- 1School of Geoscience and Technology, Southwest Petroleum University, Chengdu, China
- 2Sichuan College of Architectural Technology, Chengdu, China
In-situ stress plays a crucial role in governing various parameters such as the distribution of oil and gas accumulation zones, the fracture pattern of reservoirs, formation fracture pressure, and collapse pressure. Understanding the distribution characteristics of current in situ stress of reservoirs has significant implications for exploration and development of oil and gas. This paper focuses on the characterization methods for current in situ stress of oil and gas reservoirs, discussing the research progress in testing methods, computational approaches, numerical simulations, and seismic prediction methods. The results indicate that the testing method including the on-site testing method and the laboratory testing method offer the relatively high accuracy, but this method only provides point-specific magnitude and direction of current in situ stress. The Computational approaches can obtain continuous profiles of current in situ stress along individual wells. After using the testing method for calibration, we can obtain relatively accurate calculation results. The numerical method can predict current in situ stress over large areas, but it requires rigorous model setup, boundary definition, and parameter selection. The seismic prediction method also can predict broad distribution of current in situ stress, but this method is influenced by many factors and we had better apply this method in conjunction with other methods. In the future, engineers and researchers should innovate testing technologies and instruments, and establish models and processes for joint use of multiple methods, and explore the development of novel current in situ stress prediction models based on artificial intelligence and big data.
1 Introduction
In-situ stress primarily results from factors such as the gravity of overlying rock layers, formation pressure (pore pressure), tectonic stress, and thermal effects (Banerjee and Chatterjee, 2021; Bouchachi et al., 2022). In-situ stress is typically represented by a vertical principal stress (Sv) and two horizontal principal stresses (SHmax and Shmin) (Gowida et al., 2022). Current in situ stress refers to the current stress state of rock formations in comparison to past stress conditions. Current in situ stress plays a crucial role in the analysis of oil and gas migration and accumulation patterns, engineering design of well drilling, hydraulic fracturing design of reservoirs, and the location of exploration and development well (Radwan et al., 2021; Wu et al., 2022a). Clarifying the distribution characteristics of current in situ stress is an important aspect of oil and gas exploration and development (Baouche et al., 2023). In this context, studying only localized, single-well, and stratified micro-scale stress distribution and stress field states are insufficient. It's essential to conduct macroscopic, regional research on current in situ stress.
At home and abroad, the exploration and development depth of oil and gas reservoirs generally ranges from 1,000 to 10,000 m, and the depth are deeper than the depth of current in situ stress research in fields like mining, water resources, and tunnel engineering. Methods for characterizing current in situ stress of oil and gas reservoirs differ substantially from those used for characterizing current in situ stress of shallow rock layers. Researchers have proposed numerous approaches to characterize current in situ stress of oil and gas reservoirs (Hikweon and See, 2018; Fang et al., 2022; Garavand and Hadavimoghaddam, 2022; Zhou et al., 2023).
2 Testing methods
The testing method is one of the earliest techniques used to characterize current in situ stress of reservoirs (Yin et al., 2018). Compared to other characterization methods, this approach provides the most direct and accurate information regarding the direction and magnitude of current in situ stress (Aadnoy et al., 2013; Kim et al., 2017; Subrahmanyam, 2019; Krietsch et al., 2022). According to Table 1, the testing method can be divided into two categorys: the on-site testing method for well drilling and the laboratory testing method for rock core samples (Kruszewski et al., 2022).
There are many on-site testing methods for well drilling. Hydraulic fracturing is the most commonly used method for measuring current in situ stress in oil and gas reservoirs (Yang et al., 2021). This technique is characterized by its simplicity, broad applicability, and independence from the elastic parameters of the rock. It is also the most reliable testing method for current in situ stress of reservoirs (Xiong and Hampton, 2021). Building upon hydraulic fracturing, additional methods have been proposed, including Micro-Hydraulic Fractures (M-HF), Sleeve Fracture (SF), Hydraulic Test of Pre-existing Fractures (HTPF), and Diagnostic Fracture Injection Test (DFIT). These methods extend the scope of hydraulic fracturing. However, the use of hydraulic fracturing alone can only determine the magnitude of current in situ stress and can’t determine the direction. Usually, micro-seismic monitoring is combined with hydraulic fracturing to define the direction of current in situ stress (Agharazi, 2016; Li et al., 2019; Li et al., 2020; Li et al., 2022a; Li et al., 2023a).
The analysis methods of borehole collapse, induced wellbore cracks, and borehole deformation can determine the direction of current in situ stress. However, these methods are significantly influenced by factors such as formation stress state, rock mechanics properties, drilling parameters, and testing instruments, leading to considerable result variability. Additionally, these methods struggle to determine the magnitude of in situ stress (Gao, 2021; Wang et al., 2021; Ye et al., 2023). Core samples can measure both the magnitude and direction of current in situ stress. Acquiring high-quality oriented core samples is a fundamental and essential requirement for conducting testing work. Alternatively, other methods can be employed to determine the orientation of core samples, such as measuring the geomagnetic information carried by the core. However, the precision of these tests can impact the accuracy of subsequent experimental result.
Laboratory testing methods for rock cores can effectively determine the value of current in situ stress. Core based current in situ stress testing methods include Acoustic Emission method (AE), Anelastic Strain Recovery (ASR), Differential Strain Curve Analysis (DSCA), Differential Wave Velocity Analysis (DWVA), Circumferential Velocity Anisotropy (CVA), Drilling Induced Fracture in Core (DIFC)/Core Discing (CD), Overcoring of Archived Core (OCAC), Petrographic Examination of Micro-cracks and Axial Point Load Test.
The Acoustic Emission Method is related to the stress state of rock and the generation and propagation of elastic waves. Therefore, rocks with better elastic properties will exhibit a noticeable Kaiser effect, resulting in relatively accurate test of current in situ stress (Goodfellow and Young, 2014; Wu et al., 2022b). However, the Kaiser effect may not be prominent for porous and loose rocks, leading to relatively low accuracy of test result (Holcomb, 2013). Additionally, determining the Kaiser point during the experimental process can be challenging. To enhance the accuracy of current in situ stress test, the acoustic emission test can be complemented with other testing methods.
The Stress Relief Method based on viscoelastic strain recovery demonstrates high effectiveness and cost-effectiveness. However, the reliability of result from individual test is diminished due to numerous influencing factors (Sugimoto et al., 2021). In practice, supplementary prior information can be used to validate the accuracy of this method. When combined with the Differential Strain Curve Analysis method, better testing results can be achieved, and the influence of core placement time on test results can be minimized (Sanada et al., 2013).
The Differential Wave Velocity Analysis method and the Circular Wave Velocity Anisotropy Analysis method can determine the direction of current in situ stress based on the speed of acoustic wave in core samples (Ren and Hudson, 1987). These two methods are significantly affected by rock properties, rock structures, and micro-fractures, resulting in relatively lower accuracy when used individually to determine the direction of current in situ stress.
Analyzing cylindrical cores or core-induced cracks to determine the direction of current in situ stress will yield highly accurate results. However, the occurrence of cylindrical cores and core-induced cracks is extremely rare, and orientation can be challenging, limiting the applicability of this method (Li and Schmitt, 1998).
The Secondary Stress Relief Method of cores can be used to determine the direction and estimate the value of current in situ stress (Hoskins and Russell, 1981). However, not all cores exhibit distinct stress relief characteristics, and rock structure may introduce significant errors during testing.
Microfracture lithofacies analysis and axial point load analysis can also determine the direction of current in situ stress. However, the accuracy of result obtained from these methods when used individually is relatively low. They can serve as supplementary or validation methods for other testing techniques (Liang et al., 1998).
Relative to other methods, result obtained through the testing method is the most accurate. However, this method has challenges like limited data volume, high cost, and complex testing procedures.
3 Computational approaches
In order to reduce the cost of exploration and development and obtain more stress information, researchers employ well-logging data to determine the direction and magnitude of current in situ stress within reservoirs.
(Wang et al., 2022a). Well-logging data possesses characteristics such as large testing depth, large amount of information, and relatively continuous data, making characterization methods based on well-logging data have unique advantages. This approach can obtain a profile of continuous current in situ stress from top to bottom along the well drilling (Urban and Aguilera, 2015; Zhang and Yin, 2019).
Currently, the calculation of vertical principal stress has little dispute and its value is considered equal to the overlying rock mass’s gravity and can be obtained by integrating density data from the surface to the target depth (Radwan et al., 2021).
Using acoustic well logging data, the magnitude of horizontal stress can be estimated along the well drilling (Figure 1A). Due to the formation and existence conditions of stress in the formation are closer to the rock’s static testing environment, estimating horizontal stress using static mechanical parameters is more accurate (Musa et al., 2021). Therefore, it is necessary to convert dynamic mechanical parameters to static mechanical parameters using the attenuation coefficient calculated from the acoustic well logging data and then compute the horizontal principal stress within the formation. Various models exist for the calculation of horizontal principal stress, including uniaxial strain models, Mohr-Coulomb failure models, Coulomb-Naive failure models, combined spring models, Gassmann models, poroelastic strain models, biaxial strain models, and Huang’s model (Fan et al., 2014; Rasouli and Sutherland, 2014). While these models possess simple mathematical formulations and relatively low calculation precision, calculated result can be analyzed and corrected based on actual current in situ stress testing data to meet the requirements of oil and gas reservoir exploration and development. Additionally, selecting the appropriate calculation model for horizontal stress according to the research area’s characteristics and acquired data are crucial to fully leverage the advantages of current in situ stress estimation methods based on the date of acoustic well logging.
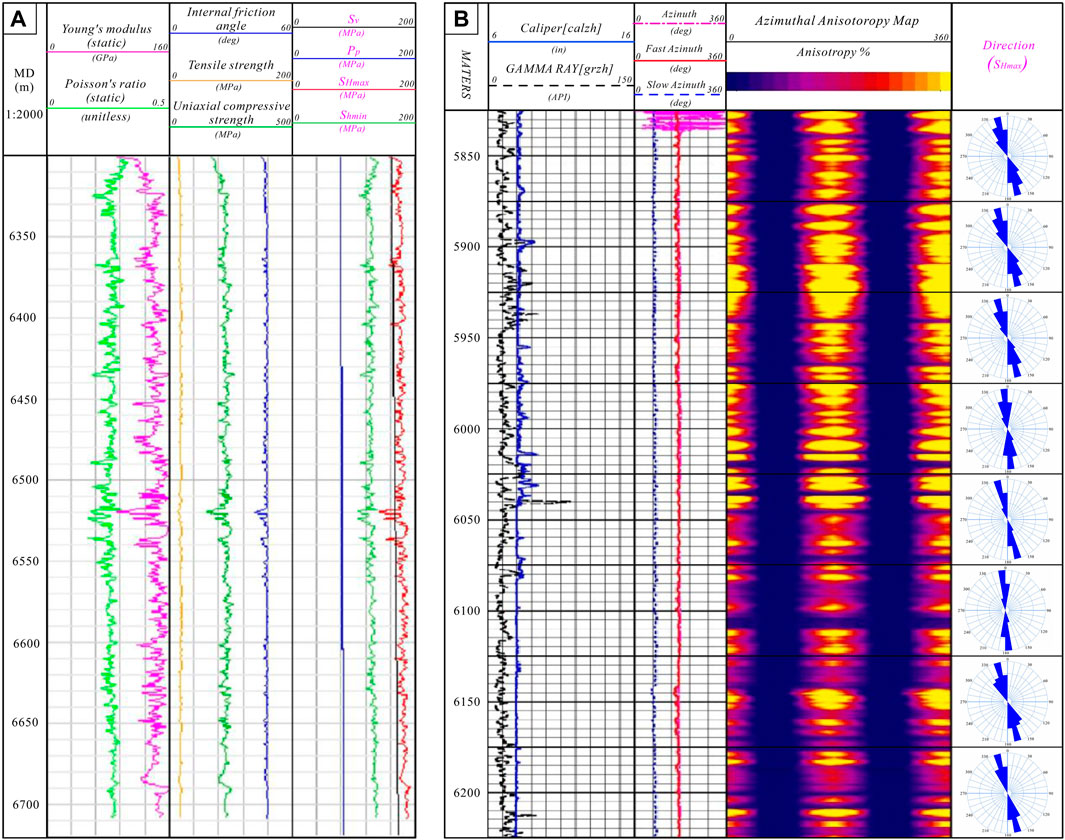
FIGURE 1. Continuous distribution profile of current in situ stress based on logging data: (A) The magnitude of current in situ stress; (B) The direction of current in situ stress.
Acoustic well logging data can also be used to identify current in situ stress direction and obtain continuous profiles of direction of current in situ stress (Figure 1B). The principle involves utilizing the characteristics of shear wave splitting in anisotropic formations, extracting fast and slow shear wave velocities and orientations from multipole acoustic well logging waveform data, and determining the directions of maximum and minimum horizontal stress based on the orientation of the fast shear wave. Additionally, micro-resistivity imaging well logging or borehole acoustic imaging well logging can identify the orientation of borehole collapse sections, induced fracture zones, and stress release fractures, allowing for the determination of horizontal principal stress direction (Willams et al., 2015). Furthermore, the well deviation logging provides borehole radius and dip angles of strata, aiding in determining borehole collapse orientation and thus deducing horizontal principal stress direction (Wang et al., 2022b).
Result obtained by calculating and analyzing current in situ stress magnitude and direction based on well-logging data need calibration through other testing methods to attain greater accuracy. Furthermore, this method only provides continuous in situ stress profiles along well depth and cannot predict in situ stress across the entire field, particularly in areas that have not been drilled.
4 Numerical methods
Some scholars have employed large-scale numerical simulation techniques for the inversion analysis of current in situ stress (Radwan and Sen, 2021). Based on relevant geological data, a three-dimensional geological computational model is established. Using measured stress values from known locations and appropriate statistical analysis methods, optimal factors for evaluating the stress field are selected (Ning et al., 2021). The constitutive relationship of rock mechanics is established, and a more realistic distribution characteristic of current in situ stress is obtained through numerical methods. By using numerical methods, we can predict the magnitude and direction of current in situ stress.
Numerical methods can predict widely ranging and continuous current in situ stress, yielding relatively accurate predictive results in undrilled areas. The accuracy of numerical simulation is directly related to the resemblance between the model and the actual reservoir and the accuracy of the model’s boundary loads. In practical applications, numerical methods are often combined with other analytical methods to enhance the accuracy of simulation results (Scelsi et al., 2019; Miao et al., 2022).
Common numerical methods include finite difference method, variational method, discrete method, boundary method, and finite element method (Sun, 2023; Sun et al., 2023). The finite difference method has a more rigorous theoretical foundation, but it mainly discretizes the fundamental governing equations and generally applies to geometric shapes with simple outlines. The finite element method discretizes the objects constituting the system and this method can simplify the analyzed object’s complex mechanical properties and boundary conditions, thus having a broader scope of application. The finite element method also features relatively simple calculation procedures, high discretization accuracy, and easy convergence of computation results, making it the primary numerical simulation method for stress field studies (Sharafisafa et al., 2023). The application of variable method, discrete method, and boundary method are relatively limited.
In recent decades, with the rapid development of computer technology, more and more scholars have been dedicated to numerical simulation research of current in situ stress of oil and gas reservoirs. Commonly used finite element analysis software includes NASTRAN, ANSYS, ABAQUS, COMSOL, ADINA, and so on. These software packages can solve complex linear and nonlinear problems, but each has relatively suitable domains. ANSYS and ABAQUS have simpler modeling methods, comprehensive pre-and post-processing, and higher accuracy in computed result, making them widely applied tools (Li et al., 2022b; Li et al., 2023b; Zhao et al., 2023).
5 Seismic prediction methods
In addition to numerical methods to predict regional current in situ stress, seismic data volume can also be utilized to estimate current in situ stress in a region (Yang et al., 2022). This method can provide a continuous distribution of current in situ stress in the study area, even in areas with few wells, and this method can overcome the limitations of location constraints. It offers lateral predictions of current in situ stress for the working area, providing theoretical guidance for well deployment and hydraulic fracturing development.
Common seismic prediction methods include the two-dimensional stress analysis method based on thin plate theory (Ma et al., 2020), the prediction of in situ stress magnitude based on pre-stack elastic parameters (Wang et al., 2020), and the prediction of in situ stress direction based on pre-stack azimuthal differences (Goodway et al., 2012).
The process of two-dimensional in situ stress seismic prediction based on thin plate theory (Figure 2A) involves analyzing the relationship between rock elastic parameters such as compressional wave velocity, shear wave velocity, density, Young’s modulus, Poisson’s ratio, and in situ stress. Sensitivity characterization parameters for in situ stress are selected (Gray et al., 2012). Nonhomogeneous elastic parameter inversion based on scattering theory is used to invert elastic parameters such as compressional to shear wave velocity ratio, Poisson’s ratio, Lamé constants, and shear modulus (Ma, 2023). Trend surface analysis is employed to fit the surface using fault data as constraints. Based on the calculated tectonic curvature distribution, a three-dimensional finite difference numerical simulation method using an elastic thin plate model is applied to simulate and estimate current in situ stress. This method integrates pre-stack seismic elastic parameter inversion technology to construct a refined nonhomogeneous mechanical model. By combining stress field numerical simulation and seismic inversion technology, the influences of factors such as structure, faults, formation thickness, and lithology are more reasonably considered, greatly improving the accuracy of simulation result.
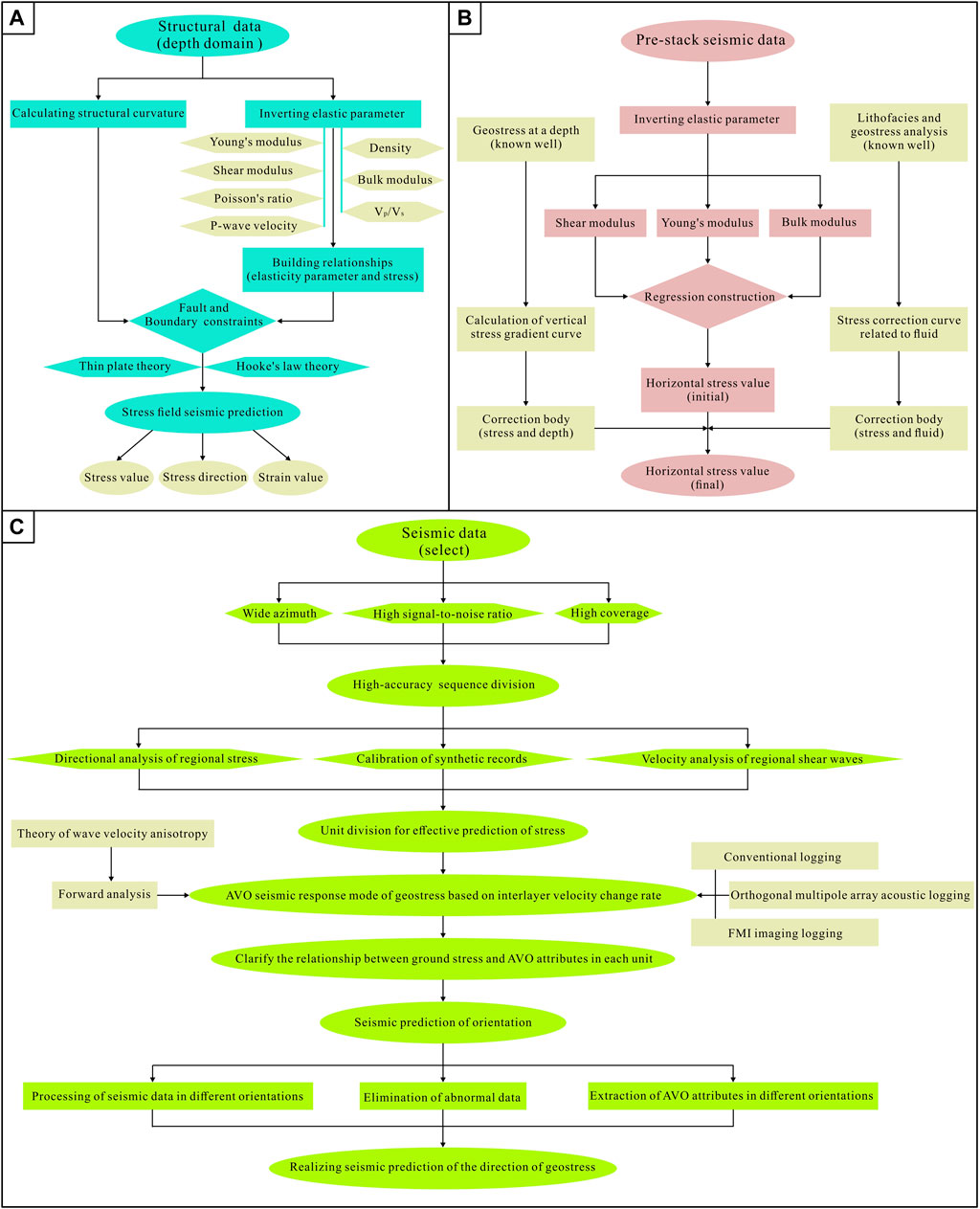
FIGURE 2. Technical roadmap for seismic prediction of current in situ stress: (A) Based on the theory of thin plates; (B) Based on pre-stack elastic parameters; (C) Based on pre-stack orientation differences.
Predicting current in situ stress magnitude based on pre-stack elastic parameters (Figure 2B) involves utilizing rock physics elastic parameters as the foundation. Multivariate linear regression establishes a stress calculation formula based on three elastic parameters, and followed by a multi-factor correction. Finally, pre-stack inversion technology is used to obtain elastic parameters such as Young’s, bulk, and shear modulus for the working area. The formula is used for fitting and correction to calculate the maximum and minimum stresses.
Predicting current in situ stress direction based on pre-stack azimuthal differences (Figure 2C) is built upon AVO forward modeling. With the assistance of transverse isotropy theory, pre-stack wide azimuthal seismic data is processed for azimuthal angle extraction. Different azimuthal AVO attributes are derived, and the relationship between seismic azimuthal and current in situ stress direction is established using azimuthal variation in transverse wave velocities due to in situ stress-induced azimuthal anisotropy (Ma et al., 2017). Validation and comparison are performed using FMI imaging and sonic anisotropy logging data to determine the in situ stress direction ultimately.
Using seismic data for current in situ stress prediction often requires establishing an in situ stress prediction model (Zhang et al., 2015). First, the relationship between stress and rock elastic and physical parameters (such as Young’s modulus, Poisson’s ratio, density, compressional wave velocity, etc.) is clarified. Then, seismic inversion methods extract relevant parameter information from seismic data and indirectly predict current in situ stress (Yang et al., 2023). This model-based current in situ stress prediction method provides good spatial coverage but is highly dependent on the accuracy of the current in situ stress prediction model (Ma et al., 2018). All factors affecting current in situ stress will significantly increase model complexity, reducing the method’s applicability (Li, 2023). It's best to use this method with other methods to enhance prediction accuracy.
6 Conclusion and recommendations
Due to the close relationship between current in situ stress and various issues in the process of oil and gas exploration and development, such as the migration and accumulation of oil and gas, wellbore stability during drilling, design of horizontal wells, reservoir modification, and well network arrangement in water injection development, scholars at home and abroad have proposed various methods for characterizing the current in situ stress of oil and gas reservoirs. We systematically review those methods for characterizing the current in situ stress of oil and gas reservoirs and arrive at the following conclusions.
(1) Testing methods encompass on-site testing and laboratory testing. While testing methods can obtain accurate results, the testing cost is relatively high, and the obtained stress data are limited to specific points.
(2) Continuous stress profiles can be obtained through calculating based on well-logging data. High accuracy magnitude and orientation of current in situ stress can be achieved by calibrating with testing method.
(3) Numerical methods can predict wide-ranging and continuous current in situ stress. Utilizing accurate modeling, boundary condition setting, parameter optimization, and known data of current in situ stress, accurate prediction result in unexplored areas can be achieved.
(4) Stress estimation using seismic data volume can also predict continuous current in situ stress, even in areas with few wells. However, it is influenced by multiple factors and should be used in conjunction with other testing methods to obtain reliable estimation result.
While various methods for characterizing current stress can measure, estimate, and predict stress from single points to wellbore profiles and even broader areas, a further in-depth research is still required for characterization methods of current in situ stress.
(1) Advancements in testing techniques and instruments. Researchers believe that the basic theory of stress testing has not undergone significant changes. The development of future testing methods will primarily focus on innovation in testing techniques and instruments (Wang, 2014). With the exploration and development of deep and ultra-deep oil and gas reservoirs, there is an increasing demand for testing deep-seated current in situ stress, necessitating the study of techniques and instruments for testing the current in situ stress in deep and ultra-deep reservoirs.
(2) Determination of combined application modes and procedures for various methods. Testing methods, computational approaches, numerical methods, and seismic prediction methods all contribute to research of in situ current stress of oil and gas reservoirs. However, each method has advantages, disadvantages, and limitations. Determining combined application modes and procedures for various methods based on reservoir conditions, geological structures, lithological characteristics, drilling designs, and fracturing methods is an ongoing challenge for engineers and researchers. This challenge aims to simultaneously satisfy the requirements of oil and gas exploration and development while minimizing time and cost.
(3) Development of new models for stress prediction based on artificial intelligence and big data. Uncertainties still exist even after calibrating geological mechanical models with core test and fracturing data. Artificial intelligence method can establish reliable and accurate prediction model without presupposing rocks' in situ mechanical behavior and physical properties (Li, 2022; Mahmoodzadeh et al., 2023). Therefore, by combining rock mechanics theory with big data methodologies, new methods for stress prediction and uncertainty characterization are significant trends in the future development of current in situ stress prediction technology.
Author contributions
TZ: Funding acquisition, Investigation, Methodology, Project administration, Writing–original draft, Writing–review and editing, Conceptualization, Data curation, Formal Analysis. QQ: Formal Analysis, Funding acquisition, Project administration, Supervision, Validation, Visualization, Writing–review and editing.
Funding
The author(s) declare financial support was received for the research, authorship, and/or publication of this article. This study was financially supported by the key R&D projects of the Deyang Science and Technology Plan (No. 2021SZ002).
Conflict of interest
The authors declare that the research was conducted in the absence of any commercial or financial relationships that could be construed as a potential conflict of interest.
Publisher’s note
All claims expressed in this article are solely those of the authors and do not necessarily represent those of their affiliated organizations, or those of the publisher, the editors and the reviewers. Any product that may be evaluated in this article, or claim that may be made by its manufacturer, is not guaranteed or endorsed by the publisher.
References
Aadnoy, B. S., Kaarstad, E., and Goncalves, C. J. (2013). “Obtaining both horizontal stresses from wellbore collapse,” in Proceeding of the SPE/IADC Drilling Conference. 163563, Amsterdam, The Netherlands, March 5–7, 2013, 1–12. doi:10.2118/163563-MS
Agharazi, A. (2016). “Determining maximum horizontal stress with microseismic focal mechanisms-case studies in the Marcellus, Eagle Ford, Wolfcamp,” in Unconventional Resources Technology Conference. URTeC:2461621, San Antonio, Texas, USA, August 1–3, 2016. doi:10.15530/urtec-2016-2461624
Banerjee, A., and Chatterjee, R. (2021). Pore pressure modeling and in situ stress determination in Raniganj basin. India. Bull. Eng. Geol. Environ. 81, 49. doi:10.1007/s10064-021-02502-0
Baouche, R., Ganguli, S. S., Sen, S., and Radwan, A. E. (2023). Assessment of reservoir stress state and its implications for Paleozoic tight oil reservoir development in the Oued Mya Basin, northeastern Algerian Sahara. Geo. Geo. 2 (1), 100112. doi:10.1016/j.geogeo.2022.100112
Bouchachi, Y., Boudella, A., Bourouis, S., Boukhallat, S., and Harbi, A. (2022). In-situ stress analysis of ahnet basin, south western Algeria: A 1D geomechanical approach. J. Afr. Earth. Sci. 196, 104678. doi:10.1016/j.jafrearsci.2022.104678
Fan, X., Gong, M., Zhang, Q., Wang, J., Bai, L., and Chen, Y. (2014). Prediction of the horizontal stress of the tight sandstone formation in eastern Sulige of China. J. Pet. Sci. Eng. 113, 72–80. doi:10.1016/j.petrol.2013.11.016
Fang, X., Feng, H., Wang, Y., and Fan, T. (2022). Prediction method and distribution characteristics of in situ stress based on borehole deformation—a case study of coal measure stratum in shizhuang block, qinshui basin. Front. Earth. Sci. 10, 961311. doi:10.3389/feart.2022.961311
Gao, F. Q. (2021). Influence of hydraulic fracturing of strong roof on mining-induced stress-insight from numerical simulation. J. Min. Strata. Control. Eng. 3 (2), 023032. doi:10.13532/j.jmsce.cn10-1638/td.20210329.001
Garavand, A., and Hadavimoghaddam, F. (2022). In situ stress assessment based on plastic behavior of borehole breakouts and machine learning. Int. J. Numer. Anal. Methods. Geomech. 47, 241–260. doi:10.1002/nag.3467
Goodfellow, S. D., and Young, R. P. (2014). A laboratory acoustic emission experiment under in situ conditions. Geophys. Res. Lett. 41 (10), 3422–3430. doi:10.1002/2014GL059965
Goodway, B., Monk, D., Perez, M., Purdue, G., Anderson, P., Iverson, A., et al. (2012). Combined microseismic and 4D to calibrate and confirm surface 3D azimuthal AVO/LMR predictions of completions performance and well production in the Horn River gas shales of NEBC. Lead. Edge 31 (12), 1502–1511. doi:10.1190/tle31121502.1
Gowida, A., Ibrahim, A. F., Elkatatny, S., and Ali, A. (2022). New empirical correlations to estimate the least principal stresses using conventional logging data. Acs. Omega. 7, 13507–13519. doi:10.1021/acsomega.1c06596
Gray, F. D., Anderson, P. F., Logel, J., Dellbecq, F., Schmidt, D., and Schmid, R. (2012). Estimation of stress and geomechanical properties using 3D seismic data. First Break 30 (3), 59–68. doi:10.3997/1365-2397.2011042
Hikweon, L., and See, H. O. (2018). Estimation of in situ stresses with hydrofracturing tests and a statistical method. Rock. Mech. Rock. Eng. 51, 779–799. doi:10.1007/s00603-017-1349-1
Holcomb, D. J. (2013). Observations of the Kaiser Effect under multiaxial stress states: implications for its use in determining in situ stress. Geophys. Res. Lett. 20, 2119–2122. doi:10.1029/93GL01270
Hoskins, E. R., and Russell, J. R. (1981). “The origin of the measured residual strains in crystalline rocks,” in Mechanical behavior of crustal rocks, (the handin volume), geophysical monograph 24 (Washington, D, C: AGU), 187–198. doi:10.1029/GM024p0187
Kim, H., Xie, L., Min, K. B., Bae, S., and Stephansson, O. (2017). Integrated in situ stress estimation by hydraulic fracturing, borehole observations and numerical analysis at the EXP-1 borehole in Pohang, Korea. Rock. Mech. Rock. Eng. 50 (12), 3141–3155. doi:10.1007/s00603-017-1284-1
Kruszewski, M., Montegrossi, G., Parisio, F., and Saenger, E. K. (2022). Borehole observation-based in situ stress state estimation of the Los Humeros geothermal field (Mexico). Geomech. Energy. Environ. 32, 100392. doi:10.1016/j.gete.2022.100392
Li, Y., and Schmitt, D. R. (1998). Drilling-induced core fractures and in situ stress. J. Geophys. Res-atmos. 103 (B3), 5225–5239. doi:10.1029/97/JB02333
Li, H., Tang, H., Qin, Q., Zhou, J., Qin, Z., Fan, C., et al. (2019). Characteristics, formation periods and genetic mechanisms of tectonic fractures in the tight gas sandstones reservoir: A case study of xujiahe formation in YB area, sichuan basin, China. J. Pet. Sci. Eng. 178, 723–735. doi:10.1016/j.petrol.2019.04.007
Li, Y., Zhou, D., Wang, W., Jiang, T., and Xue, Z. (2020). Development of unconventional gas and technologies adopted in China. Energy. Geosci. 1, 55–68. doi:10.1016/j.engeos.2020.04.004
Li, J., Li, H., Yang, C., Wu, Y., Gao, Z., and Jiang, S. (2022a). Geological characteristics and controlling factors of deep shale gas enrichment of the Wufeng-Longmaxi Formation in the southern Sichuan Basin, China. Lithosphere S12, 4737801. doi:10.2113/2022/4737801
Li, J., Qin, Q., Li, H., and Wan, Y. (2022b). Numerical simulation of the stress field and fault sealing of complex fault combinations in Changning area, Southern Sichuan Basin, China. Enery. Sci. Eng. 10, 278–291. doi:10.1002/ese3.1044
Li, J., Li, H., Yang, C., Ren, X., and Li, Y. (2023a). Geological characteristics of deep shale gas and their effects on shale fracability in the Wufeng–Longmaxi Formations of the southern Sichuan Basin, China. Lithosphere 2023 (1), 4936993. doi:10.2113/2023/4936993
Li, J., Qin, Q., Li, H., Zhou, J., Wang, S., Zhao, S., et al. (2023b). Numerical simulation of the palaeotectonic stress field and prediction of the natural fracture distribution in shale gas reservoirs: A case study in the longmaxi formation of the luzhou area, southern sichuan basin, China. Geol. J., 1–16. doi:10.1002/gj.4744
Li, H. (2022). Research progress on evaluation methods and factors influencing shale brittleness: A review. Energy. Rep. 8, 4344–4358. doi:10.1016/j.egyr.2022.03.120
Li, H. (2023). Coordinated development of shale gas benefit exploitation and ecological environmental conservation in China: A mini review. Front. Ecol. Evol. 11, 1232395. doi:10.3389/fevo.2023.1232395
Liang, Z., Fernandes, C. P., Magnani, F. S., and Philippi, P. C. (1998). A reconstruction technique for three-dimensional porous media using image analysis and Fourier transforms. J. Pet. Sci. Eng. 21 (3-4), 273–283. doi:10.1016/S0920-4105(98)00077-1
Ma, N., Yin, X., Sun, C., and Zong, Z. (2017). The in-situ stress seismic prediction method based on the theory of orthorhombic anisotropic media. Chin. J. Geophys. 60 (12), 4766–4775. doi:10.6038/cig20171218
Ma, N., Yin, X., Sun, C., and Zong, Z. (2018). Inversion for crustal stress based on azimuthal seismic data. Chin. J. Geophys. 61 (2), 697–706. doi:10.6038/cjg2018L0183
Ma, N., Yin, X., Zong, Z., Sun, C., and Wang, S. (2020). Structural stress prediction method based on curvature attribute. Pet. geophys. expl. 55 (3), 643–650. doi:10.13810/j.cnki.issn.1000-7210.2020.03.020
Ma, H. (2023). Application of trend surface analysis in the study of reservoir distribution regularity in Wayaobu oilfield. J. Geol. 47 (2), 175–181. doi:10.3969/j.issn.1674-3636.2023.02.009
Mahmoodzadeh, A., Nejati, H. R., Mohammed, A. H., Mohammadi, M., Ibrahim, H. H., Rashidi, S., et al. (2023). Prediction of minimum horizontal stress in oil wells using recurrent neural networks. Energy. Sci. Eng. 223, 211560. doi:10.1016/j.geoen.2023.211560
Miao, Y., Cheng, L., Zheng, X., Chen, X., Wang, Z., and Xu, B. (2022). Study on dynamic response characteristics of mining stress in shallow deep mining roadway. J. Min. Strata. Control. Eng. 4 (6), 063015. doi:10.13532/j.jmsce.cn10-1638/td.2022.06.001
Musa, I. H., Tan, C. P., Leem, J., Altaf, I., and Adams, M. (2021). “Anisotropic geomechanical rock properties modelling for unconventional shale gas formation,” in Proceeding of the International Petroleum Technology Conference, Virtual, March–April, 2021. doi:10.2523/IPTC-21281-MS
Ning, Y., Tang, H., Smith, J. V., Zhang, B., Shen, P., and Zhang, G. (2021). Study of the in situ stress field in a deep valley and its influence on rock slope stability in Southwest China. Bull. Eng. Geol. Environ. 80 (4), 3331–3350. doi:10.1007/s10064-020-02094-1
Radwan, A., and Sen, S. (2021). Stress path analysis for characterization of in situ stress state and effect of reservoir depletion on present-day stress magnitudes: reservoir geomechanical modeling in the gulf of suez rift basin, Egypt. Nat. Resour. Res. 30, 463–478. doi:10.1007/s11053-020-09731-2
Radwan, A. E., Abdelghany, W. K., and Elkhawaga, M. A. (2021). Present-day in-situ stresses in Southern Gulf of Suez, Egypt: insights for stress rotation in an extensional rift basin. J. Struct. Geol. 147, 104334. doi:10.1016/j.jsg.2021.104334
Rasouli, V., and Sutherland, A. (2014). Geomechanical characteristics of gas shales: A case study in the north perth basin. Rock. Mech. Rock. Eng. 47, 2031–2046. doi:10.1007/s00603-013-0531-3
Ren, N. K., and Hudson, P. (1987). Predicting the in-situ state of stress using differential wave velocity analysis. Int. J. Mech. Min. 24 (1), 1235–1244. doi:10.1016/0148-9062(87)91351-9
Sanada, H., Hikima, R., Tanno, T., Matsui, H., and Sato, T. (2013). Application of differential strain curve analysis to the Toki Granite for in situ stress determination at the Mizunami underground research laboratory, Japan. Jpn. Int. J. Mech. Min. 59, 50–56. doi:10.1016/j.ijrmms.2012.12.015
Scelsi, G., De Bellis, M. L., Pandolfi, A., Musso, G., and Della Vecchia, G. (2019). A step-by-step analytical procedure to estimate the in-situ stress state from borehole data. J. Pet. Sci. Eng. 176, 994–1007. doi:10.1016/j.petrol.2019.01.100
Sharafisafa, M., Sato, A., Sainlki, A., Shen, L., and Aliabadian, Z. (2023). Combined finite-discrete element modelling of hydraulic fracturing in deep geologically complex reservoirs. Int. J. Rock. Mech. Min. 167, 105406. doi:10.1016/j.ijrmms.2023.105406
Subrahmanyam, D. S. (2019). Evaluation of hydraulic fracturing and overcoring methods to determine and compare the in situ stress parameters in porous rock mass. Geotech. Geol. Eng. 37 (6), 4777–4787. doi:10.1007/s10706-019-00937-7
Sugimoto, T., Ishitsuka, K., and Lin, W. (2021). Theoretical investigation on new analyzing procedure of anelastic strain recovery method for stress measurements based on bayesian statistical modeling. Mat. Trans. 62 (12), 1695–1702. doi:10.2320/matertrans.MT-Z2021015
Sun, X., Chen, G., Huang, M., Zhang, S., Yang, J., and Ma, J. (2023). Method for analyzing the evolution characteristics of in-situ stress field considering rheology and degradation: A deeply incised valley in qinghai-tibet plateau, case study. Eng. Geol. 315, 107029. doi:10.1016/j.enggeo.2023.107029
Sun, Z. M. (2023). Superimposed hydrocarbon accumulation through multi-source and multi-stage evolution in the cambrian xixiangchi group of eastern sichuan basin: A case study of the pingqiao gas-bearing anticline. Energy. Geosci. 4, 131–142. doi:10.1016/j.engeos.2022.09.001
Urbam, E., and Aguilera, R. (2015). “Determination of principal in-situ stress magnitude from well logs in unconventional reservoirs: A practical application in willesden green field, Canada,” in SPE Latin American and Caribbean Petroleum Engineering Conference, Quito, Ecuador, November 18–20, 2015. doi:10.2118/177261-MS
Wang, C., Song, W., Lin, H., Zhang, Y., Gao, Q., and Wei, X. (2020). Prediction method of in-situ stress based on pre-stack anisotropic parameter inversion. Geophys. Geochem. Expl. 44 (1), 141–148. doi:10.11720/wtyht.2020.1172
Wang, P., Zhou, H., Wan, G., and Zheng, P. (2021). Stress characteristics of different mining directions adjacented fault under hard thick roof. J. Min. Strata. Control. Eng. 3 (4), 043529. doi:10.13532/j.jmsce.cn10-1638/td.20210525.003
Wang, S., Wang, G., Li, D., Wu, X., Chen, X., Wang, Q., et al. (2022a). Comparison between double caliper, imaging logs, and array sonic log for determining the in-situ stress direction: A case study from the ultra-deep fractured tight sandstone reservoirs, the cretaceous bashijiqike formation in Keshen8 region of kuqa depression, tarim basin, China. Pet. Sci. 19 (6), 2601–2617. doi:10.1016/j.petsci.2022.08.035
Wang, Z., Zeng, L., Li, J., Liu, D., and Yu, J. (2022b). In-situ stress logging interpretation methods and reliability analysis. J. Southwest. Pet. Univ. Sci. Tech. Ed. 44 (6), 1–9. doi:10.11885/j.issn.1674-5086.2020.11.13.01
Wang, C. (2014). Brief review and outlook of main estimate and testing methods for in-situ stresses in rock mass. Geol. Rev. 60 (5), 971–996. doi:10.16509/j.georeview.2014.05.005
Willams, J., Fellgett, M. W., Kingdon, A., and Williamson, J. P. (2015). In-situ stress orientations in the UK southern north sea: regional trends, deviations and detachment of the post zechstein stress field. Mar. Pet. Geol. 67, 769–784. doi:10.1016/j.marpetgeo.2015.06.008
Wu, Z., Cui, C., Jia, P., Wang, Z., and Sui, Y. (2022a). Advances and challenges in hydraulic fracturing of tight reservoirs: A critical review. Energy. Geosci. 3, 427–435. doi:10.1016/j.engeos.2021.08.002
Wu, Z., Tang, M., Zuo, Y., Lou, Y., Wang, W., Liu, H., et al. (2022b). Acoustic emission-based numerical simulation of tectonic stress field for tectoclase prediction in shale reservoirs of the northern Guizhou area, China. Energy. Geosci. 3, 436–443. doi:10.1016/j.engeos.2021.10.005
Xiong, Q., and Hampton, J. C. (2021). A laboratory observation on the acoustic emission point cloud caused by hydraulic fracturing, and the post-pressure breakdown hydraulic fracturing re-activation due to nearby fault. Rock. Mech. Rock. Eng. 54 (12), 5973–5992. doi:10.1007/s00603-021-02585-x
Yang, D., Ning, Z., Li, Y., Lv, Z., and Qiao, Y. (2021). In situ stress measurement and analysis of the stress accumulation levels in coal mines in the northern Ordos Basin, China. Int. J. Coal. Sci. Techn. 8, 1316–1335. doi:10.1007/s40789-021-00407-7
Yang, J., Zhang, F., and Li, C. (2022). Inversion of horizontal in situ stress field based on wide-azimuth seismic data. J. Geophys. Eng. 2, 131–141. doi:10.1093/jge/gxac006
Yang, J., Zhang, F., Sun, Z., and Zheng, X. (2023). Nonlinear constitutive relation in acoustoelastic-orthotropic media and its wide azimuth seismic reflection coefficient characteristics for in-situ stress prediction. J. Appl. Geophys. 214, 105063. doi:10.1016/j.jappgeo.2023.105063
Ye, T., Tao, W., Li, H., Zhang, Y., and Liu, R. (2023). Simulation analysis of rockfall movement characteristics with debris slope using Unity3D based on UAV remote sensing DSM: A case study of the G318 highway on the Tibetan plateau. Front. Ecol. Evol. 10, 1221915. doi:10.3389/fevo.2023.1221915
Yin, X., Ma, N., Ma, Z., and Zong, Z. (2018). Review of in-situ stress prediction technology. Geophys. Prosp. Pet. 57 (4), 488–504. doi:10.3969/j.issn.1000-1441.2018.04.001
Zhang, H., and Yin, S. (2019). Inference of in situ stress from thermoporoelastic borehole breakouts based on artificial neural network. Int. J. Numer. Anal. Mater. 43, 2493–2511. doi:10.1002/nag.2982
Zhang, G., Chen, J., Chen, H., Ma, Z., Li, C., and Yin, X. (2015). Prediction for in-situ formation stress of shale based on rock physics equivalent model. Chin. J. Geophys. 58 (6), 2112–2122. doi:10.6038/cjg20150625
Zhao, T., Qin, Q., Li, H., Wang, S., and Mou, X. (2023). Evaluation of the current in situ stress in the middle permian maokou formation in the longnüsi area of the central sichuan basin, China. Geosci 15 (1), 20220524. doi:10.1515/geo-2022-0524
Keywords: current in situ stress, characterization method, testing method, computational approaches, numerical simulation, seismic prediction, oil and gas reservoirs
Citation: Zhao T and Qin Q (2023) Characterization methods for current in-situ stress in oil and gas reservoirs: a mini review. Front. Earth Sci. 11:1276807. doi: 10.3389/feart.2023.1276807
Received: 13 August 2023; Accepted: 26 September 2023;
Published: 09 October 2023.
Edited by:
Shuai Yin, Xi’an Shiyou University, ChinaCopyright © 2023 Zhao and Qin. This is an open-access article distributed under the terms of the Creative Commons Attribution License (CC BY). The use, distribution or reproduction in other forums is permitted, provided the original author(s) and the copyright owner(s) are credited and that the original publication in this journal is cited, in accordance with accepted academic practice. No use, distribution or reproduction is permitted which does not comply with these terms.
*Correspondence: Tianbiao Zhao, enRieWhnZEAxNjMuY29t