- 1Geo-Ocean, UMR 6938 CNRS–Université de Brest, Plouzané, France
- 2Jersey Geology, St Brelade, Jersey, United Kingdom
- 3Retired, St Jean de Cornies, France
- 4Service Géologie et Environnements de Surface, Agence nationale pour la gestion des déchets radioactifs, Châtenay-Malabry, France
The evolution of the passive Armorican margin (Western France) during the Neogene and Quaternary was analyzed using field data. The morphology of the margin attests to a late Hercynian shaping, further deformation during the Mesozoic mid-Atlantic opening, during the Alpine Orogeny, and ultimately, a Late Cenozoic uplift, mostly related to an onshore isostatic accommodation in response to erosion and limited tectonic activity. A very limited strike–slip dynamic, with very low seismicity, accommodated the Neogene–Pleistocene N170 strains around the rigid Armorican terrane. The South Armorican domain and English Channel floor include shear zones that adjusted the Alpine convergence, facilitating its transpressive slip to the west. The Permo-Triassic N150 faults were reactivated during the inversion phases that began after the Bartonian under the distal control of the Alpine convergence and the decrease in the Atlantic spreading rate after 34 Ma. The Armorican marine platforms were stable after the late Eocene and slightly subsident, experiencing pulsed episodes of transient lithospheric doming during the Neogene and Quaternary. Co-seismic activity onshore without surface rupture was recorded around ∼5.3 Ma, ∼3.7 Ma, ∼2.4–1.2 Ma, and ∼400–250 ka, in tandem with an inland exhumation driven by isostatic adjustment due to an intensification of periglacial erosion at the onset of the early interstadials or by agriculture. Low-magnitude and ubiquitous shallow seismic activities seem to be related today to an isostatic uplifted old brittle–ductile transition due to the accumulation of shearing strain.
1 Introduction
The Armorican Massif is a plateau peninsula in Western Europe (Figure 1), locally covered by Permian, Mesozoic, and Cenozoic marine sediments in the present day. It is the inheritor of two main Paleozoic orogenic cycles—the Cadomian and Hercynian—that were related to oceanic sutures and were founded in a Proterozoic Icartian basement (around 2 Gyr) (Ziegler and Dézes, 2007; see the synthesis in the work of Vernhet (2003), Ballèvre et al. (2013)).
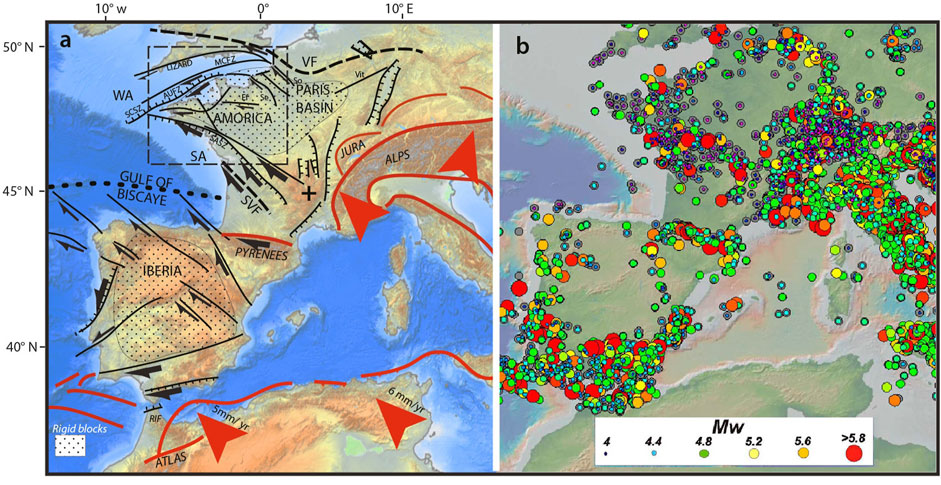
FIGURE 1. (A) Global Tortonian–Pleistocene tectonic setting, updated and modified from the work of Somoza et al. (2021). (B) present-day seismicity of Western Europe, SHARE, 2013 (Seismic Hazard Harmonization in Europe).
To evaluate the geodynamics and their regional impact on past and present-day seismicity, it is important to examine the existing deep structures that are susceptible to reactivation and that can modify the morphology of, especially, the coastal platforms. The current seismicity is low in Normandy but a little higher offshore, along the faults of the graben system of the English Channel and along the Armorican shear zones, particularly the southern ones. Today, the seismicity never exceeds Mw 6 (Sisfrance BRGM-EDF-IRSN/SisFranc, 2010; SHARE, 2013; see the synthesis in the work of Beucler et al. (2021)).
MCSZ: Middle Channel Shear Zone; AUSZ: Alderney–Ushant SZ; NASZ; North Armorican SZ; SASZ: South Armorican SZ; EF: Eure Fault; Se: Seine Fault; Vit: Vittel Fault; SO: Somme Fault; SVF and VF: Hercynian fronts; WA, Western Channel Approaches; SA, South Armorican Platform; dotted area = rigid basement; and stippled window: analyzed zone (see Figure 2).
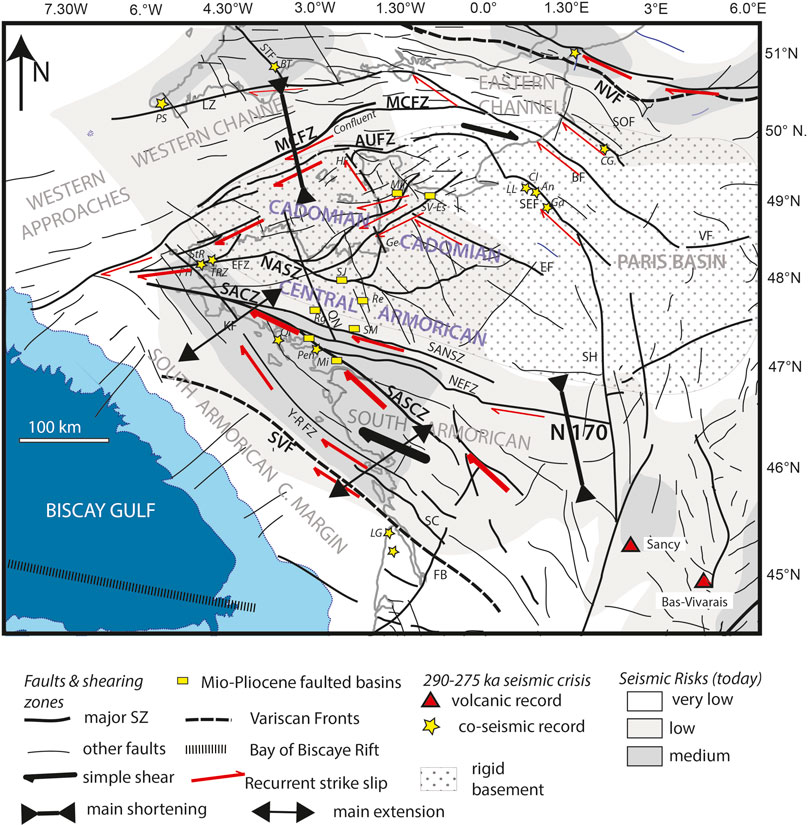
FIGURE 2. Map showing the surface faulting (compiled from the work of Lefort (1975), Ziegler and Dezès (2007), and Ballèvre et al. (2013)) related to its origin, the present-day seismic risk (adapted from the SI-Hex catalog), and the boundaries of the Hercynian Orogeny (black dashes—northern and southern Hercynian fronts). AUFZ: Alderney–Ushant Fault Zone; BF: Bray Fault; CGSZ: Cancale–Granville Shear Zone; EF: Senonche–Eure Fault; ESZ: Elorn Shear Zone; FB: Bordeaux Fault; FCSZ: La Fresnaye–Coutances Shear Zone; KF: Kerforne Fault; LB: Limagne Basin, LHSZ: La Hague Shear Zone; LMFZ: La Marche Fault Zone; LQF: Le Quessoy Fault; LZFZ: Lizard Fault Zone; LTSZ: Locquemeau–Tregor Shear Zone; MASZ: Monts d’Arrée Shear Zone; MCFZ: Mid-Channel Fault Zone; MF: Machecoul Fault; MNSZ: Montagnes Noires Shear Zone; NCSZ: North Cotentin Shear Zone; NEFZ: Nort-sur-Erdre Fault Zone; NASZ; North Armorican Shear Zone; SANSZ: South Armorican Northern Shear Zone; SASSZ: South Armorican Southern Shear Zone; NVF/SVF: Northern/Southern Variscan fronts; PGSZ: Porspoder–Guisseny Shear Zone; SC: Saintes–Cognac graben; SEF: Seine Fault; SH: Sillon Houiller; SOF: Somme Fault; STF: Sticklepath Fault; and VF: Vittel Fault; Y-R FZ: Yeu-Ré Fault Zone. Sites: LG: Le Gurp; Mi: Missilac Basin; Pen: Pénestin; Qi: Quiberon; PH: Pen Hat; TRZ: Trez Rouz; Rg: Réguigny; SM: St-Malo-de-Phily; Re: Rennes Basin; SJ: St Jouan; SV-Es: St Vigor Esquay; Mil: Millières; Ge: Genêts; LL: La Londe; Cl: Cléon; An: Anneville; GA: La-Garenne-de-Andelys; CG: Cagny Epinette; Sa: Sangatte; PS: Prah Sands; and BT: Beauvais–Tracey.
Global tectonics have contributed significantly to the stratigraphic record of sea level (SL) change (Cloetingh and Haq, 2015). This makes it possible to analyze the recorded signatures of regional SL change as a way of interpreting the geodynamic evolution of the terrane. Most stratigraphic deposits represent regionally increased accommodation space for sedimentation, as exemplified by the Tortonian and Piacenzian “Red Sands” (Van Vliet-Lanoë et al., 1998a). An examination of the stratigraphy allows an analysis of the record of paleoseismicity clusters in the Neogene and Quaternary. These are represented by various types of fault offsets and more distal co-seismic processes (Figure 2).
Apart from the major structures active at the regional scale, the impact of erosion, under the control of climate change, on the uplift and/or tectonics of Armorica also needs to be investigated because it poses a potential trigger for seismicity. Following cooling and the Tortonian lowstand (LS) at 11.6 Ma, it becomes difficult to separate tectonic signals from the global isostatic accommodation associated with the major Neogene glaciations.
In this work, we synthesized the Neogene to Quaternary geodynamic evolution of Armorica and its sedimentary SL record, along with the erosion and co-seismic activity that has occurred at the regional scale, based on the
• potential uplift mechanisms and evidence for these
• regional tectonic evolution from the Late Paleogene to Pleistocene
• shaping of and sedimentation on the marine platforms that may indicate vertical displacements
• relative stratigraphic positioning of the recorded co-seismic events with possible rupture, which involved some data reinterpretation, especially in Normandy
• and the potential source of the present-day seismicity
2 Methods
For the Neogene and Pleistocene, our group carried out a classical field survey investigation of onshore sedimentary sequences preserved in quarries, located in tectonic basins, and found along the shore face. Special attention was applied to sedimentary deformations (faults and involutions (Figure 2; Figure 3; Figure 4; Figure 5; Figure 6)) and their relationship with river incision onshore (digital elevation model). Data acquisition from the field mostly began in the 1980s and has continued to the present in Brittany and Normandy, supported by physical dating. Electronic spin resonance (ESR) dating of the sediments was of prime importance to locate the events in the geological frame. ESR dating was performed at the Geochronology laboratory in the Musée d’Histoires Naturelles (Paris) following standard procedures (Laurent et al., 1998; Bahain et al., 2007), further completed by some luminescence dating (Quaternary). They were compared with adjacent regions along the English Channel and older publications. The dating results are provided in Table 1 of Supplementary Material. Altitudes are given in NGF (the general leveling of France).
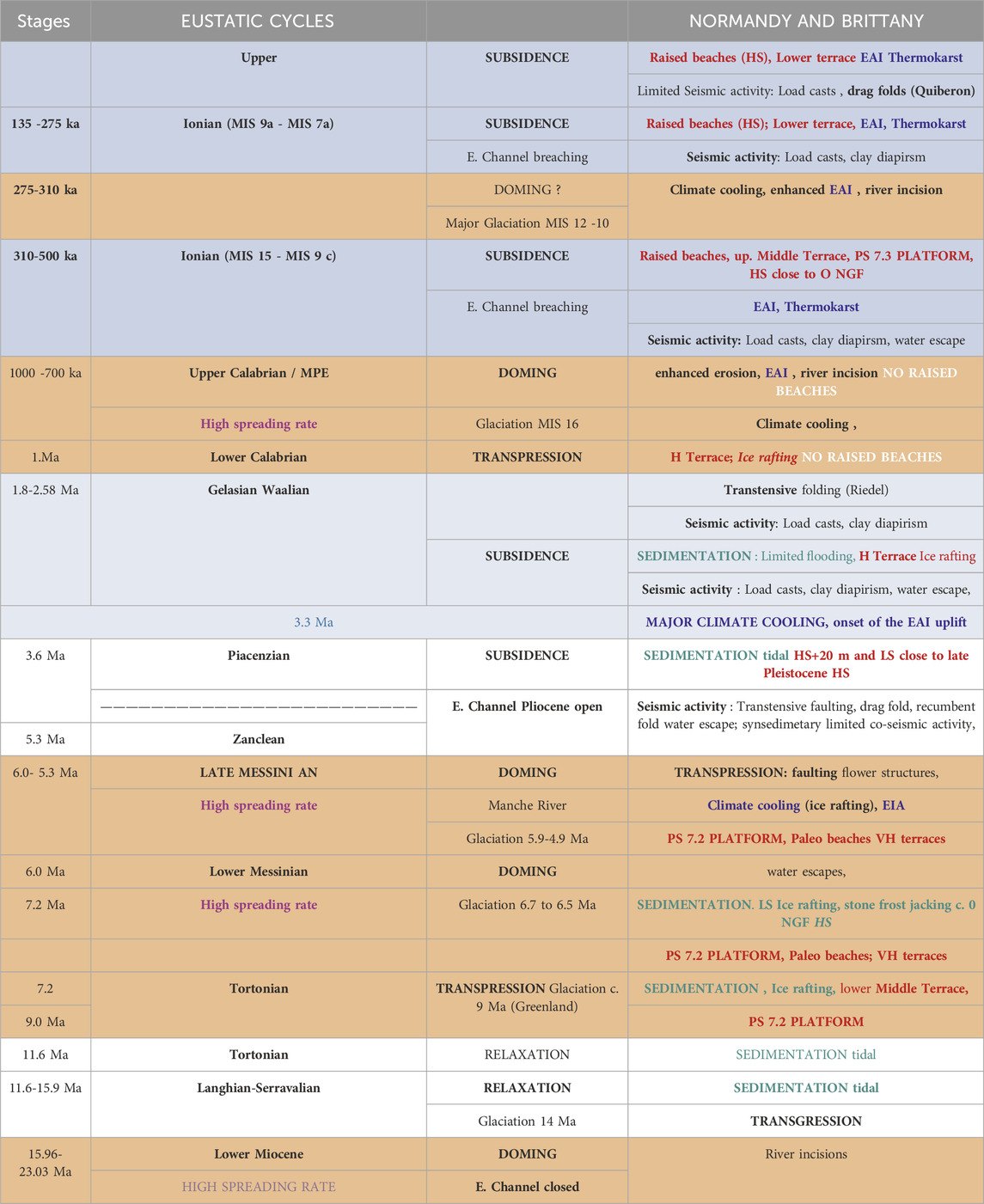
TABLE 1. Synthesis on the global functioning of Armorica during the Neogene and Pleistocene combination of the tectonic, seismic, and climatic Cenozoic events in Armorica. In bold, main phases.
Regional appreciation of the erosional budget has been performed at the coast by the evaluation in one periglacial cycle of onshore cliff erosion (Lautridou, 1985) or on the shore platform (Van Vliet-Lanoë, 1987). Another source was given for chalky substratum by Antoine et al. (1997) on the base of ESR-dated terraces (Somme River). This system has been extended in land by the altitude of ESR-dated terraces in the eastern and south-eastern borders of other Paleozoic massives (Massif Central and Vosges forelands). The obtained values are coherent with those obtained by Mazzotti et al. (2020) (Alpine Foreland and Massif Central). Historical erosion value has been appreciated in Armorica on the basis of Holocene soil truncation (Van Vliet-Lanoë et al., 1992).
Concerning the available marine seismic lines, we reanalyzed published or unpublished data in 2018 for the Cenozoic along the English Channel, offshore of the Cotentin Peninsula, and in Seine Bay (Benabdellouahed, 2011) in combination with datasets from the Western Approaches (Evans and Hughes, 1984; Le Roy et al., 2011) and the southern Brittany margin (Thinon et al., 2001).
The field traces of paleoseismic activity considered in this paper (published in the work of Van Vliet-Lanoë et al. (1997), (2002), (2004)) have been partly used in the NEOPAL database (2009) and in the work of Jomard et al. (2017). They have been completed and revised by our more recent observations (e.g., from Penestin in 2009 and Trez Rouz in 2019). They also have been analyzed here in the context of new stratigraphic reinterpretations for the Cotentin Neogene (see Supplementary Material). The co-seismic features observed include ruptures, fault offsets and/or flower structures (Figure 3), dewatering pipes, load casting (Figure 4), and shale diapirism (Figure 5), all of which have affected the sediments or the weathered basement (McCalpin, 2009; Van Loon, 2009).
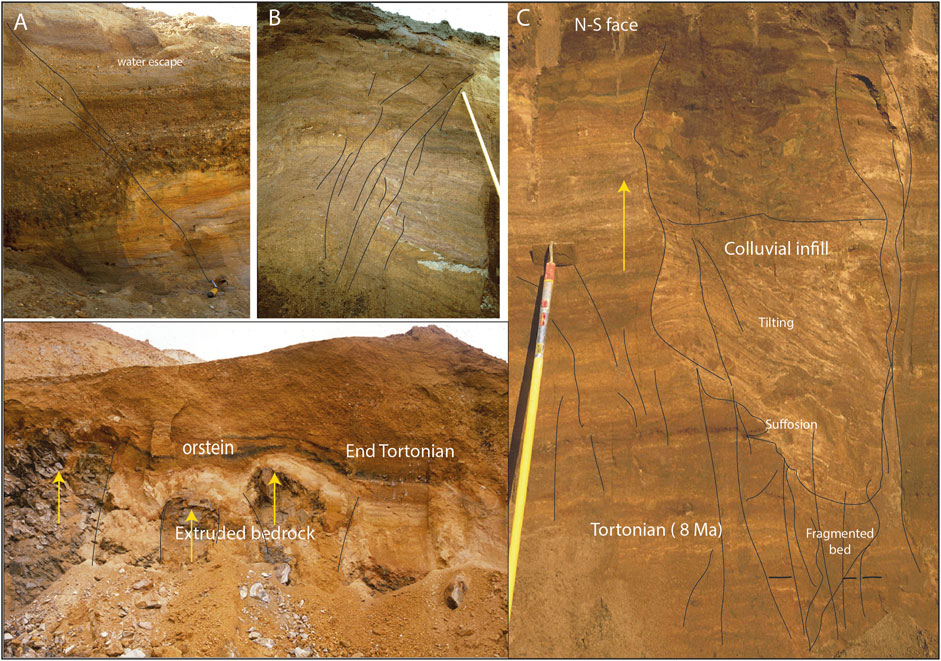
FIGURE 3. Transpressive faulting and tectonism, Central Brittany (Oust River, Réguigny Lafarge quarry): (A, B, C) evidence of Messinian transpressive faulting (N150) and (C) transtensive rupture, probably in riedel, infilled by colluvial infilling (images: Van Vliet-Lanoë).
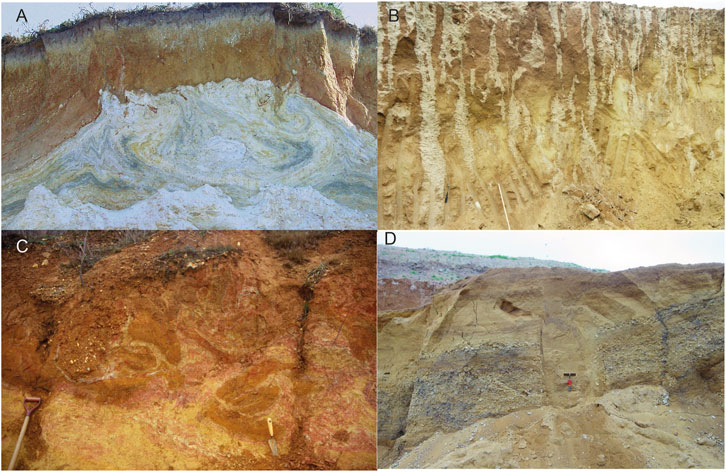
FIGURE 4. Example of various co-seismic deformations. (A) Pénestin: giant load (8 m wide) with lateral wrinkling (arrow) in saprolite with wrinkling, 275 ka earthquake (SASZ). (B) St-Malo-de-Phily: a set of vertical fractures in consolidated sands up to 3 m height (organs) above a reactivated SASZ fault line, leached by precipitations, probably Early Pliocene ot lzte Messinian. (C) Reguigny basin: classical load casts (<1.4 Ma). (D) St Jouan (NASZ): water escape pipes in Messinian gravel and the Early Pliocene, faulted after consolidation (images: Van Vliet-Lanoë).
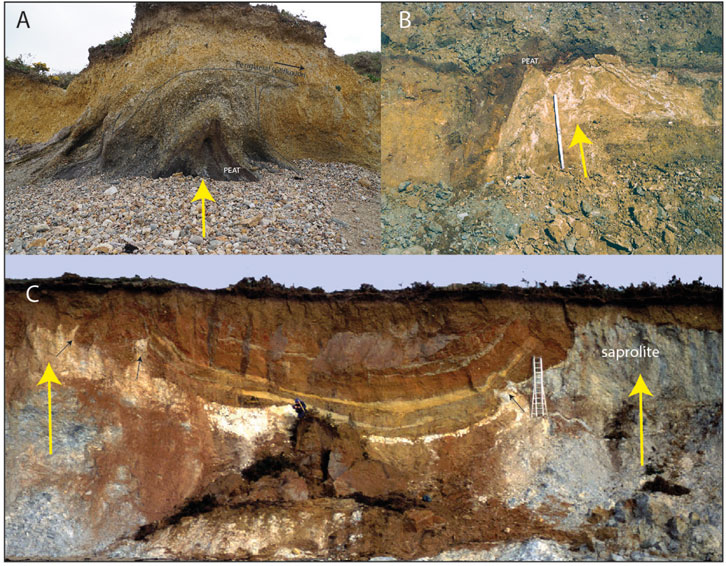
FIGURE 5. Shale diapirism. (A) Trez Rouz: MIS 15 peat injected in shaley slope deposits. (B) Landerneau: extrusion of a Gelasian tidalite in a terrace gravel. (C) Pénestin: saprolite extruded on each side of estuarian and fluvial complexes (400–300 ka) (images: Van Vliet-Lanoë).
2.1 Remarks
In Pleistocene Armorican outcrops, paleoseismic features (Van Vliet-Lanoë et al., 2004; 2019) are often confused with those resulting from periglacial activities only (cryoturbation) due to their converging morphologies as load casts, commonly attributed to cryoturbation (Lautridou, 1985; Vandenberghe et al., 2016; Bertran et al., 2019).
By opposition, superficial collapse commonly results in antithetic or listric short faults from lateral slumping. We reinterpreted these in terms of periglacial activities, of karstic or thermokarstic collapse, especially in sands (karst: Baize et al., 2002; thermokarst: Van Vliet-Lanoë et al., 2017). It is related to Pleistocene paleo-permafrost melting. Deep-seated active tectonism shows a fault offset that is accentuated with depth.
The normal to “pseudo-reverse” and “curved” superficial faulting observed in the Mio–Pliocene Red Sands, with the fault offset increasing toward the surface, corresponds to a collapsed hydrolaccolith (thermokarst as in Quaternary), the sandy bottom of paleo valleys, or lower valley sides in most of Armorica (Figure 6).
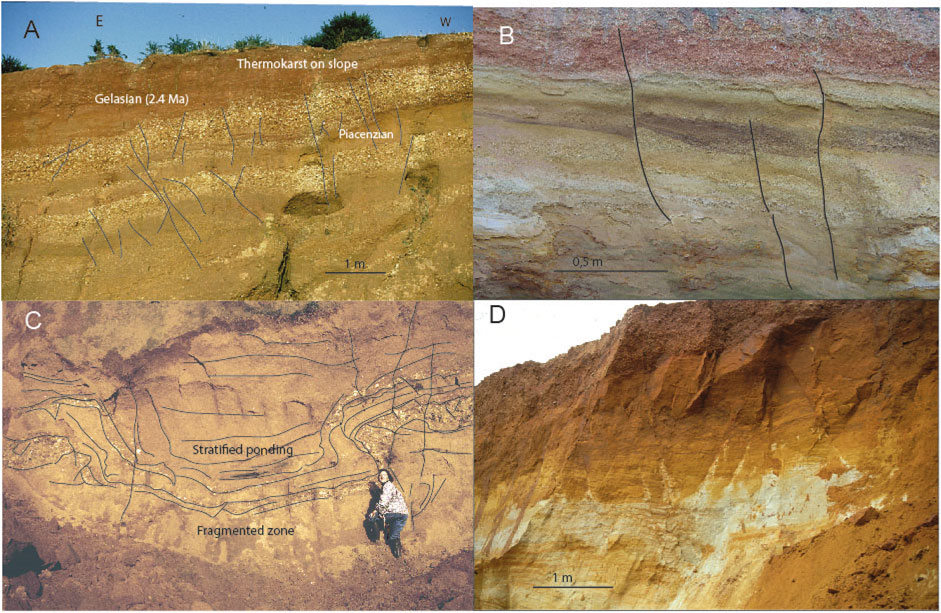
FIGURE 6. Faulting related to thermokarst collapse. (A) Reguiny: thermokarst deformation near the foot of a gentle slope. (B) Typical shallow fractures for thermokarstic activity. (C) Typical collapse of a hydrolaccolith. (D) Rennes Basin (La Frelonnière) fractured basement related to permafrost melt (images: Van Vliet-Lanoë).
3 Data
3.1 Geologic setting and climate evolution
3.1.1 Paleozoic and Mesozoic heritages
In regions of Southern Europe and North Africa, the Armorican Massif was part of the North Gondwanan margin during Paleozoic times. The development of the Cadomian Orogeny came from the subduction of the Celtic Ocean crust (north of Brittany) beneath the old Icartian continental edge. This resulted in a back-arc basin and an active continental margin during the Late Proterozoic (Ballèvre et al., 2013). The closing of the Celtic Ocean basin led to the rise of the Cadomian Cordillera and its over-thrusting onto the continental margin (see the synthesis in the work of Ballèvre et al. (2013) and Baptiste (2016)).
This led, with some metamorphism, to the formation of the old Cadomian rigid block. The major shaping of the Armorican basement was related to the Late Paleozoic Hercynian Orogeny.
This resulted from the stacking of continental masses during a continuous period of collision, first in the north, during the closing of the Rheic Ocean (NASZ), and then, in the south, leading to the formation of the SASZ (Ballèvre et al., 2013).
The Armorican basement was further reworked in a southerly directed Hercynian fold-and-thrust belt (Le Gall et al., 2014). The Paleozoic crust was stepwise reactivated from the Pennsylvanian through the Permian, first by an aborted rifting, initiated during the Pangea breakup, and then by the opening of the Central Atlantic Ocean (Kristoffersen, 1978; Chadwick et al., 1989; Srivastava and Verhoef, 1992; Faure, 1995). This led to the onset of the westward deformation of Armorica, with its extensive N180 and N150E faults and crustal thinning. To the north, the inherited offshore Alderney–Ushant Fault Zone (AUFZ) and the Middle Channel Fault Zone (MCFZ), as shown in Figure 2, reactivated as normal faults, forming onshore and offshore E–W Permo-Triassic passive-extension basins, such as the Weald Basin and the Porcupine Basin, SW of Ireland (Calvès et al., 2012).
Triassic and Early Jurassic faults (230–200 Ma) outlined an emerging Brittany and highlighted a clearly pre-Biscayan rift along the southern Armorican margin. The Western Channel Basin was aligned with the Late Jurassic North Atlantic Ridge (Hallam, 1971), extending to the southern North Sea. A regional E–W major extension occurred from 170 Ma with an accelerated phase of rifting (Müller et al., 2008), followed by the mid-Atlantic opening (Calvès et al., 2012; Greiner and Neugebauer, 2013) that led to the mid-Cretaceous inversion (125–100 Ma, Aptian–Albian). This last phase of rifting (Delanoë, 1988; Bois et al., 1994; Thinon et al., 2001) led, in the west, to an inversion of the entire English Channel, including the Weald Basin, with the reactivation of the N150 and N180 fault zones. The mid-Atlantic rifting began to slow down from 120 Ma, with the oceanic break-up then propagating eastward, into the Bay of Biscay (Martín-Chivelet et al., 2019), accompanied by extreme lithospheric thinning with an ENE–WSW extension trend (Thinon et al., 2001; Jammes et al., 2010). This event created a half-graben along Armorica (Delanoë, 1988; Bois et al., 1994), with the southern Armorican shelf behaving as a passive margin (seismic lines SWAT 6–7) (Thinon et al., 2001) with the continuation of the inversion and the reactivation of the en-echelon strike–slip faults of the AUFZ and MCFZ systems along the Western Approaches and the western English Channel (Figures 1, 2) (Jammes et al., 2010; Le Roy et al., 2011).
Renewed acceleration of the mid-Atlantic spreading rate developed between 120 and 84 Ma. Post-rift thermal subsidence of the southern Armorican margin began along the Bay of Biscay between 80 and ∼65 Ma in the Late Cretaceous, in association with a renewed slowing down of mid-Atlantic ridge formation. A third regional phase of inversion and an S–N extension occurred between 95 and 65 Ma (i.e., the Laramide tectonic phase) (Bergerat, 1987), which accentuated the relative emersion of the rigid Cadomian basement of Armorica. The relief created allowed the erosion of Mesozoic saprolites (Wyns, 1991) and the deposition of Cenomanian sands to the east of Armorica.
The morphology of Armorica attests to a major shaping issuing from the second main inversion, which deformed the post-Hercynian peneplain, mostly from ∼110 Ma. At this stage, most of the coastlines were already close to where they are now (Renouf, 1993), except for relief and flooding. Pre-existing Jurassic faults were again reactivated and remained the basic control system for Neogene and Pleistocene seismicity.
3.2 The Cenozoic
3.2.1 Paleogene and Neogene regional evolution
A major NW–SE to N–S shortening phase took place, related to the Pyrenean Orogeny that occurred in Western Europe during the Eocene (from 48 to 43 Ma) (Hillis, 1995; Parizot et al., 2021). An important and fourth inversion occurred a little after the Bartonian (40–39 Ma), with a renewed reactivation of the old Permo-Triassic N150 fault systems (Wyns, 1991; see the synthesis in the work of Ziegler and Dèzes (2007) and Le Roy et al. (2011)). The Bartonian crops out along the coasts of Brittany, in the Seine Estuary (Benabdellouahed, 2011) (Figure 8A). The Hampshire–Dieppe Basin mostly subsided during the Bartonian (Paquet et al., 2023). Subsidence of the southern Armorican margin along the Bay of Biscay accelerated from the Eocene, as also in the Western Channel (Andreiffe Evans and Hughes, 1990; Lericolais et al., 1995; Le Roy et al., 2011), with major fault activity taking place in the MCFZ. In the southern Rennes Basin, sedimentation began at ∼40 Ma (Bauer et al., 2011).
A change in stress direction occurred in the very late Eocene (∼35 Ma) (Martinez et al., 2020), with the opening of the NE Atlantic, synchronized with the late Alpine phase. This E–W extension, related to the North Atlantic widening, allowed the development or enlargement of rifts across Europe (Bergerat, 1987; Cloetingh et al., 2008). In Armorica, the inversion led onshore, to the reactivation of Permo–Triassic N180 and N130 faults, creating a series of early Oligocene pull-apart basins in Brittany, such as those along the Quessoy–Nort-sur-Erdre Fault Zone (Figure 4) or the Western Channel and Western Approaches (Figure 1B). Basin subsidence continued generally at that time in the western English Channel (Figure 2), the Hampshire–Dieppe Basin (see the synthesis in Paquet et al., 2023), and the North Sea (Vandenberghe, 2017). The following Oligo–Miocene inversion in Armorica (∼30–23 Ma) uplifted The Hague, Jersey, Central Brittany, and the Iroise Plateau horsts (Ushant Islands). Onshore, the horsts in Brittany were overall transpressively uplifted from the Chattian (23 Ma) (see the synthesis in the work of Guillocheau et al. (2003)) as also offshore from seismic lines (Le Roy et al., 2011) (Figures 1A, 4).
During the Oligo–Miocene, the shortening shifted to N150 (Jura Orogeny), regionally limiting the inversion process during the transient transpressive doming of Western Europe. An NE–SW shortening developed 18–16 Ma ago, as recorded in the Alps (Besson et al., 2005; Stoker et al., 2005) and in the northern Pyrenean Foreland (Parizot et al., 2021)—a feature related to the shaping of the Straits of Gibraltar (Civiero et al., 2020). A limited relaxation in this doming developed in Armorica from the Langhian (see the synthesis in the work of Van Vliet-Lanoë et al. (2002) and Guillocheau et al. (2003)), with marine invasions occurring regionally (Durand, 1949; Bauer et al., 2016).
From the Late Miocene (14 Ma), the shortening shifted back to an ∼N170 direction (Bergerat, 1987; Heidbach et al., 2016), with a continuation of the Gibraltar plate convergence. This allowed regionally some Messinian doming and a renewed, albeit limited, uplift of horsts, such as the Iroise Plateau, alongside further subsidence in basins, such as the Rennes (Bauer et al., 2016) and Cotentin (Van Vliet-Lanoë et al., 2002). The last traces of fault ruptures were recorded in Armorica. Glaciations already occurred frequently at that time leading to some stratigraphic confusion in Normandy with the Plio-Pleistocene cooling.
During the Zanclean and Piacenzian, from ∼5 to 4.2 Ma in Armorica, relaxation and disappearance of the Messinian doming allowed the deposition of tidal clays in the Elorn Valley (Darboux et al., 2010) and of the main Red Sands, ESR-dated. These usually infilled pre-incised Tortonian to Messinian paleovalleys (Van Vliet-Lanoë et al., 1998a; 2002) were also deposited in a large gulf, the Carentan/Cotentin Basin (Dugué et al., 2009). From the very Late Pliocene (from 3.2 Ma) to the Gelasian (2.5–1.8 Ma), Central Brittany was slightly uplifted due to the onset of Early Pleistocene doming with valley incision (Van Vliet-Lanoë et al., 2002), adapted to the N130–N150 fault sets of the Armorican Massif (Bonnet et al., 2000). Transtensive N150 subsidence of the basins continued, especially during the Gelasian, as evidenced by red–pink estuarine clays issued from eroded Miocene red–yellow podzolic soils in response to recurrent periglacial erosion. In the Western Channel, no fault reactivation has been observed along the MCFZ (Bourillet et al., 2003), which is also true in the Gulf of the Seine (Benabdellouahed, 2011).
The limited Cenozoic inversion events acting on Armorica seem to have corresponded to both Alpine orogenic events and periods of slowing along the mid-Atlantic Ridge (Figure 7). This slowing occurred due to the mantle being dragged by the gravitational stretching of the solid overlying crust (Mosar et al., 2002), possibly all the way to the Alpine and Carpathian subduction fronts (Worum and Michon, 2005; Ziegler and Dèzes, 2007).
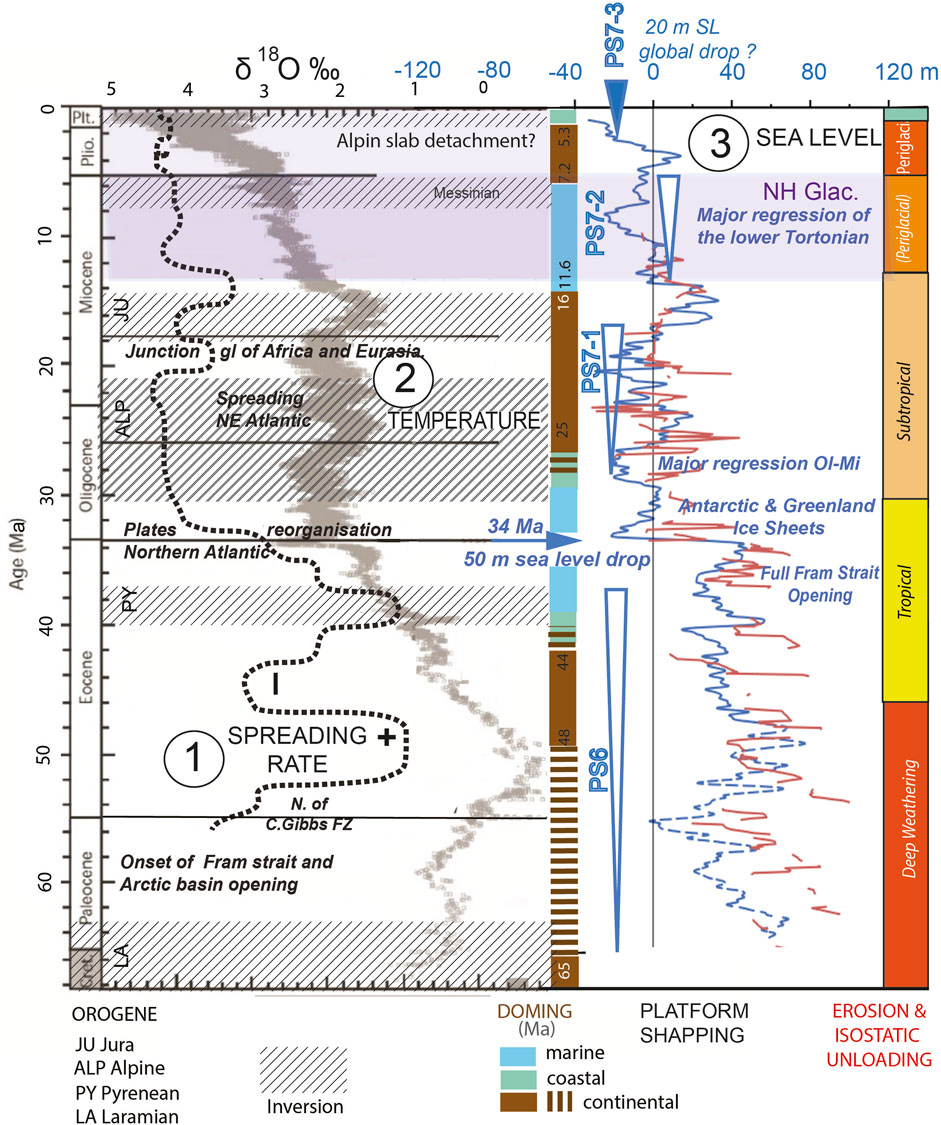
FIGURE 7. Geodynamic and climatic evolution of the central Atlantic during Cenozoic oceanic spreading and platform shaping (the Plio-Pleistocene is not detailed). (1) Mid-Atlantic spreading rates derived from the work of Le Douaran et al. (1982) and Gaina et al. (2017). (2) Isotopic temperature curve from the work of Zachos et al. (2001). (3) Sea level (blue and brown curves) according to the work of Miller et al. (2020). The two columns in the center reflect the doming episodes (in brown) and the shaping phases of the platforms (arrows). See the text for the nomenclature. The last column is a global record of climate and weathering (Durand, 1949; Wyns, 1991).
3.3 Sea level, geodynamic trend, and the history of the Atlantic coastal platforms
3.3.1 Global sea level and climate
The evolution of the global eustatic signature (Miller et al., 2020) on long timescales indicates that the tectono-eustatic component has not decreased by more than 50 m since 60 Ma (Rowley, 2013; Ogg et al., 2016) and is primarily the result of variations in ocean-floor production. Sea level is also considered stable in volume under the control of plate tectonics (Rowley, 2013; Cloetingh and Haq, 2015; Cramer et al., 2019) and under the thermal contraction of the upper portion of the oceanic mass (Purkey et al., 2014). Glacial storage on continents, based on the present-day ice extent, represents an ∼80 m SL drop (Delaygue et al., 2001). This can be used as a reference tool for defining the deformations affecting continental margins or coastal platforms.
Our paleosurface/platform nomenclature completes that of Bessin et al. (2015).
A first Cenozoic SL fall (estimated to be ∼30 m) occurred from ∼44 Ma (Figure 7) (Ogg et al., 2016; Miller et al., 2020) following the regional onset of relaxation after the last phase of the Pyrenean Orogeny in Europe and the worldwide initiation of glaciations in both hemispheres. Decreasing second-order steps in the global SL drop resulted from ice-sheet storage from the Oligocene, as highlighted by the oxygen-isotope record (Lisiecki and Raymo, 2005; Zachos et al., 2001; Cramer et al., 2009) (Figures 3, 4), in association with lifted reliefs (Japsen et al., 2014). A second global SL fall, estimated at another 20–30 m (Ogg et al., 2016; Steinthorsdottir et al., 2020), occurred at 37 Ma in relation to plate reorganization in the North Atlantic (Gaina et al., 2017; Martinez et al., 2020). These were synchronous with the progressive opening of the Fram Strait and the onset of the main Alpine Orogen. A general cooling resulted from ocean-spreading activities (Figure 7) in tandem with major late Eocene ice storage on Eastern Antarctica and Greenland at 37–35 Ma and 34–32 Ma, respectively (Figure 7) (Eldrett et al., 2007; Schouten et al., 2008; Tripati and Darby, 2018; Xia et al., 2021). This period led to a step-like resurfacing of the main regional surface (PS6) in association with a ∼50 m global SL fall (Miller et al., 2020).
The Greenland inland glaciers developed from 34 Ma onward (Table 1) (Tripati and Darby, 2018). The true Nordic ice sheet that reached the coast has been recorded on eastern Greenland’s passive margin at close to ∼9 Ma only (Table 1). Seven worldwide climate cooling phases (at 14, 11.8, 9.0, 6.7, 5.9, 5.5, and 4.7 Ma) have been recorded along the Miocene (Herbert et al., 2016; Ogg et al., 2016; Xia et al., 2021). The major Pleistocene cooling began at 3.2 Ma alongside the beginning of the Pleistocene uplift of the North Atlantic margin (see the synthesis in the work of Zachos et al. (2001) and Japsen et al. (2014)).
The maximal Neogene extent of permafrost seems to have occurred around 6–5 Ma in Eurasia, according to Gilichinsky et al. (2007). It is thus possible that periglacial and sea-ice abrasive activities (Hallégouët and Van Vliet-Lanoë, 1989) developed regionally, at least from the Upper Miocene. Also, the normal to “pseudo-reverse” superficial faulting observed in Middle Pleistocene sands in eastern France revealed it to be of thermokarstic origin (Van Vliet-Lanoë et al., 2017). Faults with an offset that increases toward the surface should correspond to a collapse with thaw ice body consolidated in drained sands (valley side), as at St Vigor (Cotentin Basin), in the Rennes and Reguigny basins (Figure 6). Converging figures occur on karstified chalk at La Londe and Valmont (Seine Estuary). As regional periglacial activity set in from the Upper Miocene, it is possible that permafrost—at least in a discontinuous form—had already occurred early in Brittany and the Cotentin Basin (Figures 6, 7).
3.3.2 Sea level and regional platform shaping
Climate cooling and SL falls created the conditions for at least two main platform reshaping occurrences from the pre-Oligocene pediplanation surface (PS6 ∼+100 m) and the Late Paleogene polygenetic surface (PS7–1) close to ∼+50 m onshore (Figure 7) (Ogg et al., 2016), corresponding to the deeply weathered +50 m terrace in the Cap de la Hougue (Figure 8) despite an attribution to the Middle Pleistocene (Coutard et al., 2003). Cooling with falling global SL continued after 38 Ma, reaching the present HS level and resulting in a polygenetic Neogene platform (PS7–2 and 3) (Figures 7, 8). The shaping of this surface along the Atlantic margins generally accelerated during the Neogene because of a pulsed spreading of the North Atlantic Ridge, offshore storminess, and sea-ice abrasive activities since the onset of the glaciations (Mosar et al., 2002; Japsen et al., 2014; see the synthesis in the work of Van Vliet-Lanoë and Guillou (2021)).
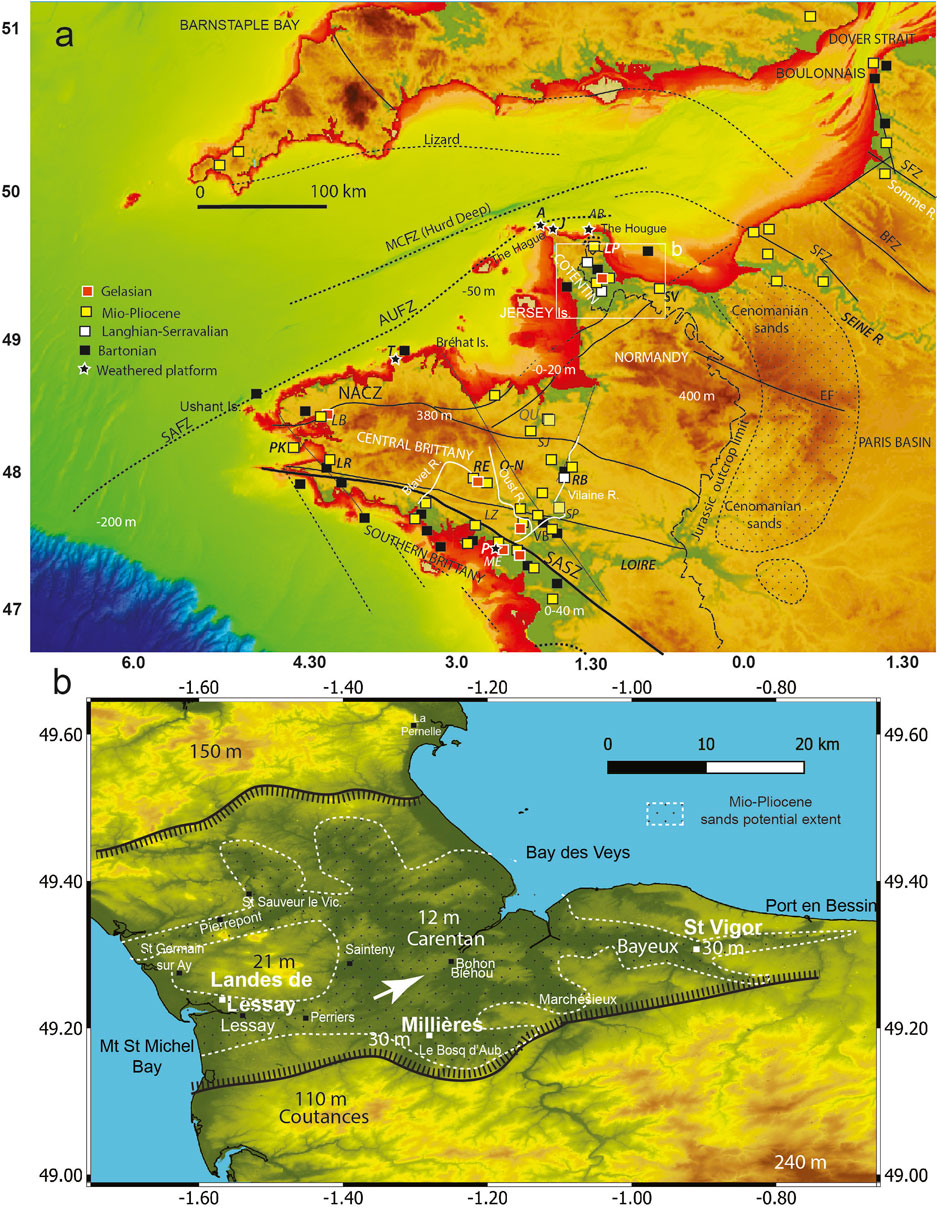
FIGURE 8. DEM. The rigid crustal bodies are uplifted in horsts. (A) Extensive coastal platforms bordering the Armorican Massif (in red) (HOMONIM project digital elevation model). (B)) Close up of the Cotentin Basin (in green), St Vigor Basin (SV), Rennes Basin (RB), and topographical control of the complex SACZ in limiting the continental margin of the Gulf of Biscay and inland basins, such as the Rennes Basin, which is also controlled by the Quessoy–Nort-sur-Erdre (QN) N150 fault.
3.3.2.1 PS6
The overall morphology of the Armorican and English Channel systems already existed before the late Cenomanian doming (Renouf, 1993; Wyns et al., 2003; Bessin et al., 2015). The first main platform (the Armorican PS), here referred to as PS6, extended to ∼+100 m (Durand, 1949; Delanoë et al., 1976; Baize, 1998; Ziegler and Dèzes, 2007; Bessin et al., 2015). Long-term, low-amplitude, transpressive doming during the Paleogene resulted in limited uplift, which led to the emergence of Armorica and further pediplanation, under subtropical subaerial weathering, that was partly inherited from previous emersion episodes (Durand, 1949; Wyns, 1991). The PS6 shaping ranged from at least the base of the Cenomanian (∼100 Ma) to the late Bartonian or early Priabonian (37 Ma) (Figure 7). Late Eocene and early Oligocene transtension wrenched the area (Durand, 1949; Duperret et al., 2016), resulting in faulting in the Bartonian (Lefort, 1975; Delanoë et al., 1976; Benabdellouahed, 2011; Paquet et al., 2023). Delanoë et al. (1976) observed that the “marine Oligocene lapped unconformably on a deformed and faulted Upper Eocene (<40 Ma), being separated from Aquitanian deposits (23 Ma) by a younger abrasion surface platform” described here as PS7–1 (Figure 7), resulting from the truncation of several meters of a deep saprolite.
A: Audierne platform; AB: anse du Brick; J: Jardeheu; LD: Landerneau (LD), LZ: Lauzach; LR: Le Rhys; ME: Mesquer; P: Penestin; PK: Porz Kubu; QB: Quiou Basin; SJ: St Jouan, SP: St-Malo-de-Phily; SV: Vigor Basin; RE: Reguigny, RB: Rennes Basin; T: Trégastel, MCFZ: Middle Channel Fault Zone, AUFZ: Alderney–Ushant Fault Zone, NASZ: North Armorican Shear Zone, and SASZ: South Armorican Shear Zone.
Offshore of Pénestin, as in most of southern Brittany, this PS6 platform is partly flooded close to the present-day SL today, south of the SASZ, dipping toward the Bay of Biscay and the Western Channel (Figures 8, 9) in relation with the post-rift thermal subsidence.
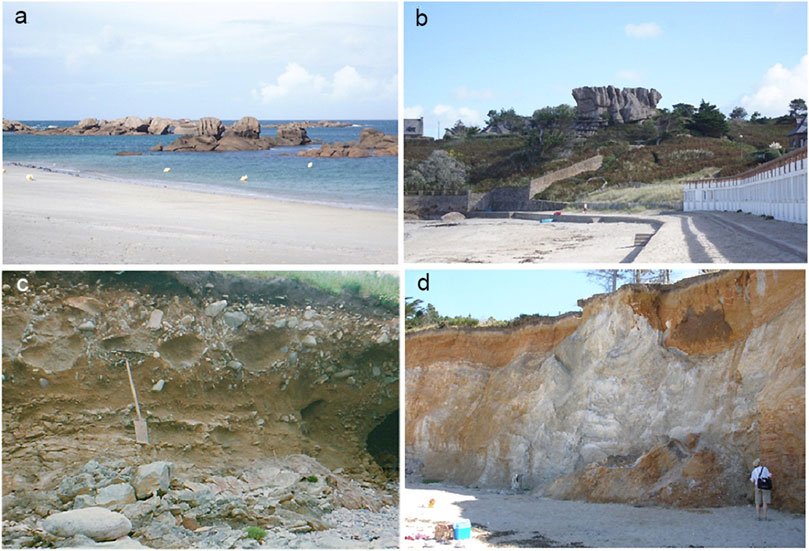
FIGURE 9. (A, B) Weathered platforms—the (A) lower PS7–1 and (B) higher PS6–2 (+35 m)—in the weathered Ploumanach Granite (Trégastel). (C) Deeply weathered platform (indicated by gibbsite) with deformed cobbles at Jardheu Point (northern Cotentin), intermixed at the top with MIS 5 cobble beaches (from pedostratigraphy). (D) Deformed and faulted saprolite on gneiss at Pénestin (Mine d’Or), reshaped by a Late Miocene LS (6.7 Ma) and Early Pliocene Red Sands (LS), truncated by Gelasian clays, and by a 400-kyr-old tidal channel and a 300-kyr-old LS periglacial alluvial terrace (see Supplementary Material) (images: Van Vliet-Lanoë).
3.3.2.2 PS7–1
The PS7–1 lower tidal platform (Figure 7) was most probably shaped between 33.9 Ma (Glaciation O1) (Ogg et al., 2016) and 28 Ma (Early Miocene). This surface persists today all along the low, coastal peri-Armorican Platform (Figure 8A), where it is often, from our observations, delimited by a degraded fossil paleocliff (10–40 m high). This corresponds to the region in Brittany known as the “côte à écueuils” (Battistini and Martin, 1956), which has been mostly cleared from the clayey saprolite to the north of Armorica. This platform at Trégastel (Figure 9) exhibits reefs bearing large “piped” weathering scars on a +5 m NGF platform bounded by a deeply weathered paleocliff at +35 m height. This also occurs from our observations along the north-western Cape de La Hague, on the wide Auderville weathered platform, on the weathered-boulder paleobeach at Jardeheu (gibbsite in the beach matrix (Van Vliet-Lanoë, 1987), and on the Anse du Brick, a consolidated and weathered raised beach ridge (east of Cherbourg) (Figure 1B; Figure 4A; Figure 5), all of which were further reshaped during the Middle Pleistocene (Van Vliet-Lanoë and Guilloou, 2021).
3.3.2.3 PS7–2 and PS7–3
The platform shaping along the Atlantic margins, thus, accelerated during the Neogene and Pleistocene due to doming/uplift events and occurred alongside the formation of glaciers during the onset of the glaciations (Japsen et al., 2014; Van Vliet-Lanoë and Guillou, 2021).
Partly emerging (doming and weathering) between 28 and 21 Ma, PS7–2 was, during the early LSs, related to the Miocene glacial events (between 23 Ma to 10 ka) (Ogg et al., 2016), sometimes flooded and following us, efficiently wave-abraded, supplemented by early sea-ice action and periglacial activities (Figure 7). Plate motions, such as the Middle Miocene closing of the Balboa (Montes et al., 2015) and Gibraltar straits (Krijgsman et al., 2018), also occurred at this time.
The last platform reshaping (PS7–3) started to develop during the Late Miocene LSs (14–6.7 Ma), as recorded at the base of the Cockburn Formation in the Western Approaches (Evans et al., 1990; Le Roy et al., 2011). The onset of PS7-3 occurred in Armorica with re-flooding of the onset of the Langhian–Serravallian relaxation and in the Tortonian, between 14 and 9.0 Ma (Van Vliet-Lanoë et al., 2002; Guillocheau et al., 2003), being interrupted by Upper Miocene glaciations (Herbert et al., 2016; Ogg et al., 2016). These coolings were recorded here by ice-rafted blocks and probably sand wedges as far south as Pénestin (Van Vliet-Lanoë et al., 2009). This continued into the Pliocene, with a renewed glacio-isostatic SL fall of ∼30 m (Figure 3) (Miller et al., 2020), with the same geometry and altitude as the Middle Pleistocene to present-day coastal HSs (Van Vliet-Lanoë et al., 2002). These Neogene and Pleistocene cooling stages also resulted during LS in the incision of inland Western European valleys, first down to the base of a Late Miocene Middle Terrace (Van Vliet-Lanoë et al., 2002), as also recorded offshore along the subsiding southern Amorican margin (Van Vliet-Lanoë et al., 2009; Paquet et al., 2010).
During the Plio-Pleistocene, the worldwide marine HS is considered to have always been close to that of the present day (±10 m) (Miller et al., 2020). The increase in the amplitude of glacial–interglacial cycles from 700 ka marked an increase in the severity of glaciations and, thus, an increased ice storage during LSs, the Middle Pleistocene Event (MPE) of the work of Clark et al. (2006) (Table1) with an apparent drop of approximately ∼50 m for LSs in the decreasing global SL due to a major glacial event, the MIS 16 (Van Vliet-Lanoë et al., 2021; Hodell et al., 2023). The strongest glaciations attested to by the marine oxygen-isotope (MIS, based on δ18O) records were MISs 10, 12, 16, and 20 (Lisiecki and Raymo, 2005, 2016), but possibly also due to ice storage in the permanent permafrost in the NH (Van Vliet-Lanoë et al., 2024 accepted).
Data from western Iceland attest to the initial shaping of the now-flooded (to approximately 100 m deep) PS7–1 surface during the long stable stand from the O1 Oligocene glacial LS (∼33 Ma) (Liebrand et al., 2011; Ogg et al., 2016; Xia et al., 2021) (Table 1; Figure 7). This surface was drowned by thermal subsidence after the formation of the Iceland Plateau around 20 Ma (see the synthesis in the work of Van Vliet-Lanoë and Guillou (2021)). The youngest peri-Icelandic surface, thus, developed between ∼15 Ma (Iceland isolation, from K–Ar dating) and the recent times. This seems valid for the entire North Atlantic coast (Mosar et al., 2002; Japsen et al., 2014). This PS7-1 surface has been partly abraded by platform glaciers, forming a strandflat, but had commonly already been deeply scoured by ice streams that surged from a ∼9 Ma former fluvial system (Table 1) (Mosar et al., 2002; Van Vliet-Lanoë and Guillou, 2021). This indicates that the Late Miocene–Pleistocene platform reshaping in Brittany was not an exception along the passive margins of the Atlantic Ocean.
To summarize, PS6 recorded the events in Armorica from at least the Late Cretaceous through the late Eocene, despite doming relaxation and the onset of ocean cooling. PS6 has commonly been faulted and deformed before the Bartonian, onshore as well as offshore (Andreieff et al., 1972; Andreieff and Lefort, 1972; Béchennec et al., 2012). The PS7–1 platform was reshaped between 34/28 and 21 Ma, after the major SL drop (estimated here to approximately −30 m) related to plate tectonics and glaciation, and from 14 to 1.2 Ma by the LSs (PS7–3) associated with progressive tectonic doming from 9 to 5.3 Ma (Messinian Event). The PS6 paleocliffs were eroded retrogressively from PS7–1 to PS7–3, with the formation of steps, notches, coastal caves, and periglacial slope deposits (screes) as at the coast at Porz Kubu (Crozon peninsula) and Le Rhys (Douarnenez), further stained during the Tortonian by a Fe-Mn ortsein (Figures 3 and 10) as at Pénestin. The PS7–3 coastal platform and cliff reshaping developed from the Early Miocene (∼11 Ma) as an LS, reactivated in association with a potential relaxation of the Messinian doming. From 700 ka, after an apparent SL drop of 30 m based on fluvial archives (Middle Terrace), a renewed reshaping of the PS7–3 platform took place as HSs at least eight times based on dated coastal deposits (Van Vliet-Lanoë et al., 2021 and 2024).
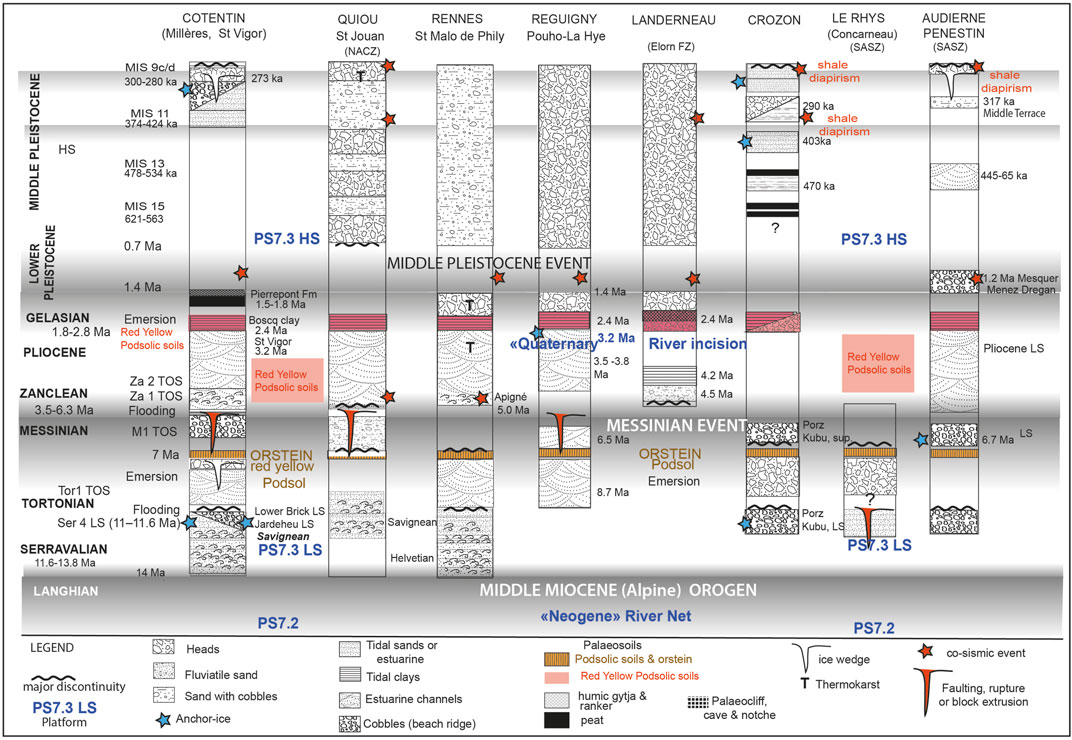
FIGURE 10. Global stratigraphy of the sedimentary, tectonic, and climatic record for Armorica. The doming intensity is marked in gray.
3.3.3 Coastal and fluviatile indices for discrete doming events
Regionally, mostly fluviatile onshore Upper Miocene HS deposits apparently reached 80–100 m in central Brittany and northern Cotentin, as on the uppermost terrace of the Oust River (Le Pouho-La Hye), and in the Réguiny Quarries (Blavet River) (Table 1) (Van Vliet-Lanoë et al., 1998b; 2002). This has led to the attribution of these deposits to continuous tectonic uplift (Coutard et al., 1991; Bonnet et al., 2000; Pedoja et al., 2018) or to a transient doming linked to tectonic pulses transmitted at the boundary of the Europe–Africa convergence (Alvinerie et al., 1992; Van Vliet-Lanoë et al., 2002; Guillocheau et al., 2003; Dugué et al., 2009). Successive transient doming events (Burdigalian–Langhian, Messinian, and Late Pliocene–Middle Pleistocene) caused bulging in Armorica, interrupted by relaxation episodes (early Tortonian, Zanclean, Late Pliocene, and possibly, Middle Pleistocene) (Figure 10) (Van Vliet-Lanoë et al., 2002; Guillocheau et al., 2003).
3.3.3.1 Evidence of the Messinian doming and tectonism in Armorica
In the Quiou quarries (NASZ) (Figure 10), the Savignean crags, including an orstein, were N150 faulted before the deposition of the “Red Pliocene Sands” (Néraudeau et al., 2003; Néraudeau, 2007). The base of these Tortonian crags (Biagi et al., 1996) yielded an age of 11 Ma based on 87Sr/86Sr at Doué-la-Fontaine and Savigné (Loire River) (Barrat et al., 2000), indicating the end of the Ser 4 LS (11–11.6 Ma)—a third-order sequence (TOS) (Hardenbol et al., 1998; Miall, 2010).
At Pénestin (Figure 2), in the Vilaine estuary incised into the weathered basement to +4 m NGF, an old goethite-cemented cobble deposit (orstein) recorded ghosts of Fenestellidae bryozoans. This suggests locally an early Tortonian LS close to 11.6 or 9 Ma (Van Vliet-Lanoë et al., 2009). Onlapping this orstein is a frost-jacked cobble beach with ice-rafted blocks at +6 m NGF. This “cold” beach yielded an ESR date of 6.7 Ma, equivalent to the first Messinian cooling event (Van Vliet-Lanoë et al., 2009; Herbert et al., 2016; Van Vliet-Lanoë et al., 2019). Here, a transgressive Pliocene estuarine channel (Red Sands) has lapped onto the dated LS (Van Vliet-Lanoë et al., 2009), finally onlapped by Gelasian tidal red clays (Figure 10).
This is clearly visible nearby at St Congar (+30 m NGF), on the Oust River, incising the already uplifted SASZ (Figure 8): a Tortono–Messinian Middle Terrace is consolidated by orstein. This terrace is buried by Gelasian rubified screes as also at the coast for the Tortonian to Gelasian shore face complexe record at Pors Kubu. The base of the Pliocene upper Red Sands in the Rennes Basin was ESR dated at ∼ 5.35 Ma (+50 m in Apigné Quarry) (Van Vliet-Lanoë et al., 1998a; Van Vliet-Lanoë et al., 2002; Bauer et al., 2016).
To synchronize the Brittany and Normandy geodynamical events, we reanalyzed in the field (1995) the stratigraphically of the poorly time-constrained Millières core (1991) in the Cotentin Basin (Figures 8A,B; Table 1; Supplementary Table SA), Figs. 12, 11, 14), considered to be Plio–Pleistocene in age (Clet pellerin et al., 1997; Baize, 1998; Dugué et al., 2000; 2009; Paquet et al., 2023). Our reanalysis in the field (1995) of the lower Millières Formation (Fm) compared with the fair description of Dugué et al. (2000), re-using the cold-water foraminifera data (see Millières discussion in Supplementary Figures S11, S12, S14)), resulted in a cool Tortononian signature, as for the lower Savigean/Tortonian crags (Courville and Bongrain, 2003), and the faulted Savignean Quiou crags, in Northern Brittany (Figure 10). Normal faults are locally pre-stained with orstein in the lower Millières Fm at Périers (see image NOR01-3 in the work of Baize et al., 2002), and Lessay, locally on crags, suggested a Tortonian cold age for it as shown in the field by some ice-rafted blocks observed near the top. The lower Millières Fm may potentially fit the 11-Ma cooling equivalent to the base of the LS tidal channels of the Loire River (Barrat et al., 2000) or the 6.7-Ma cooling and LS dated at Pénestin (Van Vliet-Lanoë et al., 2009).
Thus, the lower Millières Fm should correlate with the Tor1 TOS, as at Réguigny (>7.0 Ma). Various such faults were further reactivated as periglacial sand wedges (Supplementary Figure S10), as the Lessay tidal sands recorded a sand wedge generation. Regionally, this implies some Late Miocene coastal periglacial activity before and after the orstein formation (Supplementary Material).
The middle Millières Fm correlates then with the Piacenzian in the St Vigor Basin, in conformity with the dating obtained there (Figure 2) from the upper Red Sands (∼3.2 Ma) (Van Vliet-Lanoë et al., 2002). This should correlate with the 6.7 (M1 TOS) (Hardenbol et al., 1998; Ogg et al., 2016), 5.4 (M2 TOS), and 4.7 Ma (Za1 TOS) glaciations and the Elorn River record. The orstein should, therefore, signify the Tor3 TOS (LS) (∼7 Ma).
A similar situation existed to the NE, outside of Armorica, on the Northern Hercynian Front at Wimereux (Boulonnais, Figure 4A; Van Vliet-Lanoë et al., 1998b and 2010), where there is an LS beach (+6 m NGF) from <∼11 Ma, stained with orstein, similarly to the nearby faulted or Diestian (Messinian) goethithic coastal ridge (∼+100 m at Cap Blanc Nez) (Vandenberghe et al., 1998; Vandenberghe, 2017) and Aldershot (Weald) (Van Vliet-Lanoë et al., 2002). Here, the faulted glauconitic sand probably records the HSs at +30–35 m. The Wimereux orstein LS is laterally onlapped by Pliocene LS estuarian red sands, (∼3.67 Ma at +22 m), which rest directly on faulted Bartonian–Rupelian (Van Vliet-Lanoë et al., 2010). This record is very similar to those of the Rennes Basin (Bauer et al., 2016) and the paleoestuary of the Blavet River.
The eustatic amplitude between the Messinian LSs and HSs suggests ∼25–30 m as on the Hardenbol et al. (1998) eustatic curve. In Brest Bay, the Elorn Estuary (Figure 4A) (Darboux et al., 2010) records the onset of the post-Messinian relaxation, corresponding to the first warm transgression in the Zanclean following cooling events at 5.5 and 4.7 Ma (Herbert et al., 2016; Ogg et al., 2016) and the Za1 TOS. A second major climate cooling occurred here, with aridity between 4.1 and 4.0 Ma in the paleontological record at Landerneau (Za2 TOS). Following this, transgressive clay containing boreal vegetation (Morzadec-Kerfourn, 1982) was deposited, indicating an early Gelasian Upper Terrace at +25 m NGF with a basal ESR date of ∼2.42 Ma (Laurent, 1993).
3.3.3.2 Evidence for Pleistocene doming events
The first evidence for incised Pleistocene rivers was given by the Oust River High Terrace at the Le Pouho-La Hye site (+68 m NGF), dated at 3.05 ± 0.5 Ma, after the onset of cooling at 3.2 Ma (Ogg et al., 2016). This relatively recent river network is still immature as also the near Blavet River (Van Vliet-Lanoë et al., 1998b), reworking the older Mio–Pliocene one as also for the Cape of La Hague (Pedoja et al., 2018). This suggested an ongoing uplift, not necessarily of tectonic origin.
3.3.3.3 Inactive Plio-Pleistocene tectonic “grabens” or Mio-Pleistocene large tidal scouring?
The absence of visible offshore ruptures in the confluent and Hurd Deep zones of the Central Channel (MCFZ) (Bourillet et al., 2003) or in the Raz Blanchard or Hague Deep (Furgerot et al., 2019; Paquet et al., 2023) demonstrates that the region is still exclusively erosive today, with no fault reactivation (Figure 12). The closure of the seaway in the western Cotentin area (Figure 8B) during the Oligocene was an important benchmark in the evolution of the region: the difference in the tidal range between the “Normano–Breton” Gulf (Lessay) and the Seine Bay (Baie des Veys) is currently approximately 6 m, (Service hydrographique et océanographique de la Marine [SHOM] 2005). This suggests that, during the Neogene from ∼11 Ma, the Cotentin Basin formed a flooded paleovalley system (Figure 8B), evolving into a Gulf during HSs with the described deep tidal scours, flasers, and current megaripples (Dugué et al., 2009), as seen today in the Rhine Delta (−50 m). The Plio-Pleistocene Marchésieux, Le Boscq, and St Nicolas de Pierrepont “tectonic grabens” (Clet-Pellerin, 1983) (Figure 8B) were only investigated in cores, representing tidal channel infillings guided by late Eocene reactivated faults.
Similar successions shaped in the English Channel the submarine Hurd Deep and Confluent zones (dominated by floods due to dam destruction) (Figure 11) (Gupta et al., 2007) and the Hague Deep north of Cotentin dominated by tidal currents (Figure 12) (Furgerot et al., 2019). This scouring adaptation to the basement structure is also evident for the Mio–Pliocene Lauzach Basin (Southern Brittany; Figure 8A), a box-like one, with saprolites blocks limited by fossil faults, further cleared by tidal currents, and then infilled with Red Sands (Van Vliet-Lanoë et al., 2002). Tidal scouring also seems plausible for the St Vigor Basin in Normandy (present tidal range 6 m, Supplementary Figure S11), which is constrained by pre-existing Jurassic faults, and for the N150 Rennes and St-Malo-de-Phily basins, which have experienced regular marine invasions since the Bartonian (Bauer et al., 2016).
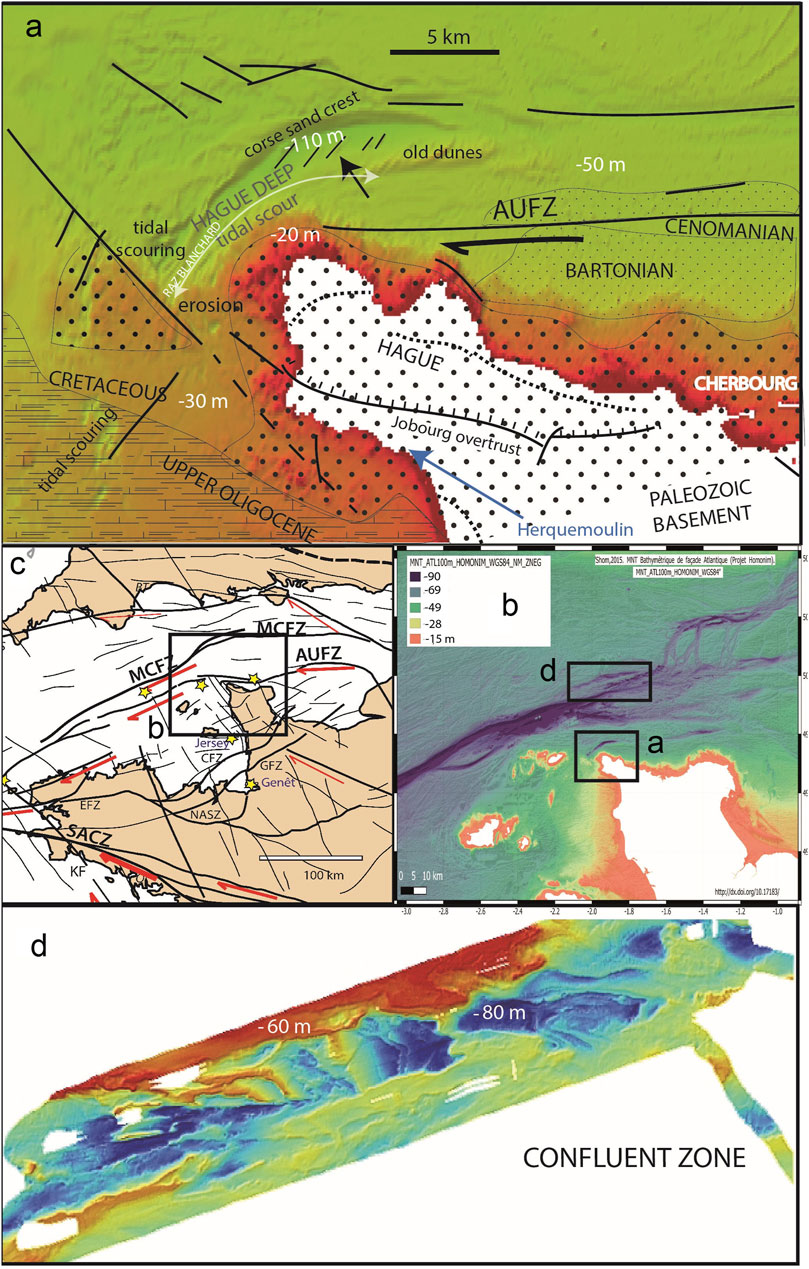
FIGURE 11. Central English Channel. (B–C) location of the studied features. Erosional scours and sediment accumulations along (A) the Hague Deep with the Raz Blanchard tidal current and (D) the Hurd Deep Confluent Zone. Dotted lines represent paleocliffs. Images are from the HOMONIM project (Service hydrographique et océanographique de la Marine). Erosion revealed and enhanced inactive Jurassic faults in the Mesozoic basement and large plunge pools (image D, right), resulting in differential erosion by both tidal currents and giant floods generated by dam rupture (Dover Strait).
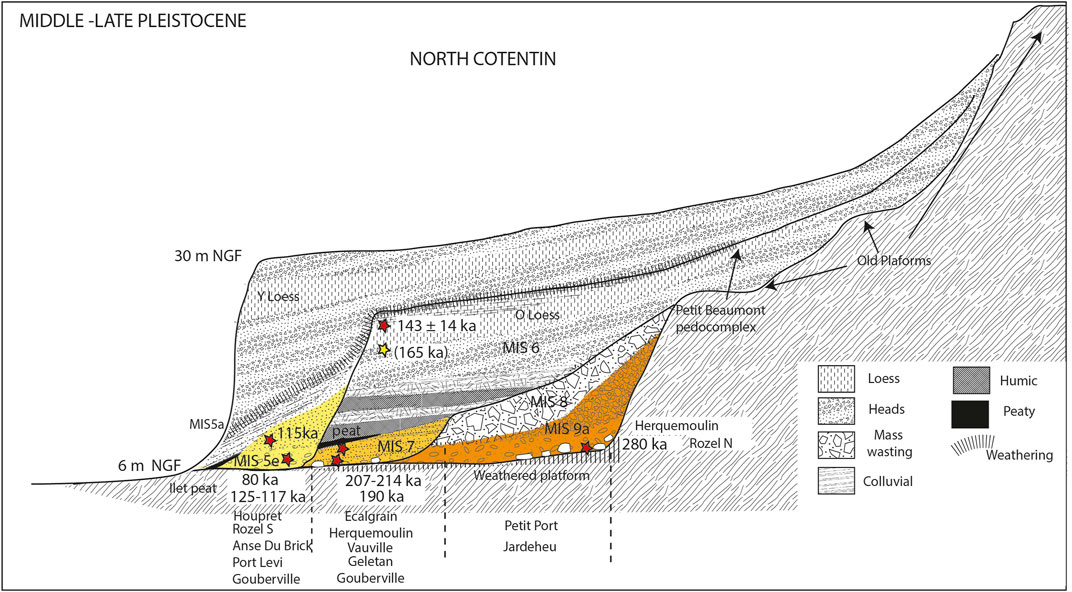
FIGURE 12. Geometric synthesis of the raised beaches and Herquemoulin cliffs (conform shale dip) in western Cotentin, presumably inherited from Platform PS7–2. The upper cliff probably derived from PS7–1, with isostatic accommodation since the onset of the major glaciation at 11 Ma.
The Hague overthrust, which parallels the AUFZ, has been considered to have been reactivated as the ‘Jobourg Fault’ during the recent Quaternary. Incidentally, at Herquemoulin (northernmost Cotentin) was considered as an active N150 fault scarp (Lagarde et al., 2000; Font et al., 2002) (Supplementary Figure S8). In the nearby Ecalgrain Bay, the low platform crossed by this Paleozoic Fault does not show any recent displacement. Its last erosional but non-tectonic reactivation took place during late MIS 7, ∼190 ka (Cliquet et al., 2009; Figure 12), although it is probably older given the weathering of the buried platform at the nearby Petit Port (1 km north of the Herquemoulin site). They were only reshaped as an old marine cliff (possibly of the Oligocene age), adapting it to the dip of metamorphic shales or by tidal currents only (Supplementary Figure S8). The Hague “fault” and the Hague Deep do not show any Plio-Quaternary reactivation offshore.
This should correspond to the Late Miocene cliff related to the PS7–3 platform or to the weathered platform at Menez Dregan (Audierne Bay), which is older than >1.2 Ma (ESR dating in the work of Monnier et al. (1994)) and further reactivated close to 400 ka (Van Vliet-Lanoë and Guillou, 2021).
The only potential source of deep seismicity in Normandy should be the ∼N150 late Hercynian fault zone in connection to the AUSZs that controlled the coastline all along the western and eastern Cotentin. No younger vertical fault reactivation (rupture) has occurred since the relaxation of the Late Miocene doming in either offshore or onshore Armorica. This wide N150 faulted zone was regularly seismically active during the Holocene (RèNaSS).
3.4 Tectonic and paleoseismic regional indices
3.4.1 Present tectonic setting
The stress pattern determined by Delouis et al. (1993) and Amorèse et al. (2000) for the Armorican Massif is currently extensive, close to a strike–slip stress pattern, with a σ3-oriented N35. The strike–slip dynamic accommodates this stress around the rigid Cadomian Armorica basement across western Normandy and the Cotentin and through movements in the central Armorican Hercynian Cadomian domain. These rigid bodies have been almost stable since 3.2 Ma despite the uplift. Around this domain, shearing zones sinistrally accommodate the southern plate convergence inland, following the southern Hercynian Front (SVF) and the Yeu–Retz Fault Zone (Mazabraud et al., 2005; Beucler et al., 2021). To the north, the shearing has remained sinistral along the MCFZ, the AUFZ, and the northern Hercynian Front (NVF) Fault Zone, contouring the rigid bodies (Figures 1, 2).
At present, these rigid bodies are commonly characterized by very low seismicity (<3.5 Mw) (Réseau National de Surveillance Sismique [National Seismic Monitoring Network], RèNaSS), although the active shearing zones show relatively frequent seismicity above 3.5 Mw, with exceptional events of ≥5 Mw occurring offshore, or especially along the southern branch of the SASZ (Beucler et al., 2021). The conjugate N130–N150–N180 late Hercynian Fault System (e.g., the Quessoy–Nort-sur-Erdre and Kerforne faults in Figure 2) operates dextrally. Focal mechanisms have shown dominant transpression. There are two earthquake groups associated with the system, the first reaching depths close to 20–35 km in the lower laminated crust and the second being shallower, at ∼10–15 km depth, especially around the transition between the English Channel and Armorica. The bulk NW–SE anisotropy inherited from the Cadomian and Hercynian orogens has primarily guided this extensive trend, as well as the reactivation of the major late-stage faults responsible for the Bay of Biscay’s geometry. Following the orientation of these faults in relation to the proposed current transpressive stress regime, the main sliding mechanisms along the faults include
• an inverted mechanism on the N60–N70 faults
• an inverted mechanism on dextral transpression in the N80–N100 faults
• a dextral transpression mechanism on the N130–N140 faults, but possibly also with normal faulting
• vertical N150–N160 faults parallel to the axis of the maximum main stress that, thus, may not be active
• possible vertical N60–N70 faults perpendicular to the axis of the maximum N150–N160 horizontal stress, which might also be inactive in the Cotentin area today
3.4.2 Neogene and Pleistocene records
3.4.2.1 Offshore seismic lines
The history of the English Channel and the Western Approaches can be seen in the tectonic activity visible in offshore seismic lines up to the Upper Miocene. Above that, these seismic lines do not provide a precise view of the tectonic timing. It is difficult to determine whether the fracturing or superficial folding of a thin sedimentary cover correlates to horizontal or vertical transtension or transpression (Benabdellouahed, 2011). The most recent sedimentary units have widely been attributed to the Miocene to Plio-Quaternary. The undisturbed marine “Plio-Quaternary” sedimentary units in these seismic lines commonly record three to four sub-units (Benabdellouahed, 2011), sometimes even up to six (Auffret and Larsonneur, 1977). Because the Tortono-Messinian interval represents six eustatic cycles (Hardenbol et al., 1998), these are probably represented by the Cockburn Fm (Upper Miocene) and possibly the Pliocene Little Sole Fm (Zanclean to Gelasian) under a thin cover of middle to recent Quaternary (Le Roy et al., 2011). In the Western Approaches (Le Roy et al., 2011), the Garlizenn−1 core clearly reveals this succession, based on micropaleontological data (geological map Ouessant sheet 1/250,000), such as the well-dated record from offshore of Iberia (Somoza et al., 2021). This seems also valid for the Cenozoic early history of the Hurd Deep issued from seismic profiles (Bourillet et al., 2003).
Surface ruptures, sometimes containing giant co-seismic load casts in deep saprolite, have been observed by us onshore in association with N60 directions, such as in old quarries in small basins in central Brittany, and with N45 directions at Avranches (in the works of the E3 highway). Further evidence exists at the top of the Jurassic clays close to Saint Côme du Mont (Carentan Basin, E46 highway). All these features are difficult to date.
3.4.2.2 Upper Miocene
Most of the Upper Miocene coastal deposits in Brittany were transgressive prior to 7 Ma during the early Tortonian and early Zanclean, guided by the earlier transtensive N–S deformations, filling small basins at ∼+40 m NGF, such as at Missillac and Lauzauch, and in the prolongation of the SASZ at Le Rhys (+4 m NGF, Supplementary Figure S1) but emerged afterward. The transtension has been associated with clear co-seismic events at Réguigny, as large dewatering pipes at St Jouan-de-l’Isle (NASZ; Supplementary Figure S4) and in the St Vigor Basin (Normandy, Supplementary Figure S11) (see Supplementary Material). They were then faulted by N150–N170 transpression (flower structures, extrusion of iron-/manganese-stained blocks, or faulted paleopodzols and orsteins: Figure 4) (Van Vliet-Lanoë et al., 2002). This N150 faulting occurred during the merging Messinian doming mostly from 6.7 Ma (Supplementary Table S1). After the late Messinian doming, the shelly limestone (faluns) at Le Quiou (NASZ) (Néraudeau et al., 2011) were transtensively N–S faulted during the early Zanclean relaxation, as also deforming the Tortono–Messinian of the Valmont outcrop in the Seine Estuary (Figure 3) (Dugué et al., 2012).
3.4.2.3 Plio-Quaternary co-seismic activity
The NEOPAL database (2009) does not contain any evidence of paleoseismic ruptures or Quaternary tectonic deformation. Between the two Hercynian fronts, paleoseismic activities have been recorded in brief clusters (<2.4 and ∼1.4 Ma), as observed along the coast or in the upper layers of quarries (Figures 1B, 4). Load casts deforming flood plain deposits younger than 1.2 Ma, as at Millières or Reguigny basins (Supplementary Figure S3, S12), suggesting a period of global relaxation with recurrent earthquakes. This is consistent with observations along the Iberian Chain (eastern Spain, N170 fault) between 2.9 and 2.6 Ma (Liesa et al., 2016) or the Danube Upper Terrace (2.4–2.0 Ma) (Magyari et al., 2005; Ruszkiczay-Rüdiger et al., 2018; Ruszkiczay-Rüdiger et al., 2020), but in Hungary with at least twice the Armorican uplift rate and ongoing tectonic contributions.
In SE Normandy, the Senonche/Eure Fault (N110) moved dextrally, deforming the highest terrace conglomerate (Messinian) in Riedel’s folds (Moguedet et al., 2000; Van Vliet-Lanoë et al., 2002), in connection with an uplift in the Western Paris Basin around the Early/Middle Pleistocene boundary (from fluvial terrace dating) (Guillocheau et al., 2000; Antoine et al., 2007).
An ∼1,2–0.7 Ma S–N transient doming period seemed to develop in certain regions of the European plate, especially in Iberia (Gilbert et al., 2005), resulting from an increase in stress at its southern boundary. This doming is evidenced in Armorica by the lack of raised beaches of that age (Van Vliet-Lanoë and Guillou, 2021), despite a continuous record of fluvio–periglacial inland terraces in Picardy and high Normandy (Antoine et al., 2007).
A second cluster of paleoseismic activities took place at ∼400 ka at Pen Hat and Trez Rouz on the Crozon Peninsula (Supplementary Figure S7). Here, the paleoseismic activity was associated with shale diapirism (Figure 5; Supplementary Figure S3) and recorded on the Crozon Peninsula, in the Elorn Estuary, and at Pénestin, as the distal response to a potential MLv 6 deep earthquake in the SASZ or the nearby Kerforne Fault Zone (N150) (Van Vliet-Lanoë et al., 2009; 2019). At the regional scale, the 400 ka one possibly corresponds to a distal response to a major earthquake, ∼MSK 4.5–5 (magnitude analytical scale in Olson et al., 2005), probably along the SASZ or the nearby Elorn NASZ (Van Vliet-Lanoë et al., 2019). This 400-ka seismic and/or tectonic event has been recognized as a major boundary in Quaternary stratigraphy along the North Atlantic coasts, as previously mentioned by Mangerud et al. (1996). In Iceland, the event followed a period of dated high sub-glacial volcanic activity, suggesting some enhanced activity on the mid-Atlantic Ridge (Van Vliet-Lanoë and Guillou, 2021).
No younger vertical fault reactivation (rupture) has occurred since the relaxation of the Late Miocene doming in either offshore or onshore Armorica. This wide ∼N150 western Cotentin faulted zone was regularly seismically active during the Holocene (RèNaSS).
The normal to “pseudo-reverse” superficial faulting observed in the Middle Pleistocene in eastern France revealed it to be of thermokarstic origin (ice-melt and collapse) (Van Vliet-Lanoë et al., 2017). In the Mio–Pliocene Red Sands, faults with an offset that increases toward the surface correspond to a collapse feature with consolidation on karstified chalk or permafrost in drained sands (valley side). Various such faults were further reactivated as sand or ice wedges, as at Lessay and St Vigor (Cotentin Basin), in the Rennes Basin, at Pénestin (SASZ), or at La Londe and Valmont (Seine Estuary, Supplementary Figure S15) (Figure 2). Faulting younger than 700 ka (MIS 16) in tidal sands is mostly related to continued collapse activity.
The maximal Neogene extent of permafrost seems to have occurred around 6–5 Ma, according to the work of Gilichinsky et al. (2007, personal communication 2018). As regional periglacial activity set in from the Tortonian, it is possible that permafrost—at least in a discontinuous form—had already occurred prior to orstein formation in the Cotentin Basin. The polygenetic sand wedges described from the Lessay tidal sands recorded (Supplementary Figure S10), prior to the orstein (>6.7 Ma), should, thus, correspond to the second Tortonian glacial event. Various so-called faults were further reactivated during the Quaternary in the form of sand or ice, as in the Rennes Basin or at Pénestin (SASZ).
3.5 Armorican isostatic uplift
3.5.1 Doming, Atlantic rifting, and glaciations
Basement horsts, such as western Brittany, Jersey, and northern Cotentin (Renouf, 1993), have been in relief since at least the Alpine Orogeny and perhaps even since the Laramide Orogeny (Maastrichtian) (Figure 3). HS cobbles attributed to the Cenomanian in Central Brittany (Hallégouët, 1972) have been found close to 200 m, suggesting a total of 120 m in relative inland uplift as the early Turonian eustatic HS is considered to have reached ∼80 m (Van Sickel et al., 2004). This suggests an average Cenozoic uplift rate of 0.03 mm ky−1 for Central Brittany.
The post-Pyrenean doming of the continents began around 33 Ma along the North Atlantic (early Oligocene) (Ogg et al., 2016) after a change in the rifting geometry north of Iceland (Gaina et al., 2017; Martinez et al., 2020). An acceleration in rifting from 33 Ma led to a major SL drop at 28 Ma, synchronous with the isolation of Antarctica and the formation of the East Antarctic Ice Sheet. The rifting activated renewed uplift around the North Atlantic during the Early Miocene to 15 Ma, in the very late Tortonian (Late Miocene, 7–5.4 Ma), and during the Early Pleistocene (Mosar et al., 2002; Anell et al., 2009; Japsen et al., 2014; Figure 7), synchronous with the erosion of the Paleogene saprolites. The evolution of the morphology correlates with the mid-Atlantic spreading rates, which were moderate from ∼5 to 2 Ma, slowed down between ∼2 and 1 Ma, and then speeded up again from ∼1 Ma (Le Douaran et al., 1982).
Glaciations have also been recorded from 33 Ma (Table 1). These were responsible for an increase in glacial and periglacial erosion efficiency and the related unloading all over the North Atlantic region (Japsen et al., 2014). According to Pérez et al. (2018), several major phases of ice-sheet advance in the Northern Hemisphere (NH) took place in the Early Pliocene and around the Plio-Pleistocene transition (at 5.3 Ma and between 2.9 and 2.3 Ma, respectively), synchronous with the observed relaxation events. This retroactively led to an unloading-triggered uplift of the continents connected to the isostatic accommodation to erosion and crustal thinning (Mosar et al., 2002; Cloetingh et al., 2013; Japsen et al., 2014; Bridgland et al., 2020). Only early-glacial coolings were associated with abrasion in periglacial shoreface contexts (Van Vliet-Lanoë and Guillocheau, 1995; Van Vliet-Lanoë et al., 2009). During full glacials, the decrease in precipitation reduced their erosion capability (Bonnet and Crave, 2003).
3.5.2 Raised beaches and river terraces
Quaternary (marine or fluvial) staircase terraces have commonly been attributed to tectonic activity (Lenôtre et al., 1999; Kirby and Whipple, 2001; Coutard et al., 2006; Antoine et al., 2007). In Western Europe, the Quaternary interglacial raised beaches, recorded on the lower platform south of the Dover Strait, are commonly limited in age to the last ∼500 ka (MIS 15), as in Armorica (Van Vliet-Lanoë and Guillou, 2021). In Armorica, the absence of Quaternary interglacial raised beaches between 0.7 and 1.4 Ma suggests a lowered HS altitude than the current global SL average (±10 m or less) between late MIS 22 and 16—the oldest major Quaternary glaciations. This absence of traces, thus, corresponds to most of the Middle Pleistocene Event (MPE) at 1.25–0.7 Ma, a major shift in climate (Landais et al., 2010). However, along the Armorican coasts, there is no evidence of marked vertical movements for the last 500 ka despite the two recorded seismic clusters (Table 1; Figure 13) with between 0.7 and 0.4 Ma, hosting possibly a transient doming related to the main shear zones and N150 faults in the plate. A now-flooded HS is susceptible to confusion with other LSs.
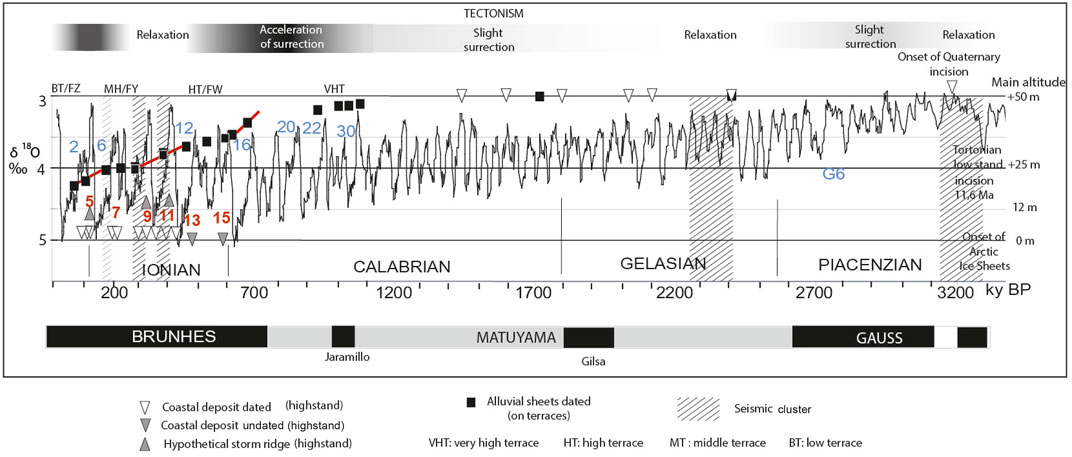
FIGURE 13. Periods of seismic activity and uplift identified in the sedimentary record and a comparison of the coastal record (raised beaches) with the inland record (river terraces) averaged to 50 m of uplift (Antoine et al., 2007; Defive et al., 2007; Voinchet et al., 2007) and the LR04 isotopic curve (Lisiecki and Raymo, 2005). Numbers in blue indicate major glaciations.
The apparent limited rise in the altitude of the HSs back to the MIS 11 HSs (∼+15 m NGF) was not controlled by climate, all the interglacials, except for MIS 7, having similar temperate climates and HSs close to those of the present (∼+4 m NGF). The intensity of regional coastal reshaping by HSs appears to have been limited during the Quaternary and is mostly expressed in terms of retrogressive coastal erosion and periglacial erosion during the “lowered” SL in the early interstadials. This was synchronous with sedimentary transfer to the shelf and responsible for limited unloading and uplift at the coast (Figure 8) during the last glacial period, on the order of 1 m or of 0.02 mm kyr−1.
Onshore, the stepwise incision of the river network records evident major inland uplift when using averaged terrace altitudes within a 30-m-amplitude range (averaged uplift value for the dated alluvial sheets: Antoine et al., 2007; Defive et al., 2007; Voinchet et al., 2007). Based on a homogenized scale for altitude, the ESR-dated fluvial terraces have revealed a nearly continuous uplift from 1.1 Ma (Figure 13). Significantly, the incised steps (the classical upper, middle, and lower terraces) were marked by periods of accelerated uplift (red bars in Figure 8), resulting from transient and limited doming. Inland, in Picardy, the average Quaternary rate of uplift reached 0.06 mm yr−1 on the frost-susceptible chalk of the Somme terraces (Antoine et al., 2007). Data from the Ebro River terraces in Spain (Regard et al., 2021) fit these observations, with a major river incision tracing an uplift of tectonic origin occurring between 0.65 and 0.4 Ma in southern Spain (Gilbert et al., 2005). Traces of surface rupture were recorded on the French side of the Pyrenean Belt (Baize et al., 2002).
Older Neogene sediments may record similar events. The Messinian lithospheric doming event, associated with the Jura Orogeny (Becker, 2000), developed from ∼8–4 Ma. The Jura Plateau has been deeply incised by rivers since at least the Pliocene. From the onset of Messinian doming, periglacial erosion became dominant in the still-moist early-glacial stages. This seems to have been associated with enhanced unloading, followed by a seismic cluster. The river incision to the Middle Terrace in Brittany was related to a transient acceleration in uplift (Figures 12, 13; Table 1), in tandem with the observed Messinian doming from approximately 8 Ma to 4.5 Ma. Periglacial erosion has also probably been having an impact for the last 5.4 Myr and possibly also during the 11.6, 9.0, and 6.7 Ma glacial events. The Middle Pleistocene event set on from 3.2 Ma for a maximal efficiency from 1.1 to 0.7 Ma.
Neogene paleoseismic event clusters have been recorded at the onset of tectonic strain relaxation (deglaciation: Hasegawa and Basham, 1989), supplemented by unloading events ruled by major periglacial erosion or sea-ice-erosion-related events (Van Vliet-Lanoë et al., 2002). This has generally occurred close to the onset of regressions with a limited fall in SL and major sediment transfer (Van Vliet-Lanoë and Guillocheau, 1995). This long-term interpretation of uplift agrees with the hypothesis for an Early Miocene onset of the coastal marine terrace sequence preserved on the Cotentin Peninsula (Pedoja et al., 2018). A similar situation likely also occurred during pre-Langhian doming (23–14 Ma), related to shorter coolings and higher precipitation on the weakly incised Oligo–Miocene River system (Van Vliet-Lanoë et al., 2002).
4 Discussion
The passive continental margin of Armorica has experienced a pulsed N170 compressive stress field since at least the Neogene, in combination with a W–E transtensive regime. The application of compressive stress at the edge of a craton can lead to uplift supplemented by the development of extensive erosion surfaces (Japsen et al., 2014). Here, this was reflected in pulsed and distal episodes of compression-induced crustal doming in Armorica, monitored by the plate’s collision history. In the Appalachian Mountains—a situation similar to that in Armorica—evidence points to an ongoing wave of 100–150 m of river incision that has been propagating upstream for at least 3.5 Myr and possibly since the 11 Ma LS. This drives the isostatic relief uplift across this part of the passive margin (Miller et al., 2013).
4.1 Quaternary crustal unloading: differential uplift between the marine platform, the coast, and the continent
We indicated that the first sedimentary evidence of the present river network goes back to ∼3.05 Ma in Brittany (Van Vliet-Lanoë et al., 2002) after the onset of extended Quaternary glaciations at 3.2 Ma. The Early Quaternary SLs (>1.25 Ma) also indicate an apparent regional stabilization of the relative HS at ∼50 ± 10 m between 3.2 and 1.4 Ma (Supplementary Table S1). The recorded SL was lowered by only a few meters during this period despite some major glaciations defined by the LR04 δ18O curve (Lisiecki and Raymo, 2005) (Figure 8).
The absence of raised beaches between 1,000 and 700 ka contrasts with the river terraces, several of which are dated as between ∼1,250 and 700 ka (see references in Figure 13). This suggests a coastal uplift or doming of undated raised beaches above 20–30 m a.s.l., making them difficult to discriminate from the Neogene ones, except where an orstein is present. Ablation unloading after 700 ka on the PS7–3 platform was potentially responsible for an uplift of ∼14 m (0.02 m kyr−1) during successive interglacial–glacial transitions. This uplift does not agree with the known regional thermal subsidence of 5.2 mm kyr−1, according to Rouby et al. (2013). This suggests a regional deficit of ∼5–10 m in coastal margin subsidence, probably due to a passive or tectonic uplift.
A transient lithospheric doming and a major differential uplift suggest that the absence of an Early Quaternary record is related to a nearly stable coastal platform as opposed to the inland uplift rate (0.05–0.06 mm kyr−1) (Mazzotti et al., 2020). The main erosional efficiency of the Weichselian Glacial occurred inland during the Early Glacial (∼113–75 ka) (Van Vliet-Lanoë and Guillocheau, 1995) relative to a more discontinuous vegetation cover on the continent. The coastal 0.02 mm kyr−1 value likely explains the limited and progressive raising of the paleo beaches above the present mean SL from MIS 5 to MIS 11 (to ∼+15 m), regionally completed by the interglacial glacio-isostatic accommodation of 0.8 kyr−1 (Peltier, 1999) that was only active during post-deglaciation interglacial periods.
4.2 Seismicity
4.2.1 Generality
Amplification of the regional inversion structures that grew from the Late Jurassic to the early Oligocene was related to a temporary decrease in the spreading rates in the mid-Atlantic and oceanic-crust sagging (Figure 7) (Müller et al., 2008; Gaina et al., 2017). Reduced mid-Atlantic spreading rates led, especially from 34 Ma, to a W–E extension induced by mantle drag (Mosar et al., 2002), a subsequent inversion of Western European Mesozoic basins, and platform unloading (with an SL drop of ∼50 m) (Figure 3). An acceleration of the mid-Atlantic spreading rate confirmed the field dating—∼15, ∼8–5, briefly ∼2.4–2, and limited ∼1–0.7 Ma (Figure 3)—resulting in S–N transpression. Since 14 Ma, transient doming events complemented climate-induced erosion (Stoker et al., 2005).
4.2.2 Deep-seated seismicity: the impact of lithospheric doming events and climate
From our Neogene record, seismic activity clearly increased regionally at the onset of transtensive relaxation following doming events. From 18 Ma, the progressive closing of the Gibraltar Strait (Krijgsman et al., 2018; Civiero et al., 2020), related to the late Alpine Orogeny, induced a rise in S–W to SE–NW strain, coupled with an overall lowering of the mid-Atlantic spreading rate, and a renewed glacio-eustatic SL fall, especially from 5.3 Ma (Figure 3). Transpressive deformations induced by lithospheric doming events were associated with surface rupture up to the Messinian–Zanclean transition (5.3 Ma), such as around the North Atlantic (Stoker et al., 2005; Anell et al., 2009). It is not possible to be more precise. No younger surface rupturing or faulting was observed regionally.
From the paleoseismic regional evidence and ESR dating, we can, thus, assume that the major Quaternary earthquake clusters in Armorica were associated with the resumption of transient, but limited, lithospheric doming events related to the ∼N170 oriented strain, transmitted via the main shearing zones from the southern plate convergence (Figure 1A). This should explain the slightly accelerated uplift during the paleoseismic clusters (Figure 13, red bars).
The Armorican and Channel Shear Zones, with their conjugate faults, maintained control over the deep-seated seismicity during the Pliocene and Pleistocene, similar to the present. This is especially the case for the SASZ complex, under the supplementary tensional influence of the nearby Gulf of Biscay (Figures 1, 2) or of the AUFZ. Presently, N150 crustal faults activate along the main shear zones, allowing deep-seated (20–30 km, laminated crust) regional earthquakes to occur (BCFS/RéNaSS, 2022). The highest regional magnitude has been recorded by the French Seismological Central Office (BCFS/RéNaSS, 2022) at a depth close to 15 km along the northern branch of the SASZ for the Lorient earthquake (30 September 2002, 5.4 MLv, Mw 4.3) (Perrot et al., 2005) and the Oléron Island earthquake (30 August 2002, 5.7 MLv) The most recent, in Vendée on 16 June 2023, yielded an Mw 4.8 at a depth of only 5 km (RéNaSS).
More limited, ubiquitous, and shallow (15–5 km) regional seismicity or natural seismic noise could accommodate all the existing, sometimes hindered, Tardi–Hercynian faults, reactivated by the Late Jurassic to mid-Cenozoic inverted horsts, as stressed more globally by Sibson (1990) and Scholz (1998).
A climate-induced increase in rhexistasy during cooling episodes (Van Vliet-Lanoë and Guillocheau, 1995) was, thus, complemented by the isostatic accommodation to an erosion-induced unloading mechanism (i.e., erosion isostatic accommodation). With the progressive differential isostatic uplift related to erosion, accommodation earthquakes could potentially occur with limited ≤4-MLv strength (BCFS/RéNaSS, 2022) and produce no visible surface rupture.
4.2.3 Shallow seismicity: the impact of climate-induced isostatic unloading on merging old basement
During the onset of glaciations (MIS 12 and MIS 8), the limited reduction in precipitation, but with recurrent drought, led to the increase in sedimentary transfer to the platform (progradation). This onshore unloading with the onset of SL regression could have superficially increased the stress on existing faults and shear zones along the coasts, such as during the ∼275 ka seismic clusters along the SASZ. This could have induced some shallow events complementary to the classical deep-seated earthquakes, such as for the ∼400 and ∼275 ka paleoseismic event clusters (∼MSK 4.5–5 in the Olson et al. (2005) scale; Van Vliet-Lanoë et al., 2019).
4.2.4 Erosion-induced seismic activity
Because the region is mostly W–E extensional, after the late Early Cretaceous inversion and mid-Atlantic widening, a rise in the old crust of the brittle–ductile transition (BDT), from 30 km below the surface to <15 km (cooling from ∼350°C) (Mehl et al., 2005), may have complemented the erosion isostatic accommodation uplift by exposing the Cadomian or Hercynian BDT. This could potentially have contributed to the exhumation of the metamorphic and granitic bodies (Mehl et al., 2005; Petrillo et al., 2020) inherited from the Cadomian subduction front, as recorded North of the Bay of St Michel-en-Grève (Supplementary Figure S17). This process is normally controlled by the thermal gradient and fluid pressure in the crust, which aids in reactivating older, strain-opened faults and other fractures (Sibson, 1990). The limit for shallow earthquakes could result from this rising of the former BDT in the crust, lifted by isostatic accommodation to erosion. Recent low-magnitude earthquakes (28 February 2022, 1 June 2022, and 27 June 2021) along the inactive N150 fault line off western Cotentin, with a focal depth close to ≤10 km deep, could argue for this scenario for shallow instrumentally recorded earthquakes (Sisfrance BRGM-EDF-IRSN/SisFranc, 2010) yielding ∼2–4 MLv. From the Middle Holocene, some accentuation of the isostatic uplift may have resulted from a generalized anthropogenic transformation and powerful erosion of the landscape, of ∼0.2 mm kyr−1 in the last 2 kyr (according to the work of Van Vliet-Lanoë et al. (1992)) instead of an average value of ∼0.05 mm kyr−1 inland. The ubiquitous seismic noise (≤MLv 3.5) recorded regionally might be due to the local reactivation of pre-existing faults that are not necessarily parallel to the stress field (Sibson, 1990) in an unloaded BDT zone.
5 Conclusion
The post-Eocene marine platforms (PS–7) around Armorica are relatively continuous and have been shaped since at least 34 Ma, but have a post-Eocene marked thermal subsidence in the Western Approaches of the English Channel with a thick sedimentation load along the southern Armorican shelf edge. The PS7–3 platform developed during the late Neogene LSs (particularly since 11.6 Ma) was only re-occupied after the 700 ka HSs, often in continuity with the Paleogene PS6 and younger platforms. Several transient, strain-induced, lithospheric doming events, distal from the southern plate boundary, enhanced the marine regressions, particularly from 33 Ma to, notably, 1.2 to 0.7 Ma. From the Pleistocene (∼2.5 Ma), the stability of the HS altitude has been recorded at the coast by limited isostatic uplift, together with major continental unloading and uplift in response to enhanced periglacial erosion during regressions as perhaps also from the Oligocene, the Miocene, and especially, the Messinian LSs. The Middle Pleistocene glacial amplification and the glacial events with the longest early-glacial stages were, with erosion unloading, the major contributors to the Quaternary uplift. This would explain the non-tectonic differential uplift between the continental relief and the coastal zones, including nearshore platforms.
In terms of the seismicity, a strike–slip deformation accommodated the Paleogene, Neogene, and Pleistocene SW–NE strains around the rigid part of the NW European Plate constituted by Normandy, the Cotentin, and the central Armorican Basin, zones of very low actual seismicity. The shearing zones have remained ductile and have accommodated the 14–16 Ma, 8–5.4 Ma, and probably, quaternary domings. The southern plate’s convergence during the Neogene was imprinted in the lower laminated crust (∼30 km), with a limited deformation to the west of Western Europe and deep-seated earthquakes. This is mostly constraining the SVF (Figure 2), the Yeu–Retz Fault Zone, and the complex SASZ. To the north of Armorica, the shearing remains counterclockwise along the Middle Channel Fault Zone, the AUFZ, and the NVF.
The traces of seismic activity in Armorica did not show any surface rupture since the Pliocene (4.4 Ma). Seismic records revealed a prevailing transtensive regime after the Gelasian (2.4 Ma) and from 0.7 Ma (the MPE) in association with an acceleration in continental uplift. From the beginning of the Late Pliocene, and mostly from the MPE onward, the intensification in continental erosion and temporary episodic N170 compressive strain on the ∼N150 faults were responsible for the 400 ka and 280–275 ka seismic event clusters.
Present-day seismicity along the shear zones has mostly been in the upper continental crust (≤20 km). Low-magnitude and ubiquitous shallow seismic activity is probably occurring close to the now-fragile and uplifted Hercynian BDT, as well as in zones of shear–strain accumulation (shear zones). A major Holocene isostatic uplift related to the man-made intensification of erosion could, for the most part, be responsible for the present-day ubiquitous and shallow seismicity.
Author contributions
BVV-L: conceptualization, data curation, formal analysis, investigation, methodology, resources, supervision, validation, and writing–original draft. CA: data curation, formal analysis, investigation, validation, and writing–review and editing. PL: data curation, formal analysis, validation, and writing–review and editing. JR: data curation, investigation, resources, validation, and writing–original draft. PC: data curation, formal analysis, funding acquisition, investigation, project administration, resources, validation, and writing–original draft. FE: conceptualization, funding acquisition, methodology, supervision, validation, and writing–review and editing.
Funding
The authors declare that no financial support was received for the research, authorship, and/or publication of this article.
Acknowledgments
The authors thank M. Benabdellouahed for reanalyzing the seismic sections, along with PC, from the Bay of Seine and around Cotentin.
Conflict of interest
The authors declare that the research was conducted in the absence of any commercial or financial relationships that could be construed as a potential conflict of interest.
Publisher’s note
All claims expressed in this article are solely those of the authors and do not necessarily represent those of their affiliated organizations, or those of the publisher, the editors, and the reviewers. Any product that may be evaluated in this article, or claim that may be made by its manufacturer, is not guaranteed or endorsed by the publisher.
Supplementary material
The Supplementary Material for this article can be found online at: https://www.frontiersin.org/articles/10.3389/feart.2023.1269598/full#supplementary-material
Abbreviations
MCSZ, Middle Channel Shear Zone; AUSZ, Alderney–Ushant Shear Zone; NASZ, North Armorican Shear Zone; SASZ, South Armorican Shear Zone; EF, Eure Fault, Se, Seine Fault; Vit, Vittel Fault; SO, Somme Fault; (S)VF, (southern) Hercynian Front; WA, Western Approaches; and SA, South Armorican Platform; HS, Highstand; LS, lowstand; BDT, brittle–ductile transition.
References
AlvInerie, J., Antunes, M. T., Cahuzac, B., Lauriat-Rage, A., Montenat, C., and Pujol, C. (1992). Synthetic data on the paleogeographic history of northeastern atlantic and betic-rifian basin, during the neogene (from Brittany, France, to Morocco). Palaeogeol., Palaeoclim. Palaeoecol. 95, 263–286. doi:10.1016/0031-0182(92)90145-u
Amorèse, D., Walker, A., Lagarde, J. L., Santoire, J. P., Volant, P., Font, M., et al. (2000). New seismotectonic data from an intraplate region: focal mechanisms in the Armorican Massif (northwestern France). Geophys. J. Inter 143, 837–846. doi:10.1046/j.0956-540x.2000.01285.x
Andreieff, P., Bouysse, P., Horn, R., and Monciardini, C. (1972). Contribution à l’étude géologique des approches occidentales de la Manche. Mémoire Du. BRGM 79, 31–48. doi:10.2113/gssgfbull.182.5.451
Andreieff, P., and Lefort, J.-P. (1972). Contribution à l’étude stratigraphique des terrains secondaires et tertiaires affleurant en Manche occidentaleMém. BRGM, Colloque sur la géologie de la Manche, 79, 49–56.
Anell, I., Thybo, H., and Artemieva, I. M. (2009). Cenozoic uplift and subsidence in the North Atlantic region: geological evidence revisited. Tectonophysics 474 (1–2), 78–105. doi:10.1016/j.tecto.2009.04.006
Antoine, P., Lozouet, N. L., Chaussé, C., Lautridou, J. P., Pastre, J. F., Auguste, P., et al. (2007). Pleistocene fluvial terraces from northern France (Seine, Yonne, Somme): synthesis, and new results from Interglacial deposits. Quat. Sci. Rev. 26, 2701–2723. doi:10.1016/j.quascirev.2006.01.036
Auffret, J. P., and Larsonneur, C. (1977). Paléovallées et bancs sableux entre l’estuaire de la SeIne et le Nord- Cotentin. Bull. Soc. Géol. Normandie 6 (2), 21–33.
Bahain, J.-J., Falguères, C., Voinchet, P., Duval, M., Dolo, J.-M., Despriée, J., et al. (2007). Electron spin resonance (ESR) dating of some European late lower Pleistocene sites. Quaternaire 18 (2), 175–186. doi:10.4000/quaternaire.1048
Baize, S. (1998). Tectonique, eustatisme et climat dans un système géomorphologique côtier. Le Nord-Ouest de la France au Plio-Pléistocène: exemple du Cotentin (Normandie). [PhD thesis] [France], Univ. Caen, Doc. BRGM 289, 366.
Baize, S., Cushing, E. M., Lemeille, F., Granier, T., Grellet, B., Combes, P., et al. (2002). Inventaire des Indices de rupture affectant le Quaternaire en relation avec les grandes structures connues en France métropolitaine et dans les régions limitrophes. Mém. Soc. Géol. Fr. 175, 1–142.
Ballèvre, M., Bosse, V., Dabard, M. P., Ducassou, C., Fourcade, S., Paquette, J. L., et al. (2013). Histoire Géologique du Massif armoricain: actualité de la recherche. Bull. Soc. Géol. Minér. Bretagne (D) 10-11, 5–96. insu-00873116
Baptiste, J. (2016). Cartographie structurale et lithologique du substratum du BassIn parisien et sa place dans la chaîne varisque de l’Europe de l’Ouest: approches combInées géophysiques, pétrophysiques, géochronologiques et modélisations 2D. [PhD thesis] [France]. Univ. Orléans.
Barrat, J. A., Taylor, R. N., Andre, J. P., Nesbitt, R. W., and Lecuyer, C. (2000). Strontium isotopes in biogenic phosphates from a neogene marine formation: implications for palaeoseawater studies. Chem. Geol. 168 (3–4), 325–332. doi:10.1016/S0009-2541(00)00200-X
Battistini, R., and MartIn, S. (1956). La « Plate-forme à écueils » du Nord-Ouest de la Bretagne. Norois 10, 147–161. doi:10.3406/noroi.1956.1121
Bauer, H., Bessin, P., Saint-Marc, P., Châteauneuf, J. J., Bourdillon, C., Wyns, R., et al. (2016). The cenozoic history of the armorican Massif: new insights from the deep CDB1 borehole (Rennes Basin, France), C R Geoscience 348, 387–397. doi:10.1016/jcrte201602002
BCFS/RéNaSS. (2022). National seismic MonitorIng network/réseau national de Surveillance sismique (RéNaSS). Paris. https://renass.unistra.fr (Accessed 10/September/2022).
Béchennec, F., Hallégouët, B., Thiéblemont, D., Thinon, I., Cocherie, A., Guerrot, C., et al. (2012). Notice explicative, feuille Lorient, 383, Carte géol. Fr. (1/50 000). BRGM Orléans, 206.
Becker, A. (2000). The Jura Mountains — an active foreland fold-and-thrust belt? Tectonophysics 321 (4), 381–406. doi:10.1016/S0040-1951(00)00089-5
Benabdellouahed, M. (2011). La Seine fluviatile plio-quaternaire en baie de Seine: évolution morphologique et sédimentaire (rôle du subtratum géologique et des cycles climato-eustatiques). [ PhD thesis] [France]. Univ. Caen, 300. Available at: https://theses.hal.science/tel-00660489.
Bergerat, F. (1987). Stress fields in the European Platform at the time of Africa-Eurasia collision. Tectonics 6, 99–132. doi:10.1029/TC006i002p00099
Bertran, P., Font, M., Giret, A., Manchuel, K., and Sicilia, D. (2019). Experimental soft-sediment deformation caused by fluidization and Intrusive ice melt in sand. Sedimentology 66, 1102–1117. doi:10.1111/sed.12537
Bessin, P., Guillocheau, F., Robin, C., Schroëtter, J.-M., and Bauer, H. (2015). Planation surfaces of the Armorican Massif (western France):Denudation chronology of a Mesozoic land surface twice exhumed in response to relative crustal movements between Iberia and Eurasia. Geomorphology 233, 75–91. doi:10.1016/j.geomorph.2014.09.026
Besson, D., Parize, O., Rubino, J. L., Aguilar, J. P., Aubry, M. P., Beaudoin, B., et al. (2005). Un réseau fluviatile d’âge Burdigalien terminal dans le Sud-Est de la France: remplissage, extension, âge, implications. C.R. Geosci. Paris 337, 1045–1054. doi:10.1016/j.crte.2005.05.009
Beucler, E., Bonnin, M., Hourcade, C., Van Vliet-Lanoë, B., Perrin, C., Provost, L., et al. (2021). Characteristics and possible origIns of the seismicity in northwestern France. C.R. Geosci. Paris 353, 53–77. doi:10.5802/crgeos86
Biagi, R., Andre, J. P., Moguedet, G., and Vervialle, J. P. (1996). Organisation de dépôts bioclastiques proximaux associes à une variation rapide du niveau marin relatif au Miocène supérieur, Ouest de la France. Bull. Soc. Géol. Fr. 169, 1145–1160.
Bois, C., Cazes, M., Choukroune, P., Gariel, O., Hirn, A., Le Gall, B., et al. (1994). “Seismic reflection images of the pre-mesozoic crust in France and adjacent areas,” in Pre-mesozoic geology in France and related areas IGCP-project 233. Editor J. Chantraine, (Berlin: Springer). doi:10.1007/978-3-642-84915-2_1
Bonnet, S., and Crave, A. (2003). Landscape response to climate change: insights from experimental modelIng and implications for tectonic versus climatic uplift of topography. Geology 31, 123–126. doi:10.1130/0091-7613(2003)031<0123:lrtcci>2.0.co;2
Bonnet, S., Guillocheau, F., Brun, J.-P., and Van den Driessche, J. (2000). Large-scale relief development related to Quaternary tectonic uplift of a Proterozoic–Paleozoic basement: the Armorican Massif, NW France. J. Geophys. Res. 105, 19273–19288. doi:10.1029/2000jb900142
Bourillet, J. F., Reynaud, J. Y., Baltzer, A., and Zaragosi, S. (2003). The ‘Fleuve Manche’: the submarine sedimentary features from the outer shelf to the deep-sea fans. J. Quat. Sci. 18, 261–282. doi:10.1002/jqs.757
Bridgland, D. R., Westaway, R., and Hu, Z. (2020). Basin inversion: a worldwide Late Cenozoic phenomenon. Glob. Planet. Change 193, 103260. doi:10.1016/j.gloplacha.2020.103260
Calvès, G., Torvela, T., Huuse, M., and Dinkleman, M. G. (2012). New evidence for the origin of the porcupine median volcanic ridge: early cretaceous volcanism in the Porcupine Basin, atlantic margin of Ireland. Geochem. Geophys. Geosyst. 13, Q06001. doi:10.1029/2011GC003852
Chadwick, R. C., Livermore, R. A., and Penn, I. E. (1989). Continental extension in southern Britain and surrounding areas and its relationship to the opening of the North Atlantic Ocean. Am. Ass. Petrol. Geol. Mem. 46, 411–424.
Civiero, C., Custódio, S., Duarte, J. C., Mendes, V. B., and Faccenna, C. l. (2020). Dynamics of the Gibraltar Arc System: a Complex Interaction between plate convergence, slab pull, and mantle flow. J.Geophys. Res. Solid Earth 125, 1–23. doi:10.1029/2019JB018873
Clark, P. U., Archer, D., Pollard, D., Blum, J. D., Rial, J. A., Brovkin, V., et al. (2006). The middle Pleistocene transition: characteristics, mechanisms, and implications for long-term changes in atmospheric pCO2. Quat. Sci. Rev. 25, 3150–3184. doi:10.1016/j.quascirev.2006.07.008
Clet-Pellerin, M. (1983). Le Plio-Pléistocène en Normandie. Apports de la palynologie. [PhD thesis], [France]. Caen: Univ. Caen, 135.
Clet-Pellerin, M., Baize, S., Walter, A. V., Dugué, O., and Coutard, J. P. (1997). Mise en évidence d’un Interglaciaire du Pléistocène Inférieur dans une formation fluviatile du Seuil du Cotentin (Normandie, France). Géogr. Phys. Quat. 51, 363–378. doi:10.7202/033136ar
Cliquet, D., Lautridou, J. P., Lamothe, M., Mercier, N., Schwenninger, J. L., Alix, P., et al. (2009). Nouvelles données sur le site majeur d’Écalgrain: datations radiométriques et occupations humaines de la Pointe de la Hague (Cotentin, Normandie). Quaternaire 20 (3), 345–359. doi:10.4000/quaternaire5244
Cloetingh, S., Beekman, F., Ziegler, P., Van Wees, A., and Sokoutis, D. (2008). Post-rift compressional reactivation potential of passive margins and extensional basins. Geol. Soc. Lond. Spec. Pub. 306, 27–70. doi:10.1144/SP306.2
Cloetingh, S., Burov, E., Maţenco, L., Beekman, F., Roure, F., and Ziegler, P. A. (2013). The Moho in extensional tectonic settings: insights from thermo-mechanical models. Tectonophysics 609, 558–604. doi:10.1016/j.tecto.2013.06.010
Cloetingh, S., and Haq, B. U. (2015). Inherited landscapes and sea level change. Science 347, 6220. doi:10.1126/science.1258375
Courville, P., and Bongrain, M. (2003). Les PectInidae miocènes des faluns (Ouest de la France) Intérêts biostratigraphiques des associations. Ann. Paléonto. 89, 125–151. doi:10.1016/S0753-3969(03)00022-3
Coutard, J. P., Van Vliet-Lanoë, B., and Clet, M. (1991). Les fentes de gel et coins de glace de Lessay (Manche). Bull. Soc. Linn. Normandie. 114-115, 69–78.
Coutard, S., Lautridou, J. P., Rhodes, E., and Clet, M. (2006). Tectonic, eustatic and climatic significance of raised beaches of Val de Saire, Cotentin, Normandy, France. Quat. Sci. Rev. 2 (5–6), 595–611. doi:10.1016/j.quascirev.2005.02.003
Darboux, J. R., Marcoux, E., Hallégouët, B., et al. (2010). “Notice explicative, carte géol France (1/50 000), feuille 239 Landerneau, BRGM orléans,” in With the 1995 contributions. Editors B. Hallégouët, J. P. Margerel, P. Carbonel, A. Lauriat-Rage, Ph. Brébion, and R. P. Carriol, 146.
Defive, E., Pastre, J. F., Lageat, Y., Cantagrel, J. M., and Méloux, J. L. (2007). “L’évolution géomorphologique néogène de la haute vallée de la Loire, comparée à celle de l’Allier » in Du continent au bassin versant,” in Théories et pratiques en géographie physique (Clermont-Ferrand, France: Univ Blaise-Pascal), 469–484. 978-2-84516-335-5.
Delanoë, Y. (1988). Les grands traits de la structure et de l’évolution géodynamique des dépôts tertiaires du plateau continental sud-armoricain d’après les enregistrements de réflexion sismique. Géol. Fr. 1, 79–90.
Delanoë, Y., Margerel, J. P., and Pinot, J. P. (1976). En Baie de Concarneau, l’Oligocène marin est discordant sur un Eocène ondulé, faillé et érodé, et l’Aquitanien a voilé l’ensemble après une nouvelle pénéplanation. C.R. Acad. Sci. Paris 282 s.d, 29–32.
Delaygue, G., Jouzel, J., Minster, J. F., Dufresne, J. L., Boucher, O., and Méllière, M. A. (2001). La fonte des glaces et l’élévation du niveau marin. Planet Terre - ISSN 2552-9250 https://planet-terre.ens-lyon.fr/ressource/fonte-des-glaces.xml.
Delouis, B., Haessler, H., Cisternas, A., and Rivera, L. (1993). Stress tensor determination in France and neighbouring regions. Tectonophysics 221, 413–438. doi:10.1016/0040-1951(93)90171-f
Dugué, O., Bourdillon, C., Quesnel, F., and Lautridou, J. P. (2012). The neogene and lower Pleistocene crags of upper Normandy: biostratigraphic revision and paleogeographic implications. CR Géoscience 344, 415–422. doi:10.1016/j.crte.2012.07.001
Dugué, O., Lautridou, J. P., Quesnel, F., Poupinet, N., Clet, M., Camuzard, J. P., et al. (2009). Évolution sédimentaire cénozoïque (Paléocène à Pléistocène inférieur) de la Normandie. Quaternaire 20 (3), 275–303. doi:10.4000/quaternaire.5211
Dugué, O., Poupinet, N., Baize, S., Auffret, J.-P., Coutard, J.-P., Ozouf, J.-C., et al. (2000). Stratigraphie du Plio-Pléistocène Inférieur de Normandie: les séries marines et fluviatiles des bassins du Seuil du Cotentin. Géol. Fr. 3, 99–125.
Durand, S. (1949). Yprésien fossilifère et Lutétien aux environs de Port-Louis (Morbihan) C. R. Acad. Sci. Paris 229, 230–232.
Eldrett, J. S., Harding, I. C., Wilson, P. A., Butler, E., and Roberts, A. P. (2007). Continental ice in Greenland during the Eocene and Oligocene. Nature 446, 176–179. doi:10.1038/nature05591
Evans, C. D. R., Hillis, R. R., Gatliff, R. W., Day, G. A., and Edwards, J. W. F. (1990). British geol. Surv., U K offshore. Rep. Pub. HMSO. https://pubs.bgs.ac.uk/publications.html?pubID=B01850. The geology of the western English Channel and its western approaches
Evans, C. D. R., and Hughes, M. J. (1984). The Neogene succession of the southwestern approaches, great Britain, 141. London: J. Geol. Soc. London, 315–326.
Faure, M. (1995). Late orogenic carboniferous extensions in the variscan French Massif central. Am. Geophys. Un. 14, 132–153. doi:10.1029/94TC02021
Font, M., Lagarde, J. L., Amorèse, D., Coutard, J. P., and Ozouf, J. C. (2002). Une méthode de quantification de la dégradation d’un escarpement de faille au cours des cycles climatiques du Quaternaire: la faille de Jobourg (Cotentin, France). C.R. Geosci. Paris 334, 171–178. doi:10.1016/s1631-0713(02)01732-7
Furgerot, L., Poprawsk, Y., Violet, M., Poizot, E., Bailly du Bois, P., Morillon, M., et al. (2019). High-resolution bathymetry of the Alderney Race and its geological and sedimentological description (Raz Blanchard, northwest France). J. Maps 15 (2), 708–718. doi:10.1080/17445647.2019.1657510
Gaina, C., Nasuti, A., Kimbell, G. S., and Blischke, A. (2017). Break-up and seafloor spreading domains in the NE atlantic. Geol. Soc. Lond. Spec.Pub. 447 (1), 393–417. doi:10.1144/sp447.12
Gardère, P., and Pai, J. (2007). Palynologic data from aquitaine (SW France): middle Miocene sables fauves formation climatic evolution. Ciências Terra (UNL) Lisb. 15, 151–161.
Gilbert, L., Sanz de Galdeano, C., Alfaro, P., Scott, G., and Lopez Garrido, A. C. (2005). Seismic-induced slump in early Pleistocene deltaic deposits of the Baza basin (SE Spain). Sedim. Geol. 179, 279–294. doi:10.1016/j.sedgeo.2005.06.003
Gilichinsky, D. A., Wilson, G. S., Friedmann, E. I., McKay, C. P., Sletten, R. S., Rivkina, E. M., et al. (2007). Microbial populations in Antarctic permafrost: biodiversity, state, age, and implication for Astrobiology. Astrobiology 7 (2), 275–311. doi:10.1089/ast.2006.0012
Greiner, B., and Neugebauer, J. (2013). The rotations opening the Central and Northern Atlantic Ocean: compilation, drift lines, and flow lines. Int. J. Earth Sci. 102, 1357–1376. doi:10.1007/s00531-012-0860-6
Guillocheau, F., Brault, N., and Thomas, E. (2003). Histoire géologique du Massif armoricain depuis 140 Ma (Crétacé-Actuel). Bull. Info. Géol. Bassin Paris 40, 13–28.
Guillocheau, F., Robin, C., Allemand, P., Bourquin, S., Brault, N., Dromart, G., et al. (2000). Meso-Cenozoic geodynamic evolution of the Paris Basin: 3D stratigraphic constraints. Geodin. Acta 13, 189–245. doi:10.1016/S0985-3111(00)00118-2
Gupta, S., Collier, J. S., Palmer-Felgate, A., and Potter, G. (2007). Catastrophic flooding origin of shelf valley systems in the English Channel. Nature 448, 342–345. doi:10.1038/nature06018
Hallam, A. (1971). Mesozoic geology and the opening of the North atlantic. J. Geol. 79, 129–157. doi:10.1086/627605
Hallégouët, B. (1972). Découverte d’un gisement de galets marins vers 200 mètres d’altitude, près de Scaer (Finistère). C.R.Acad.Sci. Paris 275, 1859–1861.
Hallégouët, B., and Van Vliet-Lanoë, B. (1989). Héritages glaciels sur les côtes du Massif armoricaIn, France. Géogr. Phys. Quat. 43, 223–232. doi:10.7202/032771ar
Hardenbol, J., Thierry, J., Farley, M. B., Jaquin, T., Graciansky, P. C. D., and Vail, P. (1998). “Mesozoic and Cenozoic sequence chronostratigraphic chart,” in Mesozoic and Cenozoic sequence stratigraphy of European basins. Editors P. De Graciansky, J. Hardenbol, T. Jacquin, and P. Vail (Tulsa: SEPM Spec . Publ.), 60, 3–13. Charts 1-8.
Hasegawa, H. S., and Basham, P. W. (1989). “Spatial correlation between seismicity and postglacial rebound in eastern Canada,” in Earthquakes at north-atlantic passive margins: neotectonics and postglacial rebound. NATO ASI series (Dordrecht: Springer), 266. doi:10.1007/978-94-009-2311-9_28
Heidbach, O., Rajabi, M., Reiter, K., and Ziegler, M. (2016). World stress map 2016, GFZ data serv. doi:10.5880/WSM2016002
Herbert, T. D., Lawrence, K. T., Tzanova, A., Cleavel, L., Peterson, L., Caballero-Gill, R., et al. (2016). Late Miocene global cooling and the rise of modern ecosystems. Nat. Geos 9, 843–847. doi:10.1038/ngeo2813
Hillis, R. R. (1995). “Regional Tertiary exhumation in and around the United Kingdom,” in Basin inversion, geol. Soc. Editors J. G. Buchanan, and P. G. Buchanan (London: London Spec Publ), 88, 167–190.
Hodell, D. A., Crowhurst, S. J., Lourens, L., Margari, V., Nicolson, J., Rolfe, J. A., et al. (2023). A 1.5-million-year record of orbital and millennial climate variability in the North Atlantic. Clim. Past. 19, 607–636. doi:10.5194/cp-19-607-2023
Jammes, S., Lavier, L., and Manatschal, G. (2010). Extreme crustal thinning in the Bay of Biscay and the western pyrenees: from observations to modeling. Geophys Geosyst 11, Q10016. doi:10.1029/2010GC003218
Japsen, P., Green, P. F., Bonow, J. M., Nielsen, T. F. D., and Chalmers, J. A. (2014). From volcanic plains to glaciated peaks: burial, uplift and exhumation history of southern East Greenland after opening of the NE Atlantic. Glob. Planet. Change 116, 91–114. doi:10.1016/j.gloplacha.2014.01.012
Jomard, H., Cushing, E. M., Palumbo, L., Baize, S., David, C., Chartier, T., et al. (2017). Transposing an active fault database into a seismic hazard fault model for nuclear facilities—Part 1: building a database of potentially active faults (BDFA) for metropolitan France. Nat. Hazards Earth Syst. Sci. 17 (9), 1573–1584. doi:10.5194/nhess-17-1573-2017
Kirby, E., and Whipple, K. X. (2001). Quantifying differential rock-uplift rates viastream profile analysis. Geology 29 (5), 415–418. doi:10.1130/0091-7613(2001)
Krijgsman, W., Capella, W., Simon, D., Hilgen, F. J., Kouwenhoven, T. J., Meijer, P. T., et al. (2018). The Gibraltar Corridor: watergate of the Messinian salinity crisis. Mar. Geol. 403, 238–246. doi:10.1016/j.margeo.2018.06.008
Kristoffersen, Y. (1978). Seafloor spreading and the early opening of the North atlantic. Earth Planet Sci. Lett. 38, 273–290. doi:10.1016/0012-821X(78)90101-2
Lagarde, J. L., Baize, S., Amorèse, D., Delcaillau, B., Font, M., and Volant, P. (2000). Active tectonics, seismicity, and geomorphology with special reference to Normandy (France). J. Quat. Sci. 15, 745–758. doi:10.1002/1099-1417(200010)15:7<745::aid-jqs534>3.0.co;2-6
Lagoe, M. B., Eyles, C. H., and Eyles, N. (1989). Paleoenvironmental significance of foramIniferal biofacies in the glaciomarine yakataga formation, middleton Island, gulf of Alaska. J. Foram. Res. 19, 194–209. doi:10.2113/gsjfr193194
Landais, A., Dreyfus, G., Capron, E., Masson-Delmotte, V., Sanchez-Goni, M., Desprat, S., et al. (2010). What drives the millennial and orbital variations of δ18Oatm? Quat. Sci. Rev. 29 (1-2), 235–246. doi:10.1016/j.quascirev.2009.07.005
Laurent, M. (1993). Datation par résonance de spin électronique (ESR) de quartz de formations quaternaires: comparaison avec le paléomagnétisme. [PhD thesis] [France] Muséum d’Hist. nat. Paris and Univ. Rennes 1.
Laurent, M., Falguères, C., Bahain, J. J., Rousseau, L., and Van Vliet Lanoë, B. (1998). ESR dating of quartz extracted from Quaternary and Neogene sediments: method, potential and limits. Quat. Geochron. 17, 1057–1062. doi:10.1016/s0277-3791(97)00101-7
Lautridou, J. P., (1985). Le cycle périglaciaire pléistocène en Europe du Nord-Ouest et plus particulièrement en Normandie. [ Habil. Thesis], [France] Univ. Caen, 908 pp.
Le Douaran, S., Needham, H. D., and Francheteau, J. (1982). Pattern of opening rates along the axis of the Mid-Atlantic Ridge. Nature 300, 254–257. doi:10.1038/300254a0
Lefort, J. P. (1975). Le socle péri-armoricain, étude géologique et géophysique du socle submergé à l’Ouest de la France, [PhD thesis] , 1. [France]: Univ. Rennes, 250.
Le Gall, B., Authemayou, C., Ehrhold, A., Paquette, J. L., Bussien, D., Chazot, G., et al. (2014). LiDAR offshore structural mapping and U/Pb zircon/monazite dating of Variscan strain in the Leon metamorphic domain, NW Brittany. Tectonophysics 630, 236–250. doi:10.1016/j.tecto.2014.05.026
Lenôtre, N., Thierry, P., Blanchin, R., and Brochard, G. (1999). Current vertical movement demonstrated by comparative levelling in Brittany (northwestern France). Tectonophysics 301, 333–344. doi:10.1016/j.tecto.2014.05.026
Lericolais, G., Auffret, J. P., Bourillet, J. F., Berne, S., Guennoc, P., Le Drezen, E., et al. (1995). L’énigmatique Fosse Centrale de la Manche: une approche de sa morphologie et de son remplissage par géophysique haute résolution. C.R.Acad. Sci. Paris - Ser. IIA - Earth Planet. Sci. 321, 39–46. Available at: https://archimer.ifremer.fr/doc/00071/18205/.
Le Roy, P., Gracia-Garay, C., Guennoc, P., Bourillet, J.-F., Reynaud, J.-Y., Thinon, I., et al. (2011). Cenozoic tectonics of the western approaches channel basins and its control of local drainage systems. Bull. Soc. Géol. Fr. 182, 451–463. doi:10.2113/gssgfbull1825451
Liebrand, D., Lourens, L. J., Hodell, D. A., de Boer, B., van de Wal, R. S. W., and Pälike, H. (2011). Antarctic ice sheet and oceanographic response to eccentricity forcing during the early Miocene. Clim. Past. 7, 869–880. doi:10.5194/cp-7-869-2011
Liesa, C. L., Rodríguez-López, J. P., Ezquerro, L., Alfaro, P., Rodríguez-Pascua, M. Á., Lafuente, P., et al. (2016). Facies control on seismites in an alluvial–aeolian system: the Pliocene dune field of the Teruel half-graben basin (eastern Spain). Sed. Geol. 344, 237–252. doi:10.1016/j.sedgeo.2016.05.009
Lisiecki, L. E., and Raymo, M. E. (2005). A Pliocene-Pleistocene stack of 57 globally distributed benthic d18O records. Paleoceanography 20, PA1003. doi:10.1029/2004PA001071
Madsen, H. B., and Knudsen, K. L. (1994). Recent foraminifera in shelf sediments of the Scoresby Sund fjord, East Greenland. Boreas 23, 495–504. doi:10.1111/j.1502-3885.1994.tb00617.x
Magyari, Á., Musitz, B., Csontos, L., Unger, Z., and Van Vliet-Lanoë, B. (2005). Quaternary neotectonics of the Somogy Hills, Hungary (part I): evidence from field observations. Tectonophysics 410, 43–62. doi:10.1016/j.tecto.2005.05.044
Mangerud, J., Jansen, E., and Landvik, J. (1996). Late cenozoic history of the scandinavian and barents sea ice sheets. Glob. Planet. Change 12, 11–26. doi:10.1016/0921-8181(95)00009-7
Marincovich, L., and Gladenkov, A. (1999). Evidence for an early opening of the Bering Strait. Nature 397, 149–151. doi:10.1038/16446
Martín-Chivelet, J., López-Gómez, J., Aguado, R., et al. (2019). “The late jurassic–early cretaceous rifting,”. The geology of Iberia: a geodynamic approach. Editors Quesada,, and J. T. Oliveira (Springer), 5, 169–249. Region Geol. Rev. doi:10.1007/978-3-030-11295-0_556
Martinez, F., Hey, R., and Höskuldsson, Á. (2020). Reykjanes Ridge evolution: effects of plate kinematics, small-scale upper mantle convection and a regional mantle gradient. Earth Sci. Rev. 206, 102956. doi:10.1016/j.earscirev.2019.102956
Mazabraud, Y., Béthoux, N., Guilbert, J., and Bellier, O. (2005). Characterisation of the seismological pattern in a slowly deforming intraplate region: central and western France. Tectonophysics 409, 75–192. doi:10.1111/j.1365-246X.2004.02430.x
Mazzotti, S., Jomard, H., and Masson, F. (2020). Processes and deformation rates generating seismicity in metropolitan France and conterminous Western Europe. BSGF - Earth Sci. Bull. 191, 19. doi:10.1051/bsgf/2020019
McCalpin, J. (2009). in Paleoseismology –. Inter. Geophys. Series. Editors R. Dmowska, D. Hartmann, and H. T Rossby. 2nd ed., 95, 613.
Mehl, C., Jolivet, L., and Lacombe, O. (2005). From ductile to brittle: evolution and localization of deformation below a crustal detachment (Tinos, Cyclades, Greece). Tectonics 24, TC4017. doi:10.1029/2004TC001767
Miall, A. D. (2010). The geology of stratigraphic sequences. 2d edition. BerlIn: Springer-Verlag, 522pp.
Miller, K. G., Browning, J. V., Schmelz, W. J., Kopp, R. E., Mountain, G. S., and Wright, J. D. (2020). Cenozoic sea-level and cryospheric evolution from deep-sea geochemical and continental margin records. Sci. Adv. 6, 1346. doi:10.1126/sciadv.aaz1346
Miller, S. R., Sak, P. B., Kirby, E., and Bierman, P. R. (2013). Neogene rejuvenation of central Appalachian topography: evidence for differential rock uplift from stream profiles and erosion rates Earth Planet. Sci. Lett., 369–370. doi:10.1016/jepsl201304007
Moguedet, G., Marchand, Y., Masson, V., Papin, H., Vauthier, S., Charnet, F., et al. (2000). Notice, carte géol France (1/50 000), sheet La loupe (253), Orléans, BRGM, 102 pp.
Monnier, J.-L., Hallegouët, B., Hinguant, S., Laurent, M., Auguste, P., Bahain, J.-J., et al. (1994). A new regional group of the Lower Palaeolithic m Brittany (France), recently dated by Electron Spin Resonance. C.R. Acad. Sci. Paris 319 (2), 155–160.
Montes, C., Cardona, A., Jaramillo, C., Pardo, A., Silva, J. C., Valencia, V., et al. (2015). Middle Miocene closure of the central American seaway. Science 348 (62 31), 226–229. doi:10.1126/science.aaa2815
Morzadec-Kerfourn, M. T. (1982). Datation pollInique et conditions de sédimentation de l’argile plio-pléistocène de Lanrinou en Landerneau (Finistère, France). Bull. Ass. Fr. étude Quat. (AFEQ) 19 (12), 179–184. doi:10.3406/quate.1982.1436
Mosar, J., Lewis, G., and Torsvik, T. (2002). North Atlantic seafloor spreading rates: implications for the Tertiary development of Inversion structures of the Norwegian–Greenland Sea. J. Geol. Soc. Lond. 159, 503–515. doi:10.1144/0016-764901-135
Müller, R. D., Sdrolias, M., Gaina, C., and Roest, W. R. (2008). Age, spreading rates, and spreading asymmetry of the world’s ocean crust. Geochem. Geophys. Geosyst. 9, Q04006. doi:10.1029/2007GC001743
Néraudeau, D. (2007). Neogene bioaccumulations (algal limestones, ‘faluns’) from western Europe and their relationship with the Messinian crisis. C. R. Palevol 6 (1–2), 59–71. doi:10.1016/j.crpv.2006.12.002
Néraudeau, D., Barbe, S., Mercier, D., and Roman, J. (2003). Signatures paléoclimatiques des échinides du Néogène final atlantique à faciès redonien. Ann. Paléonto. 89, 153–170. doi:10.1016/s0753-3969(03)00023-5
Néraudeau, D., Senan, D., and Dudicourt, J. C. (2011). Les faluns du Miocène moyen de Bretagne in Fossiles. Rev. Fr. Paléontol. 8, 36–38.
Ogg, J. G., Ogg, G., and Gradstein, F. M. (2016). A concise geologic time scale. Amsterdam: Elsevier, 240.
Olson, S., Green, R., and Obermeier, S. F. (2005). Revised magnitude-bound relation for the Wabash Valley seismic zone of the Central United States. Seismo. Res. Lett. 76, 756–771. doi:10.1785/gssrl.76.6.756
Paquet, F., Menier, D., Estournès, G., Bourillet, J. F., Le Roy, P., and Guillocheau, F. (2010). Buried fluvial incisions as a record of middle-late Miocene eustasy fall on the armorican shelf (Bay of Biscay, France). Mar. Geol. 268, 137–151. doi:10.1016/j.margeo.2009.11.002
Paquet, F., Thinon, I., Dugué, O., Tessier, B., Benabdellouahed, M., Lasseur, E., et al. (2023). The Central English Channel troughs: major source-to-sink remnants or giant tidal scours? Mar. Petrol. Geol. 153, 106303. doi:10.1016/j.marpetgeo.2023.106303
Parizot, O., Missenard, Y., Haurine, F., Blaise, T., Barbarand, J., Benedicto, A., et al. (2021). When did the Pyrenean-shortening end? Insight from U–Pb geochronology of syn-faulting calcite (Corbières area, France). Terra nova. 33 (6), 551–559. doi:10.1111/ter12547
Pedoja, K., Jara-Muñoz, J., De Gelder, G., Robertson, J., Meschis, M., Fernandez-Blanco, D., et al. (2018). Neogene - quaternary slow coastal uplift of western Europe through the perspective of sequences of strandlines from the Cotentin peninsula (Normandy, France). J. Geomorph. 303, 338–356. doi:10.1016/j.geomorph.2017.11.021
Peltier, W. R. (1999). Global sea level rise and glacial isostatic adjustment. Glob. Planet. Change 20, 93–123. doi:10.1016/s0921-8181(98)00066-6
Pérez, L. F., Nielsen, T., Knutz, P. C., Kuijpers, A., and Damm, V. (2018). Large-scale evolution of the central-east Greenland margin: new Insights to the North Atlantic glaciation history. Glob. Planet. Change 163, 141–157. doi:10.1016/jgloplacha201712010
Perrot, J., Arroucau, P., Guilbert, J., Déverchère, J., Mazabraud, Y., Rolet, J., et al. (2005). Analysis of the Mw 4.3 Lorient earthquake sequence: a multidisciplinary approach to the geodynamics of the Armorican Massif, westernmost France. Geophys. J. Inter. 162, 935–950. doi:10.1111/j.1365-246X.2005.02706.x
Petrillo, G., Lippiello, E., Landes, F. P., and Rosso, A. (2020). The Influence of the brittle-ductile transition zone on aftershock and foreshock occurrence. Nat. Commun. 11, 3010. doi:10.1038/s41467-020-16811-7
Purkey, S. G., Johnson, G. C., and Chambers, D. P. (2014). Relative contributions of ocean mass and deep steric changes to sea level rise between 1993 and 2013. J. Geophys. Res. Oceans 119, 7509–7522. doi:10.1002/2014JC010180
Quan, C., Liu, Y. S., Tang, H., and Utescher, T. (2014). Miocene shift of European atmospheric circulation from trade wind to westerlies. Sci. Rep. 4, 5660. doi:10.1038/srep05660
Regard, V., Vacherat, A., Bonnet, S. V., Mouthereau, F., Nørgaard, J., and Knudsen, M. (2021). Late pliocene-pleistocene incision in the Ebro BasIn (north Spain). Bull. Soc. Géol. Fr. 192 (1), 30. doi:10.1051/bsgf/2021020
Renouf, J. T. (1993). “Solid geology and tectonic background,” in Quaternary of Jersey: field guide. Editor D. H. Keen (London: Quat. Res. Asso.), 1–11.
Resig, J. M. (1964). The southernmost occurrence of Elphidiella hannai (Cushman and Grant), 1927, off the west coast of North America. Paleontology 38, 393–396.
Rouby, D., Braun, J., Robin, C., Dauteuil, O., and Deschamps, F. (2013). Long-term stratigraphic evolution of Atlantic-type passive margins: a numerical approach of Interactions between surface processes, flexural isostasy, and 3D thermal subsidence. Tectonophysics 604, 83–103. doi:10.1016/j.tecto.2013.02.003
Rowley, D. B. (2013). Sea level: earth’s dominant elevation—implications for duration and magnitudes of Sea level variations. J. Geol. 121, 445–454. doi:10.1086/671392
Ruszkiczay-Rüdiger, Z., Balázs, A., Csillag, G., Drijkoningen, G., and Fodor, L. (2020). Uplift of the transdanubian range, pannonian basin: how fast and why? Glob. Planet. Change 192, 103263. doi:10.1016/j.gloplacha.2020.103263
Ruszkiczay-Rüdiger, Z., Csillag, G., Fodor, L., Braucher, R., Novothny, Á., Thamó-Bozsó, E., et al. ASTER Team (2018). Integration of new and revised chronological data to constrain the terrace evolution of the Danube River (Gerecse Hills, Pannonian Basin). Quat. Geochron. 48, 148–170. doi:10.1016/j.quageo.2018.08.003
Schouten, S., Eldrett, J. S., Greenwood, R. J., Sinninghe-Damste, J. S., and Baas, M. (2008). Onset of long-term cooling of Greenland near the Eocene-Oligocene boundary as revealed by branched tetraether lipids. Geology 36, 147–150. doi:10.1130/G24332A1
SHARE (2013). SHARE (seismic hazard harmonization in Europe). Editor D. Giardini, J. Woessner, and L. Danciu (2014). Mapping Europe’s seismic hazard. EOS 95 (29), 261–262. http://wwwSHARE-euorg.
SHOM (2015). Marnages sur les côtes françaises de La Manche et de l’Atlantique pour le coefficient 95. https://servicesdatashomfr:/geonetwork/srv/api/records/MAREE_COURANTS_MARNAGES_MGA_VExml.
Sibson, R. H. (1990). Rupture nucleation on unfavorably oriented faults. Bull. Seism. Soc. Am. 80 (6A), 1580–1604. doi:10.1785/BSSA08006A1580
SisFrance BRGM-EDF-IRSN/SisFranc (2010). Histoire et caractéristiques des séismes ressentis en France. http://wwwsisfrancenet.
Somoza, L., Medialdea, T., Terrinha, P., Ramos, A., and Vázquez, J. T. (2021). Submarine active faults and morpho-tectonics around the Iberian margins: seismic and tsunamis hazards. Front. Earth Sci. 9, 653639. doi:10.3389/feart.2021.653639
Srivastava, S. P., and Verhoef, J. (1992). Evolution of mesozoic sedimentary basIns around the North central atlantic: a preliminary plate kinematic solution, in basins on the atlantic seaboard. IN J. Parnell (edit) “Petroleum geology, sedimentology and basin evolution”. London: Geolological Society, London Special Publublication, 62: 397–420. doi:10.1144/GSL.SP.1992.062.01.30
Steinthorsdottir, M., Coxall, H. K., de Boer, A. M., Huber, M., Barbolini, N., Bradshaw, C., et al. (2020). The Miocene: the future of the past. Paleoceano. Paleoclim. 36 (4), e2020PA004037. doi:10.1029/2020PA004037
Stoker, M. S., Praeg, D., Shannon, P. M., Hjelstuen, B. O., Laberg, J. S., Nielsen, T., et al. (2005). Neogene evolution of the Atlantic continental margin of NW Europe (Lofoten Islands to SW Ireland): anything but passive. Geol.Soc., Lond. Petrol. Geol.Conf. Ser. 6, 1057–1076. doi:10.1144/0061057
Thinon, I., Idalgo, F., Gonzalez, L., Rehault, J. P., and Olivet, J. L. (2001). Déformations pyrénéennes dans le golfe de Gascogne. C.R. Acad. Sci. Paris 332, 561–568. doi:10.1016/S1251-8050(01)01576-2
Tripati, A., and Darby, D. (2018). Evidence for ephemeral middle Eocene to early Oligocene Greenland glacial ice and pan-Arctic Sea ice. Nat. Com. 9 1038, 1038. doi:10.1038/s41467-018-03180-5
Utescher, T., Erde, I. B., François, L., and Mosbrugger, V. (2007). Tree diversity in the Miocene forests of western Eurasia. Palaeogeo. Palaeoclim. Palaeoecol. 253, 226–250. doi:10.1016/j.palaeo.2007.03.041
Vandenberghe, J., Xianyan, W., and Vandenberghe, D. (2016). Very large cryoturbation structures of last permafrost maximum age at the foot of the qilian Mountains (NE tibet plateau, China). Perm. Perigl. Proc. 27, 138–143. doi:10.1002/ppp1847
Vandenberghe, N. (2017). Tectonic and climatic signals in the Oligocene sediments of the southern North-sea basin. Geol. Belg. 20, 105–123. doi:10.20341/gb.2017.007
Vandenberghe, N., Laga, P., Steurbaut, E., Hardenbol, J., and Vail, P. R. (1998). “Tertiary sequence stratigraphy at the southern border of the North Sea Basin in Belgium,”. Mesozoic and cenozoic sequence stratigraphy of European basins. Editors P. C. de Graciansky, J. Hardenbol, T. Jacquin, and P. R. Vail (Claremore, United States: SEPM Spec. Pub), 60, 119–154.
Van Loon, A. J. (2009). Soft-sediment deformation structures in siliciclastic sediments: an overview. Geologos 15, 3–55.
Van Sickel, W. A., Kominz, M. A., Miller, K. G., and Browning, J. V. (2004). Late Cretaceous and Cenozoic sea-level estimates: backstripping analysis of borehole data, onshore New Jersey. Basin Res. 16, 451–465. doi:10.1111/j.1365-2117.2004.00242.x
Van Vliet-Lanoë, B. (1987). « Le rôle de la glace de ségrégation dans les formations superficielles de l’Europe de l’Ouest. Processus et héritages ». Caen: Univ. Paris I. Publ. Editec, 854pp. [Habil Thesis] [France].
Van Vliet-Lanoë, B., Authemayou, C., Molliex, S., Field, M., Frechen, M., Le Roy, P., et al. (2019). Quaternary surface shale diapirism induced by intraplate low seismic events: the study case of Western Brittany (France). Quat. Res. 91, 1–24. doi:10.1017/qua.2018.63
Van Vliet-Lanoë, B., Bonnet, S., Hallégouët, B., and Laurent, M. (1997). Neotectonic and seismic activity in the Armorican and Cornubian Massifs: regional stress field with glacio-isostatic Influence? J. Geodyn. 2, 219–239. doi:10.1016/S0264-3707(96)00035-X
Van Vliet-Lanoë, B., Brulhet, J., Combes, P., Duvail, C., Ego, F., Baize, S., et al. (2017). Quaternary thermokarst and thermal erosion features in northern France: origin and palaeoenvironments. Boreas 46, 442–461. doi:10.1111/bor.12221
Van Vliet-Lanoë, B., and Guillocheau, F. (1995). Evolution de l’enregistrement pédo-sédimentaire depuis 150 ka en France du NO et en Belgique: biorhexistasie et bilans sédimentaires. C. R. Acad. Sc. Paris IIa 320, 419–426.
Van Vliet-Lanoë, B., and Guillou, H. (2021). “Cenozoic evolution of Iceland and the cryosphere,” in Iceland within the northern atlantic 2: interactions between volcanoes and glaciers. Editor B. Van Vliet-Lanoe (Wiley), 103–191. doiorg/. doi:10.1002/9781119850892ch3
Van Vliet-Lanoë, B., Helluin, M., Pellerin, J., and Valadas, B. (1992). in “Soil erosion in western Europe: from the last interglacial to the present” in past and present erosion. Editors M. Bates,, and J. Boardman (Paris; Oxbow Publ), 101–114.
Van Vliet-Lanoë, B., Hérisson, D., Dabrowski, E., Authemayou, C., Frechen, M., Hallégouët, B., et al. (2021). Le gisement paléolithique inférieur de Pen Hat (Crozon, Bretagne) et son contexte stratigraphique régional. Quaternaire 32/1, 61–93. doi:10.4000/quaternaire.15038
Van Vliet-Lanoë, B., Hibsch, C., Csontos, L., Jegouzo, S., Hallégouët, B., Laurent, M., et al. (2009). Seismically induced shale diapirism: the Mine d’Or section, Vilaine estuary, Southern Brittany. Inter J. Earth Sci. 98, 969–984. doi:10.1007/s00531-007-0295-7
Van Vliet-Lanoë, B., Laurent, M., Hallegouët, B., Margerel, J. P., Chauvel, J. J., Michel, Y., et al. (1998a). Le mio-pliocène du Massif armoricain. Données nouvelles. C.R.Acad Sc. 326, 333–340. doi:10.1016/s1251-8050(98)80303-0
Van Vliet-Lanoë, B., Maygari, A., and Meilliez, F. (2004). Distinguishing between tectonic and periglacial deformations of quaternary continental deposits in Europe. Glob. Planet. Change 43, 103–1270. doi:10.1016/j.gloplacha.2004.03.003
Van Vliet-Lanoë, B., Pellerin, J., and Chauvel, J. J. (1998b). “Le bassin du Coët-Dan au coeur du Massif armoricain,” in Agriculture Intensive et qualité des eaux. Editor C. Cheverry (INRA: publ), 11–24.
Van Vliet-Lanoë, B., Vandenberghe, N., Laignel, B., Laurent, M., Lauriat-Rage, A., Louwye, S., et al. (2002). Paleogeographic evolution of the western Europe during the upper cenozoic. Geodiversitas 24 (3), 511–541. doi:10.1016/j.crte.2015.01.001
Vernhet, Y. (2003). “Carte géologique harmonisée du département de la Manche. Rapport fInal. » Notice technique BRGM IRP-52715-FR décembre 2003, 228 pp., 1 pl. hors texte. http://Infoterre.brgm.fr/rapports/RP-52715-FR.pdf
Voinchet, P., Despriée, J., Gageonnet, R., Bahain, J. J., Tissoux, H., Falguères, C., et al. (2007). ESR dating of fluvial quartz from the Middle Loire basin (Centre region, France): evidence of the impact of Quaternary tectonics in the fluvial terraces system geometry. Quaternaire 18 (4), 335–347. doi:10.4000/quaternaire.1216
Worum, G., and Michon, L. (2005). Continuous, syn-sedimentary basin inversion induced by the alpine compression: the Paleogene inversion of the west Netherlands basin integrated into a NW European scale. Bull. Geol. Soc. Lond. 162, 73–85. doi:10.1144/0016-764904-011
Wyns, R. (1991). Évolution tectonique du bâtit armoricain au Cénozoïque d’après l’analyse des paléosurfaces continentales et des formations géologiques associées. Géol. Fr. 3, 11–42.
Wyns, R., Quesnel, F., Simon-Coinçon, R., Guillocheau, F., and Lacquement, F. (2003). Major weathering in France related to lithospheric deformation. Géol. Fr. 1, 79–87.
Xia, G., Wu, C., Rodríguez-López, J. P., Mansour, A., Jin, X., Pei, J., et al. (2021). Eocene-Oligocene terrestrial cryospheric processes: bipolar glaciation and uplifted Tibet. Res. Sq. 17. doi:10.21203/rs.3.rs-835656/v1
Zachos, J., Pagani, M., Sloan, L., Thomas, E., and Billups, K. (2001). Trends, rhythms, and aberrations in global climate 65 Ma to present. Science 292 (5517), 686–693. doi:10.1126/science.1059412
Keywords: paleoseismicity, sea level, climate, uplift, erosion, tectonic
Citation: Van Vliet-Lanoë B, Authemayou C, Le Roy P, Renouf JC, Combes P and Ego F (2024) Neogene and Pleistocene geodynamics: the paleoseismic evolution of Armorica (Western France). Front. Earth Sci. 11:1269598. doi: 10.3389/feart.2023.1269598
Received: 30 July 2023; Accepted: 27 November 2023;
Published: 19 January 2024.
Edited by:
Marco Meschis, Instituto Nazionale di Geofisica e Vulcanologia (Sezione Palermo)–INGV, ItalyReviewed by:
Nicolò Parrino, University of Palermo, ItalySalvatore Gambino, University of Catania, Italy
Copyright © 2024 Van Vliet-Lanoë, Authemayou, Le Roy, Renouf, Combes and Ego. This is an open-access article distributed under the terms of the Creative Commons Attribution License (CC BY). The use, distribution or reproduction in other forums is permitted, provided the original author(s) and the copyright owner(s) are credited and that the original publication in this journal is cited, in accordance with accepted academic practice. No use, distribution or reproduction is permitted which does not comply with these terms.
*Correspondence: Brigitte Van Vliet-Lanoë, YnJpZ2l0dGVsYW5vZTRAZ21haWwuY29t