- 1Institute of Geotechnical Engineering, Nanjing Tech University, Nanjing, China
- 2Shijiazhuang Institute of Railway Technology, Shijiazhuang, China
- 3Application Technology R&D Center of Bridge and Tunnel Intelligent Construction of Hebei Colleges, Shijiazhuang, China
The tunnel construction by the drill-and-blast method can have a significant impact on the adjacent buildings, especially the historical buildings with poor structural performances. Based on a special explosive case of Nanjing Metro Line 4, an in-situ blasting test for vibration safety evaluation on the Nanjing Drum Tower was carried out. According to the site geological investigation, the location of the blasting test was selected near the planned tunnel. 6 blasting was carried out in terms of sing-hole blasting and millisecond-delay blasting. The attenuation of the ground vibration and the structural vibration responses of the historical Drum Tower were analyzed. The test results also showed that the Nanjing Drum Tower was not damaged by this blasting test. For single-hole blasting tests, the peak particle velocity increased with the increasing of the explosive charge, but decreased remarkably when using the millisecond-delay blasting technology. The horizontal and vertical ground vibration attenuation patterns were consistent, which can be described by the Sadowski formula. The ground vibration response was dominated by the horizontal vibration, and the velocity amplification factor in the case of the single-hole test with a charge quantity of 400 g reached 2.4, which was observed the most remarkable amplification effect.
1 Introduction
With the rapid development of urban rail transit, controlled blasting technique is widely used in geotechnical engineering construction. Many planned tunnels will be constructed inevitably cross the urban underground space. Meanwhile, the seismic effect induced by blasting has great impact on the surrounding environment of the blasting area. Therefore, it is necessary to strictly control the vibration of the ground surface and structure caused by tunnel blasting. Some existing research show that when the blasting vibration intensity is rather high, the blasting vibration frequency close to the natural frequency of buildings can cause resonance effect, while high-frequency blasting vibration usually leads to the local stress concentration of the rigid structure, and consequently global or partial damage at different levels is expected in the nearby structures (Ma et al., 2000; Singh, 2002; Wu and Hao, 2005; Singh and Roy, 2010; Yu et al., 2014; Li and Li, 2018; Verma et al., 2018; Bao et al., 2022; Gao et al., 2014).
The in situ blasting test is considered as an important tool to study the effect of blasting induced vibrations on the nearby structures. The measured data can also provide a reference for further research. Based on the field observations, Dowding (Dowding, 1992) concluded that blasting earthquakes have no effect on the structure of buildings located 200–300 feet away. Based on the measured data of two-story ordinary houses and the observation of cracks in the structure body when long-term blasting vibration is applied, Lou et al. (Lou et al., 2001) gave the allowable vibration velocity standard of 2 cm/s which is suitable for common residential structures in this area. Xie et al. (Xie et al., 2007) tested the blasting vibration response of the national heritage ancient stone tower and concluded that the acceleration and the velocity of the ancient tower top can be used as the evaluation criteria for the safety of the control tower structure. Through on-site monitoring of the blasting-induced stress waves and the vibration responses of the buildings with 3–4 storeys, Lin et al. (Lin et al., 2010) obtained the response characteristics of each storey under blasting vibration and found that most of the vibration energy of each floor was concentrated in the 6–25 Hz band. Ma et al. (Ma et al., 2013) tested the blasting vibration response of an ancient bridge, and compared the specifications at different countries to obtain the controlling standard of bridge vibration speed at 2 mm/s. The dynamic response of the building subject to nearby blasting is related to the dynamic characteristics of the structure itself and the ground condition (Wei, 2010). At present, the understanding of the dynamic characteristics of ancient buildings in the blasting construction is still inadequate, meanwhile the existing codes require more control over the safety of cultural buildings, and blasting design should be performed with particular caution. Very few investigations are available in the literature on the ground vibration characteristics caused by millisecond-delay blasting and the induced structural response of the historical wooden-frame structure.
Based on the planned tunnel blasting in the Nanjing Metro Line 4, an in situ blasting test to study the impact on the historical Drum Tower is carried out. By monitoring the peak vibration velocities at the blasting sites and at the Drum Tower, the correlation between the ground vibration attenuation behavior is discussed. According to the vibration characteristics at the monitoring points, the vibration response of the Drum Tower under different blasting intensities is analyzed. Finally, the peak vibration velocity of the historical Drum Tower is evaluated and the controlling blasting parameters are suggested in the planned tunnel blasting.
2 Project background
The planned Metro Line 4 starts from the Pearl Spring park, passing through the main city, and ends in Xianlin East in Nanjing. The line of concern in this study is about 660 m long, with the Gulou Station on the north side of Gulou Park. The existing underground subway station of the Metro Line 1 is on the east side of the historical Drum Tower in the middle of the park as shown in Figure 1.
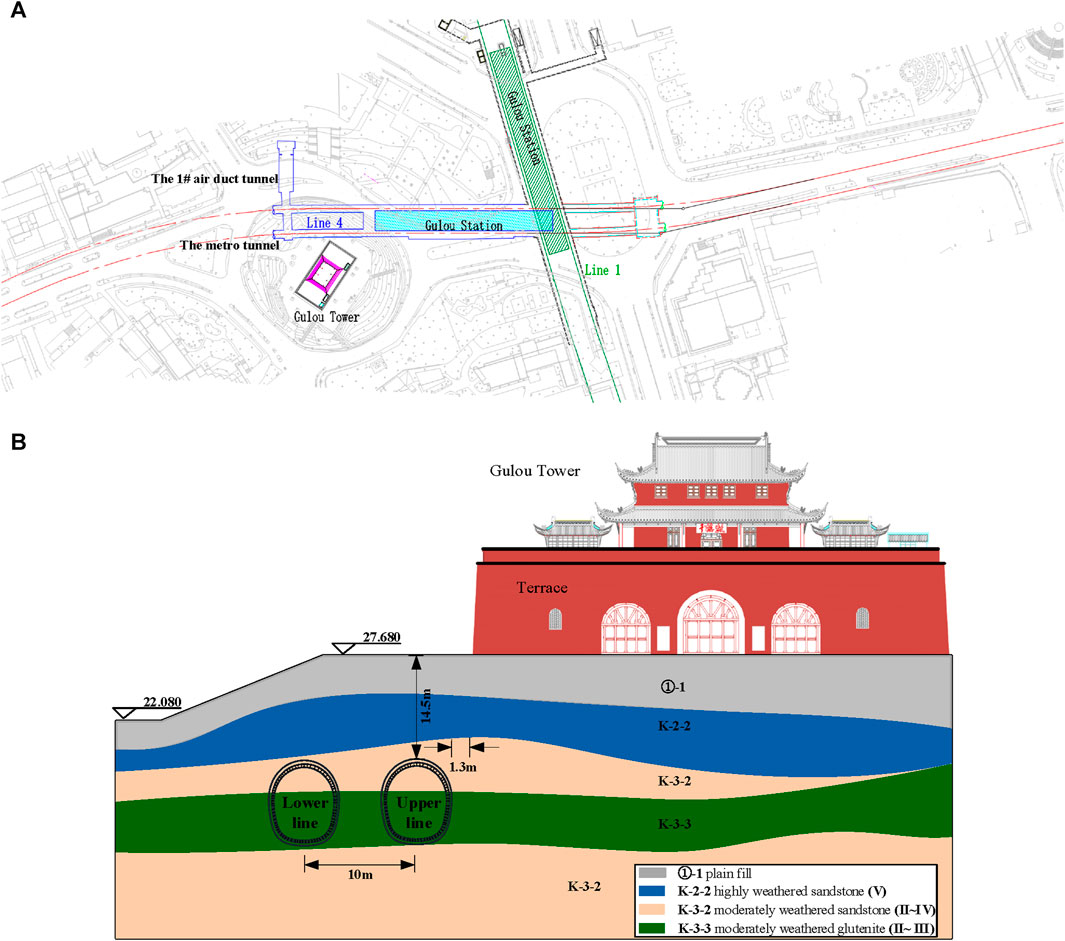
FIGURE 1. Location of the Drum Tower along Metro Line 4 in Nanjing, China: (A) Plan view of the tunnels and the Drum Tower, (B) Sectional view of the tunnels and the Drum Tower.
The Drum Tower was built in 1,382 in the Ming Dynasty, which is a multi-story wooden-frame structure based on a masonry basement. The masonry basement with a height of 8.915 m has three-arch passages, two chambers, and stairs leading to the top. The wooden-frame tower with a height of 13.315 m has two floors and a roof decorated with wood carvings, and the weight of the tower roof is sustained by wood pillars and masonry walls. Experiencing numerous natural disasters and man-made disturbances, the structure of historical Drum Tower is significantly damaged as shown in Figure 2. The drill and blast method is to be used in the vicinity of Drum Tower, including the interval tunnel, the wind tunnel, and the three-part arch underground structure of the Gulou subway station for Metro Line 4. In the proposed scheme, the interval tunnel is only about 1.3 m from the basement of drum tower, and the vertical distance is 14.5 m 1# the wind tunnel is about 19.8 m from the basement of drum tower, and the vertical distance is 13.5 m. The three-way arch is about 18.1 m, and the vertical distance is 11 m. The primary purpose of the blasting test in this study is to evaluate whether it is feasible to control this adverse structural vibration on the timber framed tower by using the millisecond delay blasting.
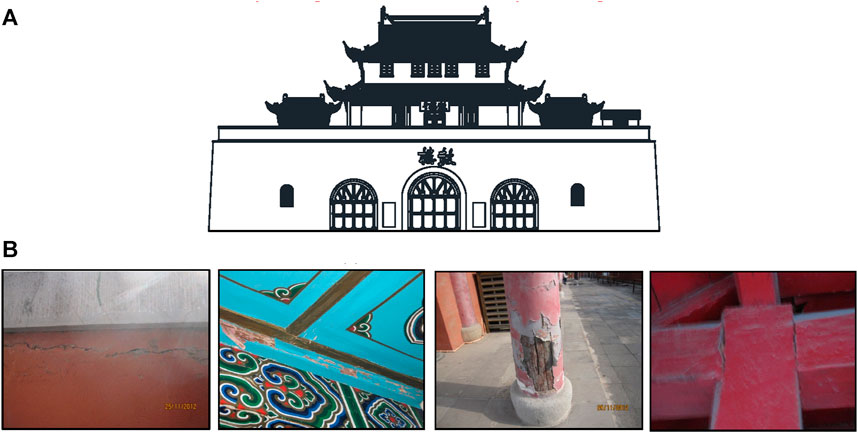
FIGURE 2. Overview of the historical Drum Tower. (A) The Drum Tower. (B) Existing damages in the Drum Tower.
3 In situ blasting test program
3.1 Test site and monitoring sensor layout
In the blasting test site, the horizontal distance between the tunnels and the Drum Tower is only about 1.3 m. In order to avoid the impact of direct blasting on the safety of historical Drum Tower, the experiment was carried out to simulate the blasting event in the surrounding environment of the Drum Tower.
The site of the blasting test should be close to the test structure and should also be level without other structures. The monitoring points should be installed within the site from the blasting source vicinity to the test structure, to facilitate to evaluate the impact on the historical structure (Wang et al., 2017). According to the actual situation of the site and the exploration data, the underground space of the 1 # air duct tunnel can be used as the simulation blasting point. The geological condition of the selected tunnel section close to the historical tower, is similar to that of the Drum Tower. Table 1 shows the geological conditions of the site and the classification of the surrounding rock. The regional site constitutes an uneven working face, which is in favor of the propagation of the low-frequency waves induced by the blasting. Gui et al. (Gui et al., 2017) pointed out that the existence of rock-soil interface usually depresses the peak ground velocity more than that of simple uniform layers, which also affects the peak velocity of the ground near the drum tower.
In order to estimate the impact of blasting induced vibration on the Drum Tower when the tunnel excavates through the Drum Tower, the relative position between the testing blasting site and each monitoring point on the ground surface of the 1 # air duct tunnel is consistent with that between the planned blasting site and the Drum Tower. The simulated blasting point at the 1 # air duct tunnel locates at the same site as the planned explosion point, but with a distance away from the planned explosion point to avoid any damage to the historical drum tower induced by the blasting test. The shortest distance between the simulated blasting point and the assumed Drum Tower is 1.3 m. Six monitoring points are installed along the ground surface between the 1 # air duct tunnel and the assumed Drum Tower, indicated by F as shown in Figure 3.
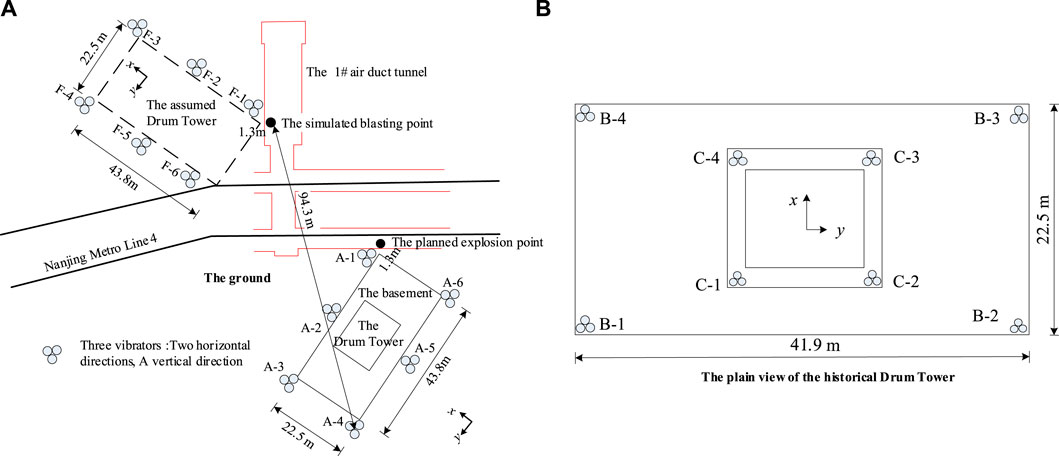
FIGURE 3. Layout of monitoring points in the blasting test. (A) The simulated blasting location and the monitoring points layout at the ground surface. (B) The monitoring point layout at the historical Drum Tower (plain view).
On the surface of the basement and the four corners of the Drum Tower, four monitoring points are arranged in each monitoring surface, which are distinguished by B and C, respectively. The total number of monitoring points is 20, and each monitoring point is used to capture the vibration velocity in the two horizontal directions and one vertical direction, which are shown in Figure 3.
3.2 Test blasting program and conditions
The blasting test was detonated by two ways (i.e., single-hole blasting and millisecond delay blasting) and six blasting cases were conducted in this study. In the case of single-hole blasting, it is recharged for another hole after the completion of previous blasting hole. According to the sequence of the hole number, the charge weights of single-hole blasting are 100 g, 200 g, 300 g, 400 g, and 500 g, respectively. The six-hole millisecond delay blasting is used in multi-hole blasting with millisecond detonation between the 6 holes. The charge of 200 g is used in each blasting hole (from hole no. 6–10) and the last hole (hole no. 11) is charged with 100 g, with the time delay between adjacent holes of 50 milliseconds.
According to the blasting intensity and the number of monitoring points arranged, a total of 120 groups of data was collected in the blasting vibration test. Among them, 84 sets of valid data are 6 monitoring points (F1 ∼ F6) on the airway surface, 4 monitoring points (A-1, A-3, A-4, A-6) on the surface of the Drum Tower and 4 monitoring points (C-1, C-2, C-3, C-4) on the Drum Tower.
4 Test result analysis
4.1 Analysis of measured results
Figures 4, 5A show the peak velocities at each monitoring point of the 1# air duct tunnel and drum tower respectively. As can be seen from Figures 5B, the area where the historical Drum Tower is located is still under the influence of simulated blasting vibration. The peak vibration velocities of the monument buildings in Drum Tower are all less than 1 mm/s, satisfying the requirements of “Safety Code for Blasting” GB6722-2014 (Republic of China industry standard writing group, 2002) for the allowable vibration velocity of ancient building. This simulation blasting test has little impact on the Drum Tower.
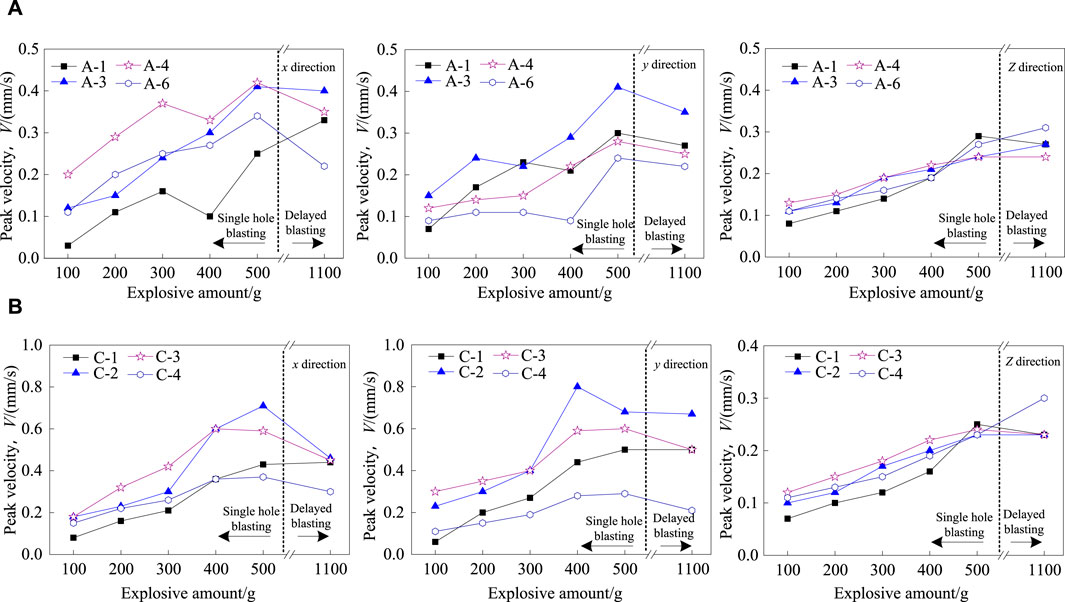
FIGURE 5. Peak particle velocities on the ground and at the structure of the Drum Tower. (A) along A side. (B) along C side.
In the single-hole blasting, the peak vibrational velocities in the x, y and z directions of the monitoring points on the surface and the historical tower generally increase with the increase of charge quantity in the range of 100–500 g. However, as can be seen, the peak velocity response for the test using millisecond delay blasting technology is significantly lower, as compared with that using the single-hole blasting. For a 6-hole millisecond delay blasting with a charge of 1,100 g, the vibration velocity at each monitoring point is lower than that measured at a single-hole charge of 500 g. And the peak vibration velocity at the monitoring point is greater than the velocities of 100 g and 200 g for the single hole charge, whereas more close to those of 300 g–400 g charge. This can be traced back to the stress wave superposition effect induced by the millisecond difference detonation time between the blasting holes.
4.2 Correlation of ground vibration attenuation of drum tower and 1# air duct tunnel
Regressive analysis is usually conducted to study the propagation law of blasting seismic waves in rock mass based on field measured data, to determine the constant K and α values in the Sadowski formula and obtain the attenuation law of the vibration wave in the rock mass (Wu et al., 2003; Yan et al., 2013), which can be expressed as:
Where: V is the maximum velocity of blasting vibrating particle; Q is the amount of explosives (kg), R is the burst source distance (m), ρ is defined as the proportional distance; K and α are the coefficients and attenuation indexes related to the topography and geological conditions between the blasting point and the monitoring point.
According to Formula 1, based on the monitoring data at the 1# air duct tunnel and the Drum Tower in six working conditions, the relationship between the proportional distance and the peak vibration velocity is established. The attenuation law of vibration in the three directions of x, y, and z is shown in Figure 6. As can be seen from this figure, when the proportional distances are close, the peak vibration velocities of the surface of the wind tunnel and the drum tower of the cultural relic basically coincide with each other, and the decay trend of the two ground surfaces is continuous. The surface vibration characteristics of the simulated blasting area can generally reflect the surface vibration of the actual blasting area characteristic. After fitting, the decay laws of x and y are basically the same, K = 86.9, α = 1.72; z direction, K = 36.6, α = 1.62.
4.3 Vibration response of the drum tower under different blasting intensities
Figure 5 shows the ratio of horizontal and vertical peak vibration velocities at the four corners of Drum Tower and ground. It can be seen that the horizontal vibration of Drum Tower Stele is obviously magnified compared to the ground surface, while simple attenuation can be reflected for the vertical vibration. The blasting vibration plays a dominant role in the horizontal direction of the drum tower, which is consistent with the general architectural structure (Verma et al., 2018). As compared with the velocity amplification factors under different blasting intensities, it is found that the maximum peak vibration velocity at the ground surface is the most significantly amplified to 2.4 times at the case of the charge quantity of 400 g (Figure 7).
Based on the information data of structural monitoring provided by authoritative organizations, natural frequency of vibration of the Nanjing Drum-tower monument floor is listed in Table 2. Due to space limitations, Figure 8 only compares the Fourier spectra of the velocity time histories for C-1-Y at 300 g, 400 g and 500 g (the monitoring point C-1 at the y-direction). It can be seen that with the charge quantity of 400 g, the vibration response of the structure is close to the first-order natural frequency of Drum Tower, and the amplitude is significant. The maximum frequency of Fourier spectrum is 1.34 Hz. As the charge quantity reaches 500 g, the vibration response of the structure is closer to the second order natural frequency of the Drum Tower, and the maximum Fourier spectral frequency is 2.32 Hz. When the charge is 300 g, the vibration response of the structure deviates from the first and second order natural frequencies of the historical Drum Tower. The frequency of the Fourier spectrum with respect to the maximum amplitude is 1.83 Hz. Geometrical spreading, material damping, and apparent attenuation are the three major causes of the attenuation of peak ground vibration, and especially the domain frequency. The domain frequency at the monitoring point is determined not only by the blasting source, but also by the propagation medium. In this test, the blasting site is geological multi layers. It is believed that multiple soil-rock layers could affect stress wave propagation significantly, like high frequency filtering and low frequency amplification effect at the soil mass.
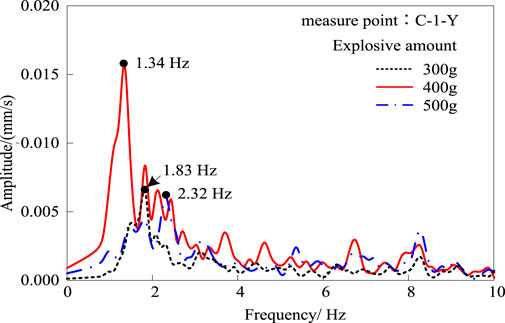
FIGURE 8. Fourier amplitudes of particle velocity time-histories at the point C-1-Y under different blasting intensities.
4.4 Prediction of vibration characteristics of drum tower in the planned blasting
Based on the above analysis, from a more realistic point of view, the testing results may be used to predict the peak vibration velocity of the Drum Tower subjected to the planned tunnel blasting. The predicted values of peak vibration velocities at the various monitoring points can be scaled and listed in Table 3.
For a linear system, the dynamic response is proportional to the input vibration intensity (Jordan et al., 2009). Here, it is assumed that the Drum Tower structure is still a linear system under all operating conditions, and its amplification effect is assumed unchanged with respect to the testing conditions. From Table 3, it can be seen that the peak vibration velocities at each monitoring point at the Drum Tower are less than 3 mm/s when the charge quantity is below 300 g. The peak vibration velocity during blasting is also basically lower than 3 mm/s. It can be seen that the millisecond delay blasting and single-hole blasting with the charge quantity below 300 g have minor impact on the safety of the Drum Tower. It is noted that the peak vibration velocity approaches 5 mm/s at the single-hole blasting case with the charge quantity of 400 g.
5 Conclusion
It can be seen from the monitoring data, the regulations of “blasting safety regulations” and the spectral characteristics of the vibration response of the Drum Tower that the testing blasting did not cause any further damage to the historical Drum Tower, which can be used as the basis for the selection of actual blasting charge and provide the basic information for the subsequent systematic study on the vibration characteristics of the Drum Tower. The vibration characteristics of the blasting ground surface and the Drum Tower can be concluded as follows:
(1) Comparing the different charges and blasting methods, the surface peak vibration velocity of 6-hole millisecond delay blasting (1,100 g in total) is close to the result of the single-hole blasting with the charge quantities of 300 g–400 g, and can be more effectively used to decrease the peak ground velocity.
(2) When the blasting central distances are similar, the peak vibration velocity of the testing blasting area is close to the ground surface of the Drum Tower, and the surface attenuation law of the blasting vibration is continuous. According to the Sadowitz empirical formula, the fitting coefficients α and K were determined, at the horizontal direction, K = 86.9, α = 1.72; and at the vertical direction, K = 36.6, α = 1.62.
(3) The horizontal vibration of the historical Drum Tower is amplified and the vertical vibration shows a rapid decay trend. Compared with the spectral characteristics of the structure velocity response at the charge quantities of 300 g, 400 g, and 500 g, a significant amplitude around the first order natural frequency of Drum Tower is observed at the charge of 400 g, in which amplification effect is the most significant, up to 2.4 times.
(4) According to the prediction of the peak vibration velocity at the Drum Tower, within the allowable range of blasting-induced vibration, the use of a single hole explosive amount of 300 g. The millisecond delay blasting is expected to have minor effect on the safety of the historical Drum Tower.
Due to the complexity of the blasting-induced environmental vibration problem, it is call for advanced data digging technology to further identify the key factors determining the millisecond-delay blasting-induced vibrations of the historical drum tower, e.g., ANN Deep Learning. Furthermore, it is essential to perform further 3D numerical analyses to accurately describe the vibration and the induced stress response of the historical structures during the blasting performed in the planned tunnel.
Data availability statement
The original contributions presented in the study are included in the article/Supplementary material, further inquiries can be directed to the corresponding author.
Author contributions
YR: Conceptualization, Funding acquisition, Resources, Writing–review and editing, Formal Analysis, Investigation, Software, Writing–original draft. SX: Writing–original draft, Writing–review and editing, Conceptualization, Methodology, Resources, Supervision. YY: Writing–review and editing, Data curation, Validation. TW: Investigation, Software, Writing–review and editing. HW: Conceptualization, Methodology, Writing–review and editing. KZ: Writing–review and editing, Conceptualization, Formal Analysis, Funding acquisition, Investigation, Resources, Writing–original draft.
Funding
The author(s) declare that no financial support was received for the research, authorship, and/or publication of this article.
Acknowledgments
The authors are truly grateful to the High Performance Computing Center of Nanjing Tech University for supporting the computational resources.
Conflict of interest
The authors declare that the research was conducted in the absence of any commercial or financial relationships that could be construed as a potential conflict of interest.
Publisher’s note
All claims expressed in this article are solely those of the authors and do not necessarily represent those of their affiliated organizations, or those of the publisher, the editors and the reviewers. Any product that may be evaluated in this article, or claim that may be made by its manufacturer, is not guaranteed or endorsed by the publisher.
References
Bao, X., Li, S. T., Liu, J. B., and Chen, Y. (2022). 3D multiscale analysis method for explosion problems based on the substructure of the explosion source. Eng. Struct. 252, 113633. doi:10.1016/j.engstruct.2021.113633
Dowding, C. H. (1992). Suggested method for blast vibration monitoring. Int. J. Rock Mech. Min. Sci. Geomechanics Abstr. 29 (2), 145–156. doi:10.1016/0148-9062(92)92124-u
Gao, H., Sun, J., Stuedlein, A. W., Li, S., Wang, Z., and Liu, L. (2024). Flowability of saturated sands under cyclic loading and the viscous fluid flow failure criterion for liquefaction triggering. J. Geotech. Geoenviron. Eng. 150(1), 04023130. doi:10.1061/JGGEFK.GTENG-11872
Gui, Y. L., Zhao, Z. Y., Zhou, H. Y., Goh, A., and Jayasinghe, L. (2017). Numerical simulation of rock blasting induced free field vibration. Procedia Eng. 191, 451–457. doi:10.1016/j.proeng.2017.05.203
Jordan, J. W., Sutcliffe, D. J., and Mullard, J. A. (2009). Blast vibration effects on historical buildings. Aust. J. Struct. Eng. 10 (1), 75–84. doi:10.1080/13287982.2009.11465034
Li, C. J., and Li, X. B. (2018). Influence of wavelength-to-tunnel-diameter ratio on dynamic response of underground tunnels subjected to blasting loads. Int. J. Rock Mech. Min. Sci. 112, 323–338. doi:10.1016/j.ijrmms.2018.10.029
Lin, J., Lin, C. M., and Lin, L. Q. (2010). Research on response characteristics of structure under dynamic blasting load. J. Vib. shock 3, 48–51.
Lou, J. W., Long, Y., Xu, Q. J., and Ji, R. M. (2001). Analysis of damage to low-rise houses under blasting seismic wave. Jounal PLA Univ. Sci. Technol. 2 (2), 21–25.
Ma, G. W., Hao, H., and Zhou, Y. X. (2000). Assessment of structure damage to blasting induced ground motions. Eng. Struct. 22 (10), 1378–1389. doi:10.1016/s0141-0296(99)00072-3
Ma, J., Luan, L. F., Li, X. H., Wang, J. G., and Li, Q. H. (2013). Monitoring and analysis of historic building vibration influenced by blasting in complex urban environment. Appl. Mech. Mater. 423-426, 1558–1562. doi:10.4028/www.scientific.net/amm.423-426.1558
Republic of China industry standard writing group. (2002). People’s Republic of China industry standard writing group. GB6722-2014. Blasting safety regulations. Beijing: China Building Industry Press (in Chinese).
Singh, P. K. (2002). Blast vibration damage to underground coal mines from adjacent open-pit blasting. Int. J. Rock Mech. Min. Sci. 39 (8), 959–973. doi:10.1016/s1365-1609(02)00098-9
Singh, P. K., and Roy, M. P. (2010). Damage to surface structures due to blast vibration. Int. J. Rock Mech. Min. Sci. 47 (6), 949–961. doi:10.1016/j.ijrmms.2010.06.010
Verma, H. K., Samadhiya, N. K., Singh, M., Goel, R., and Singh, P. (2018). Blast induced rock mass damage around tunnels. Tunn. Undergr. Space Technol. 71, 149–158. doi:10.1016/j.tust.2017.08.019
Wang, M. Y., Li, J., Qiu, Y. Y., and Chen, W. (2017). A calculation method for irreversible deformation region radius under large-scale underground explosion. Explos. shock waves 37 (4), 685–691. doi:10.11883/1001-1455(2017)04-0685-07
Wei, H. X. (2010). Study on dynamic response and safety criterion of buildings to blasting vibration waves. Qingdao, China: Shandong University of Science and Technology.
Wu, C., Hao, H., Lu, Y., and Zhou, Y. X. (2003). Characteristics of stress waves recorded in small-scale field blast tests on a layered rock–soil site. Geotechnique 53 (6), 587–599. doi:10.1680/geot.2003.53.6.587
Wu, C. Q., and Hao, H. (2005). Numerical study of characteristics of underground blast induced surface ground motion and their effect on above-ground structures. Part I. Ground motion characteristics. Soil Dyn. Earthq. Eng. 25 (1), 27–38. doi:10.1016/j.soildyn.2004.08.001
Xie, Z. Z., Yao, D. P., and Zhang, Y. F. (2007). Research on blasting vibration measuring and analysis methods for historical buildings. Blasting 24 (4), 77–81.
Yan, W. M., Tham, L. G., and Yuen, K. V. (2013). Reliability of empirical relation on the attenuation of blast-induced vibrations. Int. J. Rock Mech. Min. Sci. 59 (5), 160–165. doi:10.1016/j.ijrmms.2012.12.005
Keywords: in-situ blasting test, drum tower, ground vibration attenuation, peak particle velocity, structural safety
Citation: Ren Y, Xi S, Yang Y, Wan T, Wang H and Zhao K (2024) Structural vibration characteristics of the historical building in a nearby blasting test. Front. Earth Sci. 11:1304354. doi: 10.3389/feart.2023.1304354
Received: 03 October 2023; Accepted: 06 November 2023;
Published: 01 February 2024.
Edited by:
Jianhua Yang, Nanchang University, ChinaReviewed by:
Yonggang Gou, Hohai University, ChinaQionglin Li, Southwest Jiaotong University, China
Ting Huang, Hohai University, China
Copyright © 2024 Ren, Xi, Yang, Wan, Wang and Zhao. This is an open-access article distributed under the terms of the Creative Commons Attribution License (CC BY). The use, distribution or reproduction in other forums is permitted, provided the original author(s) and the copyright owner(s) are credited and that the original publication in this journal is cited, in accordance with accepted academic practice. No use, distribution or reproduction is permitted which does not comply with these terms.
*Correspondence: Shujuan Xi, eGlhb3N1eGkzNkAxNjMuY29t