influence of grain characteristics on the friction of granular shear zones. J. Geophys. Res. Solid Earth 107 (B10), ECV 4-1. doi:10.1029/2001jb000516
Marone, C. (1998). Laboratory-derived friction laws and their application to seismic faulting. Annu. Rev. Earth Planet. Sci. 26 (1), 643–696. doi:10.1146/annurev.earth.26.1.643
Marone, C., Carpenter, B. M., and Schiffer, P. (2008). Transition from rolling to jamming in thin granular layers. Phys. Rev. Lett. 101 (24), 248001. doi:10.1103/physrevlett.101.248001
Moore, D. E., Lockner, D. A., Ma, S., Summers, R., and Byerlee, J. D. (1997). Strengths of serpentinite gouges at elevated temperatures. J. Geophys. Res. Solid Earth 102 (B7), 14787–14801. doi:10.1029/97jb00995
Morrow, C. A., and Byerlee, J. D. (1989). Experimental studies of compaction and dilatancy during frictional sliding on faults containing gouge. J. Struct. Geol. 11 (7), 815–825. doi:10.1016/0191-8141(89)90100-4
Niemeijer, A., Di Toro, G., Griffith, W. A., Bistacchi, A., Smith, S. A., and Nielsen, S. (2012). Inferring earthquake physics and chemistry using an integrated field and laboratory approach. J. Struct. Geol. 39 (0), 2–36. doi:10.1016/j.jsg.2012.02.018
Rivière, J., Lv, Z., Johnson, P. A., and Marone, C. (2018). Evolution of b-value during the seismic cycle: insights from laboratory experiments on simulated faults. Earth Planet. Sci. Lett. 482, 407–413. doi:10.1016/j.epsl.2017.11.036
Ruina, A. (1983). Slip instability and state variable friction laws. J. Geophys. Res. 88 (B12), 10359–10370. doi:10.1029/jb088ib12p10359
Sammis, C. G., and Biegel, R. L. (1989). Fractals, fault-gouge, and friction. Pure Appl. Geophys. 131 (1-2), 255–271. doi:10.1007/bf00874490
Scholz, C. H. (1989). The mechanics of earthquakes and faulting. Geol. Curtain Engl. version 17 (1), 49.
Scholz, C. H., and Engelder, J. T. (1976). The role of asperity indentation and ploughing in rock friction—I: asperity creep and stick-slip. International Journal of Rock Mechanics and Mining Sciences & Geomechanics Abstracts 13 (5), 149–154. doi:10.1016/0148-9062(76)90819-6
Scuderi, M. M., Carpenter, B. M., Johnson, P. A., and Marone, C. (2015). Poromechanics of stick-slip frictional sliding and strength recovery on tectonic faults. J. Geophys. Res. Solid Earth 120 (10), 6895–6912. doi:10.1002/2015jb011983
Shinbrot, T., Kim, N. H., and Thyagu, N. N. (2012). Electrostatic precursors to granular slip events. Proc. Natl. Acad. Sci. 109 (27), 10806–10810. doi:10.1073/pnas.1121596109
Togo, T., Shimamoto, T., Ma, S., and Hirose, T. (2011). High-velocity frictional behavior of Longmenshan fault gouge from Hongkou outcrop and its implications for dynamic weakening of fault during the 2008 Wenchuan earthquake. Earthq. Sci. 24 (3), 267–281. doi:10.1007/s11589-011-0790-6
Tullis, T. E. (1988). Rock friction constitutive behavior from laboratory experiments and its implications for an earthquake prediction field monitoring program. Pure Appl. Geophys. 126 (2-4), 555–588. doi:10.1007/bf00879010
Weeks, J. D., and Tullis, T. E. (1985). Frictional sliding of dolomite: a variation in constitutive behavior. J. Geophys. Res. Solid Earth 90 (B9), 7821–7826. doi:10.1029/jb090ib09p07821
West, M., Sánchez, J. J., and Mcnutt, S. R. (2005). Periodically triggered seismicity at mount wrangell, Alaska, after the sumatra earthquake. Science 308 (5725), 1144–1146. doi:10.1126/science.1112462
Yao, L., Ma, S., Niemeijer, A. R., Shimamoto, T., and Platt, J. D. (2016). Is frictional heating needed to cause dramatic weakening of nanoparticle gouge during seismic slip? Insights from friction experiments with variable thermal evolutions. Geophys. Res. Lett. 43 (13), 6852–6860. doi:10.1002/2016gl069053
Keywords: friction stabilities, gypsum, kaolinite/calcite mixture, high pressure, fault gauges
Citation: Ren D (2024) Friction stabilities of gypsum and kaolinite/calcite mixture fault gauges under high pressure. Front. Earth Sci. 11:1346880. doi: 10.3389/feart.2023.1346880
Received: 07 December 2023; Accepted: 27 December 2023;
Published: 09 January 2024.
Edited by:
Lidong Dai, Chinese Academy of Sciences, ChinaReviewed by:
Zhankun Liu, Central South University, ChinaKai Zheng, Gannan Normal University, China
Kai Luo, Yunnan University, China
Copyright © 2024 Ren. This is an open-access article distributed under the terms of the Creative Commons Attribution License (CC BY). The use, distribution or reproduction in other forums is permitted, provided the original author(s) and the copyright owner(s) are credited and that the original publication in this journal is cited, in accordance with accepted academic practice. No use, distribution or reproduction is permitted which does not comply with these terms.
*Correspondence: Dongsheng Ren, ZG9uZ3NoZW5ncmVuQGZveG1haWwuY29t