- School of Civil Engineering and Architecture, Wuhan University of Technology, Wuhan, China
Based on practical construction experience, it is observed that a significant number of rockburst-prone regions exhibit structural surfaces of varying scales that exert a controlling influence on rockburst. Therefore, it is crucial to review the progress and hotspots in research on structure rockburst from a macroscopic perspective. This paper utilized CiteSpace software to summarize the references on structure rockburst research from the Web of Science Core Collection database from 2003 to 2022. The results include keyword cooccurrence and evolution analysis, and co-citation and cluster analysis. Through the in-depth analysis of structure rockburst literature, the research progress of structure rockburst in indoor experiments, numerical simulation, and on-site micro-seismic monitoring progress, the development trend and research hotspots were evaluated, and the current structure rockburst was discussed. The shortcomings of current research on structure rockbursts are discussed, and future development directions are proposed from the perspectives of structure rockburst mechanism, prediction and prevention measures.
1 Introduction
Rockburst is a geological hazard that occurs during underground construction in areas of high ground stress and is encountered widely in deeply buried mines, mountain tunnels, and water and hydropower projects. Currently, there is no uniform definition of rockburst, which can be interpreted as a nonlinear kinetic phenomenon of instantaneous release of energy from an energetic rock mass along the excavation critical surface. A rockburst can occur instantaneously or continuously after the excavation of an underground chamber has been completed. The macroscopic manifestations of rockbursts are characterized by the production of bursting, particle ejection and even throwing phenomena. Due to its sudden, unpredictable nature, a rockburst poses a serious threat to both the safety of equipment and personnel, as well as the progress of the project. Thus, it is critical to investigate the mechanisms and conditions of rockburst in complex geological environments and accurately predict their occurrence (Keneti and Sainsbury, 2018; Ghasemi et al., 2019; Liu et al., 2020; Zhang et al., 2022).
Initially, it was believed that rockbursts only occurred in tunnels with high ground stress and intact surrounding rocks. However, practical construction experience has revealed that a significant number of rockburst-prone regions exhibit structural surfaces of varying scales (Zhang et al., 2022). In fact, the mechanism of rockburst occurrence in tunnels with developed joints is not completely the same as that in tunnels with intact surrounding rocks. Conventional rockburst prediction methods such as microseismic monitoring and acoustic emission monitoring, as well as control measures such as local blasting and rockburst point pre-treatment, are not entirely suitable for tunnels with developed joints (Feng et al., 2019; Zhao, 2021; Fang et al., 2023). Structure rockburst was proposed by American geologist George Richard Gardner in the 1960s. He studied the mechanism of rockburst and found that when there is a certain structural surface in the rock, the rock is more prone to damage, and this phenomenon is known as structure rockburst. Later, this theory was widely applied and became one of the important research areas in mining and tunneling engineering. In recent years, with the large-scale development of infrastructure construction in China, Chinese scholars have done a lot of research on the damage process of rock enclosures containing structural faces such as joints and fissures, and the mechanism of rockburst occurrence (Barton et al., 2023; Wang et al., 2023). Feng et al. (2013) proposed a classification of rockburst based on their spatial and temporal manifestation characteristics, dividing them into immediate and time-lagged rockburst. Immediate rockburst can be further subdivided into immediate strain rockburst and immediate strain-structure face slip rockburst. Tang et al. (2002) investigated the incubation and rupture processes of laminated rocks until the critical state of rockburst, highlighting the significant influence of structural surfaces on the mode of damage and instability of the surrounding rock. They found that the final damage state of the rock varied based on the nature of the structural surface. Zhou et al. (2015a) observed that the fracture surface of the surrounding rock slab and the structural surface cut the surrounding rock, forming a rupture structure that created potential for rockburst. According to the rockburst classification system summarized by He et al. (2022), as shown in Figure 1, structure rockburst is classified as strain-type rockbursts and fault slip-type rockbursts, and the common damage forms of structure rockburst are buckling damage, shear damage, and fault slip damage. Structure rockburst has been studied mainly through catastrophe theory (He et al., 2022). Currently, many scholars (Zhou et al., 2015b; Feng et al., 2019) believe that the presence of structural faces affects the intensity and damage extent of rock bursts, and that the catastrophic mechanism of structure rockburst is not equivalent to that of strain rockburst, so it is necessary to examine the progress of structure rockburst research from a macroscopic perspective.
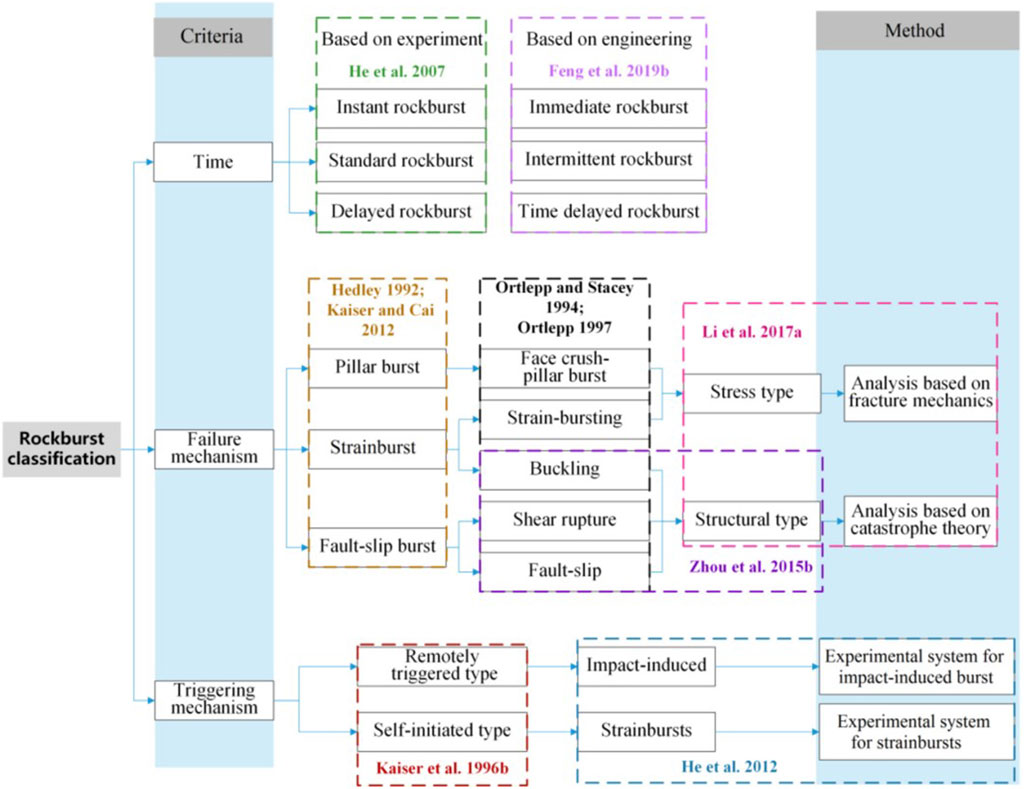
FIGURE 1. Classification of rockburst (He et al., 2022).
The CiteSpace software is a citation visualization tool created for scientometrics and data visualization purposes. It enables researchers to explore various aspects of a research field, such as hotspots, frontiers, key references, authors, and institutions, among others, and facilitates predictions of future development in structure rockburst research. CiteSpace offers multiple visualization views, including the cluster, timeline, and time zone views, which are primarily employed for co-citation analysis of reference. These views offer distinct perspectives on the distribution of structure rockburst research content, while the timeline and time zone views are particularly useful in presenting trends and interactions related to structure rockburst research over time. Additionally, CiteSpace software can assist in predicting future research directions and aid in decision-making processes. This paper presents a bibliometric review of structure rockburst research conducted between 2003 and 2022, using CiteSpace software to comprehensively analyze the existing body of knowledge in the field. The review includes keyword co-occurrence and evolution analysis, and co-citation and cluster analysis. Additionally, a summary of the current state of rockburst research is provided, along with an analysis of research hotspots and trends.
2 Scientometric principles and research methods
2.1 Scientometric principles
Citespace is an information visualization software that is developed using the Java programming language. It is designed to display the structure, patterns, and evolution of literature, while also providing an in-depth analysis of citation information. The software offers various function options, such as cooperation mapping of cited references, co-occurrence network, and co-citation network of cited references. Additionally, Citespace provides co-occurrence analysis of keywords, which allows for a comprehensive understanding of the development direction and research trend of a specific research topic over a specific period. With these capabilities, researchers can explore the development trend and hotspots of a research field, ultimately improving the quality and depth of their research analysis (Chen et al., 2009).
The analysis of prevalent node types, namely Author, Institution, and Country, in CiteSpace software, is facilitated by the utilization of co-authorship. These three types of nodes have different subject granularity in analyzing cooperation, i.e., micro to macro variation in the degree of cooperation. CiteSpace software has three types of co-citation nodes, namely Cited Reference, Cited Author, and Cited Journal. These nodes are mainly utilized for co-citation analysis and visualization of the distribution of research objects. The software offers three co-citation algorithms, which include the Cosine method, Jaccard method, and Dice method. The default algorithm utilized by the software is the Cosine method, which is the most widely used. The process of the Cosine algorithm can be understood as the normalization process of the co-occurrence matrix (Chen, 2006; Chen et al., 2009).
2.2 Research methods
Indexed databases form the fundamental basis for bibliometric analysis, and the data format of CiteSpace software is aligned with the Web of Science database. The Web of Science database is the most comprehensive literature retrieval tool currently available, and as such, it was used to gather literature on structure rockburst research. The search term for this literature search was determined as follows: TS (Topic Search) = (structural rockburst*), where the "*" character indicates the use of fuzzy search. By using this search term, the software was able to identify relevant literature and perform an in-depth bibliometric analysis, providing valuable insights into the research field of structure rockburst. When utilizing CiteSpace software for retrieval, the language, document type, and time range are restricted to “English”, “article AND review”, and “2003–2022″ respectively (with the retrieval date set as 1 December 2022). Using the search strategy mentioned earlier, a total of 384 papers were retrieved from the Web of Science database. As shown in Figure 2. These papers were then filtered and de-duplicated using the built-in Filter function of the CiteSpace software, resulting in a total of 258 unique papers that were used for the visualization study. The CiteSpace software provides several analysis options for the retrieved literature, including keyword co-occurrence and evolution analysis, and co-citation clustering analysis.
3 Hotspot and frontier analysis
3.1 Keyword cooccurrence and evolution analysis
The utilization of keywords is fundamental to the essence of a research paper, as it allows for a concise and representative description of the research content. The use of high-frequency keywords is particularly important as it aids in identifying topical issues within a research area. The construction of a keyword co-occurrence network illustrates the degree of co-occurrence among keywords in the chosen literature. This network provides a means of identifying research hotspots and frontiers. Additionally, by analyzing the evolution of keywords over time, the changing trends of research directions within a field can be comprehended.
3.1.1 Keyword cooccurrence analysis
Figure 3 presents the co-occurrence network of keywords used in structure rockburst studies. The size of each keyword reflects its frequency of occurrence, with “rockburst” and “rock burst” excluded due to their outdated status. Similar keywords were merged to avoid redundancy. The most frequent keywords (occurring over 20 times) are “stress” (50 times), “failure” (45 times), “tunnel” (36 times), “prediction” (36 times), “behavior” (33 times), “rock” (32 times), “mechanism” (32 times), “acoustic emission” (26 times), “model” (26 times), “mine” (26 times), “numerical simulation” (23 times), “fracture” (23 times), and “energy” (21 times). While some synonyms exist, their impact on the overall keyword analysis is negligible.
The data gathered indicates that research on structure rockburst is primarily focused on experimental studies of mechanical properties, numerical simulation model analysis, and prediction methods. Scholars both domestically and internationally have conducted extensive research on rockburst and related issues using various research methods, which has advanced the development of rockburst theory and deepened our understanding of the rockburst process, thereby enhancing construction safety at worksites. However, there is a dearth of research on structure rockburst theory, which is typically based on energy accumulation, model analysis, and kinetic theories used to explain the occurrence mechanism (Li et al., 2017; Zhou et al., 2018).
The higher frequency of the keywords “prediction” and “acoustic emission” is attributable to the fact that current studies on structure rockburst mainly involve site investigation and field monitoring to observe the occurrence process of rockbursts and identify precursor information. Microseismic monitoring and acoustic emission monitoring are reliable methods for field research on structure rockburst (Feng et al., 2012; Zhou et al., 2015c). Xu et al. (2016) established a real-time microseismic monitoring system based on the frequent occurrence of rock bursts during the construction of deeply buried tunnels in Jinping II Hydropower Station, obtained abundant microseismic monitoring data, and concluded that microseismic event aggregation is the precursor information for the occurrence of strain-type rockbursts. Further, Feng G. L. et al. (2018) investigated the effect of the presence of structural surfaces in the diversion tunnel of Jinping II Hydropower Station on rockburst microseismic by in situ microseismic techniques, and showed that rigid structural surfaces affect the occurrence of rockbursts to varying degrees. Relying on the method of on-site microseismic monitoring, Feng G. L. et al. (2018) proposed a microseismic-based rockburst early warning method, which utilizes real-time microseismic monitoring data and established rockburst early warning formulas to provide dynamic early warning of rockburst hazards during tunnel excavation. Studies have shown that rigid structural surfaces can affect the occurrence of rock bursts to varying degrees. Whether it is a moderate rockburst or a strong rockburst, the presence of a structural face changes the way microseismicity evolves because the structural face affects the stress distribution and structure of the surrounding rock mass. The structural face inhibits the transfer of surrounding stresses to the surrounding deep rock mass, resulting in a concentration of stress and energy in the rock mass between the excavation boundary and the structural face.
The high frequency of the keywords “model” and “numerical simulation” suggests that many experts are using numerical simulation to study structure rockburst. For instance, Feng F. et al. (2018) employed the combined finite element method ELFEN to investigate the mechanical response of structural faces in circular tunnels under unloading conditions by considering parameters such as the structural face inclination, location, and lateral pressure coefficient. Wang et al. (2021) conducted a numerical simulation on six groups of structure rockbursts with inclined angles of 60° using UDEC. The research found that as the number of structural surfaces increased to 2-3, the local energy release rate increased, and the range of rockburst destruction also increased. As the number of structural surfaces continues to increase, the local energy release rate shows a trend of reducing first and then increasing. The range of rock burst destruction linearly increases. The rockburst destruction area is always adjacent to the intersection area of the structural surface and the air surface, and the energy released during shear destruction is always greater than that during tensile destruction, indicating that shear destruction is the primary form of rockburst destruction for structure rockburst. The study (Wang et al., 2021) is consistent with the research of Zhou et al. (2015a) where the bedding plane and structural surfaces cooperate to create a fault structure through cutting through the rock mass, which becomes a potential form of rockburst. Similarly, Zhang et al. (2012) found through numerical simulation that the existence of structural surfaces blocks the process of stress adjustment in the deep rock mass, leading to stress concentration and continuous accumulation of energy between the excavation boundary and the structural surface. Some scholars (Zhou et al., 2015b) have proposed that the impact mechanism of structural surfaces on rockburst is different at different locations and orientations, especially for inclined structural surfaces, which have a high probability of experiencing shear or shear-tensile failure, thus inducing slide-type or shear fracture-type rockbursts.
In particular, the keyword “energy” is worth noting. This is because the occurrence of rockburst is essentially a process of sudden and rapid release of energy stored in the surrounding rock. For rock bodies containing structural surfaces, energy is accumulated in large quantities in the structural surface. Therefore, analyzing the structure rockburst problem from an energy perspective will be closer to the mechanism and nature of its occurrence. Most scholars recognize energy analysis as the most effective research method to analyze rockburst and other deep rock dynamic hazards. Some scholars have proposed empirical rockburst energy criteria, such as energy storage and consumption index and energy ratio method, based on the energy stored and released during the process of rock damage (Meng et al., 2017; He M. C. et al., 2018). According to Hou et al. (2016), the essence of rock destruction is the transformation of energy, and they proposed a new brittleness evaluation method by utilizing the energy evolution law of rock destruction and the acoustic emission energy. Through experimental verification, the new brittleness evaluation method was found to be reliable and effective. Jiang et al. (2017) conducted a three-way six-sided loading and then single-sided unloading test on barite, and the results showed that the more elastic strain energy released during rock sample damage, the more violent the damage process, and the more total mass and particle size of the ejected debris. Currently, there is a lack of research on the energy evolution process of structure rockburst in indoor experiments. Structure-type rock masses are anisotropic materials, and the impact of the inclination angle of the structural surface on the energy evolution law requires further in-depth research and exploration. Furthermore, from the perspective of energy, developing a layer-type rock mass destruction criterion that does not distinguish between tensile and shear failure modes is of great significance for engineering practices.
3.1.2 Keyword evolution analysis
Figure 4 presents the timezone evolution view of keywords for research on structure rockburst. The timezone view comprises nodes with keywords in the same time zone. The keywords share the same time of their first appearance, and their chronological order follows from far to near. This visualization method effectively depicts the knowledge domain’s evolution process in the time dimension. This study selected a time series spanning 2003 to 2022, revealing that the time zone between 2009 and 2012 had fewer keywords clustered, suggesting less influential results. Conversely, from 2014 to 2019, more keywords clustered in the time zone, indicating a significant accumulation of influential keywords. Node concatenation between time zones signifies research heritage. Notably, research on structure rockburst exhibits good heritage and clear research direction.
As shown in Figure 4, in recent years, research on structure rockburst has been on the rise, due to the increasing significance of structure rockbursts in underground engineering projects as they continue to deepen. For instance, at the Jinping II Hydropower Station, a representative structure rockburst site, a significant proportion of rockbursts occur in areas featuring structural faces of diverse sizes, such as joints and hard structural faces. Moreover, rockbursts in the surrounding rock containing structural faces are often severe and destructive, and strong rockbursts may occur continuously in that cavern section (Feng et al., 2015; Cheng et al., 2019; Sun, 2021; Tu et al., 2021). Since 2014, numerous scholars have conducted extensive analyses of the mechanism of structure rockburst. For instance, Pan et al. (2021) derived a quantitative general function relationship between nodal density and energy density based on energy theory and damage mechanics in 2021. They discussed the impact of nodal density on the propensity of rockburst in elastic-brittle-plastic rock masses. In 2015, Yang et al. (2015) defined rockbursts from the perspective of dynamic crack expansion. They proposed a new rockburst energy discrimination index, the unit time relative energy release rate index, and applied it to simulate rockburst in the diversion tunnel of Jinping II Hydropower Station. The results obtained were consistent with the actual rockburst situation. Furthermore, Feng et al. (2019) noted that the area where time-lag rockbursts occur is usually rich in primary structural surfaces such as joints, fissures, and interlayers. The type of structural surfaces is dominated by hidden structural surfaces with small angles to the cavern axis.
In terms of intermediary centrality, the keywords “fracture” (centrality = 0.19), “model” (centrality = 0.15), “rock” (centrality = 0.14), “acoustic emission” (centrality = 0.13), and “numerical simulation” (centrality = 0.13) are the hottest terms in the keyword network. Table 1 presents an analysis of the 14 high-centrality hot keywords from 2014 to the present and their adoption times, which indicates that deeply buried tunnels or mines are the primary environments that foster structure rockburst. Additionally, rockburst prediction and the mechanism of rockburst occurrence remain hotspots for research (Ranjith et al., 2017; Dai et al., 2022). Through keyword burst detection, 16 strongly bursty keywords can be identified. CiteSpace considers this burst information as a means of measuring deeper changes, as shown in Figure 5. The figure demonstrates that the frequency of listed keywords has significantly changed from 2016 to the present period, reflecting the adoption of keywords such as “tunnel,” “earthquake,” and “II Hydropower Station.” Furthermore, the keyword burst detection is consistent with the keywords obtained by keyword clustering, as shown in Figure 6. The top 10 keyword clusters obtained in Figure 6 are labeled as # 0 to # 9.
3.2 Co-citation and cluster analysis
The co-citation analysis of authors and journals is a powerful method to identify influential authors and important sources of knowledge in a field. Author’s co-citation analysis focuses on extracting author information from the literature titles, while journal co-citation analysis extracts information from the references. Co-citation analysis enables the identification of highly cited authors and journals, and the construction of co-citation networks and clusters that can be used to answer questions about the connections between sources of knowledge in a field (Chen et al., 2013; Liang et al., 2020).
3.2.1 Author co-citation and cluster analysis
CiteSpace function and parameter area were utilized to set the relevant parameters. The time period selected for analysis was from 2003 to 2022, with a time slice of 1 and a broad value of Top50 for each time slice. The network linkage strength was calculated using the Cosine algorithm, and the network was not clipped. The results obtained from the run were the author’s co-citation network of structure rockburst studies, represented in Figure7A with clustering view Figure7B.
The co-citation network analysis of authors in the field of structure rockburst studies reveals that Aki K (centrality = 0.71), Barton N (centrality = 0.61), Ortlepp W (centrality = 0.20), Hoek E (centrality = 0.20), and Hasegawa H (centrality = 0.15) are the most central authors. These authors are considered as mediators who have greatly influenced the research on this topic. The higher centrality of these authors suggests that they have a greater impact and a higher probability of being cited by other authors in the field. Furthermore, the analysis indicates that cited authors with higher centrality also have a high probability of having a higher number of citations. Aki. (1979) pioneered the use of stochastic methods in seismic hazard assessment, focusing on peak ground acceleration and response spectra. This approach has been adopted by numerous scholars who have used seismic methods to study the mechanism of structure rockburst. Similarly, Hoek E is a prominent international expert in the study of rockburst and has proposed the use of the ratio of maximum principal stress to uniaxial compressive strength as an indicator of brittle damage for the classification of rockburst judging model (Cook et al., 1966; He et al., 2011; He M. C. et al., 2018). The high degree of centrality of author Hoek E can be attributed to the fact that the theoretical basis of structure rockburst is developed on the basis of rockburst theory. However, the current research on structure rockburst theory is still in its early stages, as the mechanism of interaction between joints and rock masses, crack expansion, energy evolution, and damage mechanism are very complex. Thus, there are several questions that need to be further explored to understand the mechanism of influence on the stability of rock masses containing structural faces.
The burst detection algorithm proposed by Kleinberg in 2002 is utilized in CiteSpace for detecting burst events or topics in time-series data. In CiteSpace, clusters containing more burst nodes are considered to be more active or emerging trends in the field, as measured by their Active Area or Emerging Trend values. In the present study, 9 author clusters were identified, labeled as #0 to #8, with their respective information presented in Table 2, ranked by LLR score. CiteSpace software provides valuable information on author clusters, including the largest cluster (#0) with 56 members and a high silhouette value of 0.781. The cluster is labeled LLR, indicating the use of multiple algorithms in the analysis. Semblat is the most relevant citation for this cluster, suggesting that the research topics of the members in this cluster may be related to the citation or influenced by Semblat’s work.
3.2.2 Institution co-citation analysis
The co-citation network of journals for studies on structural facies rockbursts was generated, as illustrated in Figure 8 (with unidentified journals excluded). The resulting journal co-citation networks indicate that the International Journal of Rock Mechanics and Mining Sciences (15 papers), Bulletin of The Seismological Society of America (10 papers), and Pure and Applied Geophysics (9 papers) are the most highly cited journals in international research on this topic. These three journals have the highest number of publications. By analyzing journal co-citation centrality, seven top-ranking journals with centrality greater than 0.1 are identified. Most of these journals are published in the UK, Poland, USA, and the Netherlands and are related to seismology, geophysics, or mining engineering.
CiteSpace offers insight into the sudden increase in the number of journals publishing research on structural facies between 2003 and 2022. Figure 9 shows that journals such as Comput Geotech and Tunn Undergr Sp Tech have increased their research output in recent years, highlighting the importance of research on structural facies.
3.2.3 Reference co-citation and cluster analysis
CiteSpace is a highly regarded tool in the field of reference analysis, and its co-citation analysis feature is considered the most prominent. The initial development and theoretical discussions surrounding CiteSpace were primarily focused on the implementation and utilization of the co-citation analysis feature. CiteSpace provides two distinct methods for culling co-citation data, including the MST minimum tree method and the pathfinder pathfinding network method. While the MST method is known for its simplicity and fast results, the pathfinder network algorithm is preferred due to its ability to reduce the number of connected lines while maintaining completeness and a unique solution. The pathfinding network algorithm selects significant relationships among neighboring networks based on the principle of triangular inequality, and the number of network nodes processed by the pathfinding network algorithm does not change, while the number of connecting lines is greatly reduced. This chapter utilizes the pathfinding network algorithm to conduct a literature co-citation analysis and identify the core literature on structure rockburst research. The analysis produces a literature co-citation network view (as shown in Figure 10) and a reference co-citation clustering view (as shown in Figure 11). Due to the large volume of reference available, only the five largest reference clusters are analyzed to obtain information on highly cited reference, as detailed in Table 2.
Furthermore, CiteSpace can also extract data on highly cited reference using the Degree Centrality metric, which is the most direct measure of node centrality in network analysis. A higher node degree signifies greater degree centrality and greater importance in the network. As illustrated in Table 3, CiteSpace analysis identified four highly cited papers with high degree centrality, which provide valuable insights for in-depth investigations into structure rockburst.
Table 3 shows that Dong’s 2016 publication titled “Discrimination of Mine Seismic Events and Blasts Using the Fisher Classifier, Naive Bayesian Classifier and Logistic Regression” is highly cited. The study examined the different characteristics of rockburst and earthquake events by comparing the probability density distribution of various parameters. Discriminators were constructed using the Fisher classifier, plain Bayesian classifier, and logistic regression. Three databases on Australian and Canadian mines were established to train, calibrate, and test the discriminant models. The study discusses and compares the classification performance and discriminative accuracy of the three statistical techniques. Likewise, Braunmiller’s research on the evolutionary characteristics of earthquake parameters in the Euro-Mediterranean area resulted in highly cited work. Moment magnitudes were used to provide a unified estimate of earthquake size for the region.
To identify significant reference related to structure rockburst, CiteSpace automatically generates reference reports within the software. These reports assist in obtaining specialized reference most relevant to the clustered clusters. Table 4 illustrates the reference reports generated by CiteSpace.
Upon further examination of the important literature mentioned above, it was discovered that Manouchehrian and Cai (2018) conducted research on a tunnel model with and without faults. The study simulated the rock damage process under both static and dynamic loads, and identified stable and unstable damage through the analysis of factors such as the velocity and released kinetic energy of rock damage, the damage area around the tunnel, and the deformation grid. In Li’s work (Li et al., 2021), it was found that the discontinuity of rock structures can result in structural damage and instability, leading to large displacement and rotation of rocks, as well as the release of large amounts of energy from structure rockbursts. Liu et al. (2022) employed microseismic monitoring technology to investigate the characteristics of structure rockbursts, and coupled it with reflective tomographic imaging technology to predict the location of in-situ source sources. The study analyzed the spatiotemporal distribution of microseismicity and provided quantitative explanations for the parameters of microseismic sources. However, microseismic monitoring techniques are difficult to apply in indoor tests, so many researchers prefer to use acoustic emission to record the process of rockburst occurrence. For instance, Liu X. Q. et al. (2019) conducted rockburst model tests on large-sized specimens using a self-developed rockburst model test device. The results indicated that the gradient loading process, the number of acoustic emission events, and the energy of the specimen at different moments of loading varied significantly, showing a great gradient change. The initial loading produced very small acoustic emission signals, while the occurrence of rockbursts led to an instantaneous and significant increase in these signals. The more energy gathered by the specimen, the more likely it was to experience an instantaneous and strong rockburst phenomenon.
4 Discussion and perspective
In this section, discussions and prospects will be conducted from the aspects of engineering practice analysis on structure rockbursts, indoor experiments, numerical simulations, and rockburst disaster prevention.
4.1 Engineering practice analysis
Through the statistical analysis and visualization of structure rockbursts, it has been inferred that structure rockburst research is more dependent on actual on-site engineering practices. Literature (Feng, 2017; Liu F. et al., 2019) has unanimously recognized that the existence of structural surfaces hinders the adjustment of rock stress in the surrounding rock after tunnel excavation, leading to the concentration of stress and the accumulation of energy in the rock mass between the excavation boundary and the structural surface, which ultimately triggers rockbursts. Based on the excavated rock mass characteristics revealed after the occurrence of rockbursts during the actual tunnel construction process, it has been found that a large portion of the regions where rockbursts occur contain different scales of structural surfaces, and rockbursts that occur in rock masses with structural surfaces are often large in scale and powerful in destruction, and may occur continuously in the tunnel (Xiao et al., 2016; Hu et al., 2020; Yu et al., 2020). Due to the complex mechanisms resulting from on-site geological conditions and initiating factors, the occurrence mechanism of rockbursts is very complex, and there is significant difference in the occurrence mechanism of different types of rockbursts.
Currently, no integrated monitoring and forecasting technology and system for rock bursts that combines rock mechanics parameters, microseism monitoring methods, and electromagnetic wave monitoring methods exists. In the future, it is necessary to establish and perfect an integrated monitoring and forecasting system for rockbursts based on experimental research and theoretical research, and conduct in-depth analysis of the occurrence mechanism of rockbursts and the dynamic process of rockbursts. Additionally, it is necessary to strengthen the research and development work on the dynamic analysis of rockbursts.
4.2 Analysis of indoor experiments
Through statistical analysis of literature (He M. C. et al., 2018; Wang et al., 2019; He et al., 2021) on structure rockbursts in indoor experiments, it can be inferred that the main method for studying the elastic-brittle behavior or post-peak stress-strain characteristics of rocks is through single-axial compression testing, biaxial loading testing, regular three-axis testing, or true three-axis testing. However, the inclination angle of structural surfaces has a significant impact on the stress distribution, crack initiation and propagation location and path, and failure mode in the surrounding rock mass (Jia and Zhu, 2012; Li et al., 2012).
Currently, most research focuses on the role of small-scale structures such as joints and fractures in causing rockbursts during tunnel excavation, with little research on the role of large-scale structures such as faults and shear bands in causing rockbursts during tunnel excavation. Through a summary, it can be found that the structure rockburst indoor experiments rarely consider the role of structural surfaces under different stress gradients, which may be a research hotspot in the future. On the other hand, it is necessary to conduct research on low-hardness, high-brittle materials to develop suitable materials for physical model testing of structure rockbursts, and analyze the destruction process and destruction rules of rockbursts.
4.3 Numerical simulation analysis
Through statistical analysis of literature, it has been found that significant research has been conducted on the mechanism of rockbursts using numerical simulation methods (Jia and Tang, 2008; Zhang et al., 2013; Manouchehrian and Cai, 2017). The research mainly focuses on the simulation analysis of macroscopic and microscopic damage and energy change patterns of rock bursts under the action of intact surrounding rocks or single joints. However, the study of the dynamic damage process and failure mechanism of rockbursts in the presence of rock fractures is still insufficient, and numerical simulation needs to be conducted to address the effects of complex structural surface interactions (Abdul et al., 2018; Guo et al., 2022). Additionally, structure rockburst is a type of rockburst that is primarily determined by high stresses and structural surfaces, and the destruction process of rockbursts is complex. Currently, there is limited research on the destruction process of structure rockbursts in three-dimensional conditions, and researchers often overlook the impact of structural surfaces and incremental excavation unloading on the destruction of rock masses at different tunnel faces.
4.4 Analysis of rockburst prediction and control
The current rock burst mechanism is complex, and the prediction problem is also extremely complex. So far, there is no complete set of mature prediction methods used. Multi index comprehensive criteria can be used to comprehensively predict rock bursts for structure rockbursts. At the same time, we should pay attention to the main control factors selected for prediction, eliminate confounding and improve the prediction accuracy. At present, the warning of structure rockburst mainly relies on microseismic technology and acoustic emission technology, but research mainly focuses on compressive shear failure mode, and there is still relatively little research on tensile and tensile shear failure. Therefore, it is necessary to improve the level of monitoring and early warning, and explore universal indicators with strong accuracy.
Upon reviewing the literature, it has been suggested that in order to prevent and control structure rockbursts, it is essential to summarize and compare the applicable parameters for different rock section grades’ initial support. Furthermore, a detailed comparison and summary of the mining methods, core lengths, and corresponding waiting times for different rock section grades should also be conducted. To date, the rockburst criteria of tunnels in the same area, rock type, and burial depth vary greatly, highlighting the need to collect and analyze relevant data to establish a detailed prediction database with abundant data. As such, it is believed that the development of an effective prediction model based on advanced analytics and machine learning techniques will become an essential step in solving this challenging issue.
5 Conclusion
Through in-depth mining of structure rockburst literature and the improvement of the structure rockburst research field, the visualization analysis of CiteSpace software was used to obtain the current situation and research hotspots of structure rockburst research from 2003 to 2022. The following summary and analysis were conducted:
(1) The structure rockburst belongs to the category of strain rockburst and fault-slide rockburst. Buckling, shear, and fault slide destruction are common forms of structure rockburst. The study of structure rockburst is highly dependent on field engineering observations. The existence of the structural surface hinders the adjustment of rock stress in the surrounding rock after tunnel excavation, leading to the concentration of rock stress and the accumulation of energy between the tunnel edge and the structural surface, ultimately causing rockburst disasters.
(2) Under laboratory testing conditions, it is possible to simulate the intense brittle destruction phenomena shown during actual rock explosions by designing appropriate loading and unloading testing methods. This has significant implications for understanding the mechanisms, processes, and characteristics of rockburst. Through laboratory testing, it can be discovered that structural surfaces are not a necessary but rather a sufficient condition for rockbursts to occur. In addition to stress and rock conditions, the mechanical properties and geometric characteristics of structural surfaces also need to meet certain requirements for structure rockburst to occur. Structural surfaces with a tendency for slippage without filling, or with high-strength fillers, and structural surfaces with vertical orientations that are prone to exacerbate rock fragmentation, buckling, and ejection are key focuses for the prevention and control of rockburst disasters.
(3) The issue of rockburst is of significant importance. Through numerical simulation of different spatial distributions of structural surfaces, it is believed that the existence state of structural surfaces is a crucial factor affecting the ignition and development of rockbursts. The impact mechanism of structural surfaces located at different positions and orientations on rockbursts is diverse. In particular, inclined structural surfaces have a high probability of experiencing either shear or shear-tensile failure, which can initiate slide type or shear fracture type rockbursts.
(4) Through visual analysis of structure rockbursts, it can be inferred that structure rockbursts are primarily studied through on-site surveying and on-site monitoring to investigate the process of rockbursts. Meterological monitoring has become a reliable technique for predicting structure rockbursts. However, research attention is primarily focused on the compression and tension failure mode, while research on the tension and compression failure mode remains limited. Therefore, it is essential to enhance the level of monitoring and warning, and explore universally applicable indicators with high accuracy.
(5) At present, the warning of structure rockburst mainly relies on microseismic technology and acoustic emission technology, but research mainly focuses on compressive shear failure mode, and there is still relatively little research on tensile and tensile shear failure. Therefore, it is necessary to improve the level of monitoring and early warning, and explore universal indicators with strong accuracy. In the future, image processing technology and audio processing technology may be used to study structure rockbursts and improve the accuracy of rockburst prediction.
(6) At present, there is a lack of research on structure rockbursts. With the continuous development of underground space, the frequency of structure rockburst disasters will increase. A detailed visual analysis has shown that the current state of research on structure rockburst is consistent with visual analysis results. However, research on structure rockburst is still at the qualitative stage, and the mechanism and mechanism of the role of structural surfaces in the process of structure rockburst development require further exploration.
Author contributions
YZ: Data curation, Formal Analysis, Methodology, Software, Validation, Visualization, Writing–original draft. YX: Methodology, Supervision, Conceptualization, Validation, Investigation, Funding acquisition. JH: Methodology, Project administration, Resources, Writing–review and editing.
Funding
This work is financially supported by Natural Science Foundation of China (Grant No. 42077228).
Acknowledgments
We gratefully acknowledge the support of Wuhan University of Technology, Wuhan, China.
Conflict of interest
The authors declare that the research was conducted in the absence of any commercial or financial relationships that could be construed as a potential conflict of interest.
Publisher’s note
All claims expressed in this article are solely those of the authors and do not necessarily represent those of their affiliated organizations, or those of the publisher, the editors and the reviewers. Any product that may be evaluated in this article, or claim that may be made by its manufacturer, is not guaranteed or endorsed by the publisher.
References
Abdul, M., Hafeezur, R., and Muhammad, Z., (2018). Impact of shear zone on rockburst in the deep neelum-jehlum hydropower tunnel: A numerical modeling approach. Energies 11 (8), 193. doi:10.3390/en11081935
Aki, K. (1979). Characterization of barriers on an earthquake fault. J. Geophys. Res. 84 (B11), 6140–6148. doi:10.1029/jb084ib11p06140
Arablouei, A., and Kodur, V. (2014). A fracture mechanics-based approach for quantifying delamination of spray-applied fire-resistive insulation from steel moment-resisting frame subjected to seismic loading. Eng. Fract. Mech. 121–122, 67–86. doi:10.1016/j.engfracmech.2014.03.003
Barton, N., Wang, C. S., and Yong, R. (2023). Advances in joint roughness coefficient (JRC) and its engineering applications. J. Rock Mech. Geotechnical Eng., doi:10.1016/j.jrmge.2023.02.002
Braunmiller, J., Kradolfer, U., Baer, M., and Giardini, D. (2002). Regional moment tensor determination in the European-Mediterranean area-initial results. Tectonophysics 356 (1), 5–22. doi:10.1016/s0040-1951(02)00374-8
Castro, L. M., Gómez, H. W., and Valenzuela, M. (2012). Epsilon half-normal model: properties and inference. Comput. Statistics Data Analysis 56 (12), 4338–4347. doi:10.1016/j.csda.2012.03.020
Chen, C. M., Chen, Y., Horowitz, M., Hou, H., Liu, Z., and Pellegrino, D. (2009). Towards an explanatory and computational theory of scientific discovery. J. Inf. 3 (3), 191–209. doi:10.1016/j.joi.2009.03.004
Chen, C. M. (2006). CiteSpace II: detecting and visualizing emerging trends and transient patterns in scientific literature. J. Am. Soc. Inf. Sci. Technol. 57 (3), 359–377. doi:10.1002/asi.20317
Chen, C. M. (2013). Hindsight, insight, and foresight: A multi-level structural variation approach to the study of a scientific field. Technol. Analysis Strategic Manag. 25 (6), 619–640. doi:10.1080/09537325.2013.801949
Cheng, H. Z., Chen, J., and Chen, R. P., (2019). Reliability study on shield tunnel face using a random limit analysis method in multilayered soils. Tunn. Undergr. Space Technol. 84, 353–363. doi:10.1016/j.tust.2018.11.038
Cook, N., Hoek, E., and Pretorius, J., (1966). Rock mechanics applied to the study of rockbursts. South Afr. Inst. Min. Metallurgy 66 (10), 435–528.
Dai, L., Pan, Y., Zhang, C., Wang, A., Canbulat, I., Shi, T., et al. (2022). New criterion of critical mining stress index for risk evaluation of roadway rockburst. Rock Mech. Rock Eng. 55 (8), 4783–4799. doi:10.1007/s00603-022-02888-7
Diederichs, M. S. (2018). Early assessment of dynamic rupture hazard for rockburst risk management in deep tunnel projects. J. South Afr. Inst. Min. Metallurgy 118 (3), 193–204. doi:10.17159/2411-9717/2018/v118n3a1
Dong, L., Wesseloo, J., Potvin, Y., and Li, X. B. (2016b). Discriminant models of blasts and seismic events in mine seismology. Int. J. Rock Mech. Min. Sci. 86, 282–291. doi:10.1016/j.ijrmms.2016.04.021
Dong, L., Wesseloo, J., Potvin, Y., and Li, X. (2016a). Discrimination of mine seismic events and blasts using the Fisher classifier, naive bayesian classifier and logistic regression. Rock Mech. Rock Eng. 49, 183–211. doi:10.1007/s00603-015-0733-y
Fang, K., Zhang, J., Tang, H., Hu, X., Yuan, H., Wang, X., et al. (2023). A quick and low-cost smartphone photogrammetry method for obtaining 3D particle size and shape. Eng. Geol. 322, 107170. doi:10.1016/j.enggeo.2023.107170
Feng, F., Li, X., and Jamal, R., (2018a). Modeling hard rock failure induced by structural planes around deep circular tunnels. Eng. Fract. Mech. 205, 152–174. doi:10.1016/j.engfracmech.2018.10.010
Feng, G. L., Feng, X. T., Chen, B. R., and Xiao, Y. X. (2015). Microseismic sequences associated with rockbursts in the tunnels of the Jinping II Hydropower Station. Int. J. Rock Mech. Min. Sci. 80, 89–100. doi:10.1016/j.ijrmms.2015.06.011
Feng, G. L., Feng, X. T., Chen, B. R., Xiao, Y. X., and Zhao, Z. N. (2018b). Effects of structural planes on the microseismicity associated with rockburst development processes in deep tunnels of the Jinping-II Hydropower Station, China. Tunn. Undergr. Space Technol. 84, 273–280. doi:10.1016/j.tust.2018.11.008
Feng, X. T., Chen, B. R., and Ming, H. J., (2012). Modeling hard rock failure induced by structural planes around deep circular tunnels. Rock Mech. Rock Eng. 31 (3), 433–444. doi:10.1016/j.engfracmech.2018.10.010
Feng, X. T., Chen, B. R., and Zhang, C. Q., (2013). Mechanism, early warning and dynamic regulation of rock explosion incubation process. Beijing, China: Science Press.
Feng, X. T. (2017). Rockburst: Mechanism, monitoring, warning and mitigation. Amsterdam, Netherlands: Elsevier Health Sciences Division, 551–558.
Feng, X. T., Xiao, Y. X., and Feng, G. L., (2019). Study of rockburst incubation process. J. Rock Mech. Eng. 38 (4), 649–673. doi:10.13722/j.cnki.jrme.2019.0103
Ghasemi, E., Gholizadeh, H., and Adoko, A. C. (2019). Evaluation of rockburst occurrence and intensity in underground structures using decision tree approach. Eng. Comput. 36 (1), 213–225. doi:10.1007/s00366-018-00695-9
Guo, H., Chen, L., Zhu, J., Sun, Q., and Xiao, Y. (2022). Application of borehole camera technology in the identification of an instantaneous strain-structural-plane slip rockburst. Bull. Eng. Geol. Environ. 81, 186. doi:10.1007/s10064-022-02658-3
He, M. C., Cheng, T., Qiao, Y. F., and Li, H. (2022). A review of rockburst: experiments, theories, and simulations. J. Rock Mech. Geotechnical Eng. 15, 1312–1353. doi:10.1016/j.jrmge.2022.07.014
He, M. C., Huang, B., Zhu, C., and Chen, Y. (2018a). Energy dissipation-based method for fatigue life prediction of rock salt. Rock Mech. Rock Eng. 51 (5), 1447–1455. doi:10.1007/s00603-018-1402-8
He, M. C., Li, J. Y., and Ren, F. Q., (2021). Experimental study on the ejection velocity of unidirectional double-sided unloading rockbursts from sandstones with different laminar dip angles. J. Rock Mech. Eng. 40 (3), 433–447. doi:10.13722/j.cnki.jrme.2020.0753
He, M. C., Nie, W., Zhao, Z. Y., and Cheng, C. (2011). Micro and macro fractures of coarse granite under true-triaxial unloading conditions. Min. Sci. Technol. (China) 21 (3), 389–394. doi:10.1016/j.mstc.2011.05.016
He, M. C., Ren, F. Q., Cheng, C., and Faramarzi, L. (2018b). Mechanism of strain burst by laboratory and numerical analysis. Shock Vib. 2018, 1–15. doi:10.1155/2018/8940798
Hou, P., Gao, F., and Zhang, Z. Z., (2016). Method for evaluating rock brittleness based on acoustic emission and energy evolution laws. J. China Univ. Min. Technol. 45 (4), 702–708. doi:10.13247/j.cnki.jcumt.000525
Hu, L., Feng, X. T., Xiao, Y. X., Wang, R., Feng, G. L., Yao, Z. B., et al. (2020). Effects of structural planes on rockburst position with respect to tunnel cross-sections: A case study involving a railway tunnel in China. Bull. Eng. Geol. Environ. 79, 1061–1081. doi:10.1007/s10064-019-01593-0
Jia, P., and Tang, C. (2008). Numerical study on failure mechanism of tunnel in jointed rock mass. Tunn. Undergr. Space Technol. 23 (5), 500–507. doi:10.1016/j.tust.2007.09.001
Jia, P., and Zhu, W. (2012). Dynamic-static coupling analysis on rockburst mechanism injointed rock mass. J. Central South Univ. 19 (11), 3285–3290. doi:10.1007/s11771-012-1405-7
Jiang, L., Pu, W., Zhang, P., Zheng, P., and Xu, B. (2017). Numerical analysis of the effects induced by normal faults and dip angles on rock bursts. Comptes Rendus Mec. 345 (10), 690–705. doi:10.1016/j.crme.2017.06.009
Keneti, A., and Sainsbury, B. A. (2018). Review of published rockburst events and their contributing factors. Eng. Geol. 246 (1), 361–373. doi:10.1016/j.enggeo.2018.10.005
Li, D., Wong, L. N. Y., Liu, G., and Zhang, X. (2012). Influence of water content and anisotropy on the strength and deformability of low porosity meta-sedimentary rocks under triaxial compression. Eng. Geol. 126, 46–66. doi:10.1016/j.enggeo.2011.12.009
Li, G., Cheng, X., Hu, L., and Tang, C. (2021). The material-structure duality of rock mass: insight from numerical modeling. Int. J. Rock Mech. Min. Sci. 144 (1), 104821. doi:10.1016/j.ijrmms.2021.104821
Li, X., Gong, F., Ming, T., Dong, L., Du, K., Ma, C., et al. (2017). Failure mechanism and coupled static-dynamic loading theory in deep hard rock mining: A review. J. Rock Mech. Geotechnical Eng. 9 (4), 767–782. doi:10.1016/j.jrmge.2017.04.004
Li, Z., Tao, M., Du, K., Cao, W., and Wu, C. (2020). Dynamic stress state around shallow-buried cavity under transient P wave loads in different conditions. Tunn. Undergr. Space Technol. 97, 103228–103312. doi:10.1016/j.tust.2019.103228
Liang, W., Dai, B., Zhao, G., and Wu, H. (2020). A scientometric review on rockburst in hard rock: two decades of review from 2000 to 2019. Geofluids 2020 (12), 1–17. doi:10.1155/2020/8763283
Linzer, L. M. (2005). A relative moment tensor inversion technique applied to seismicity induced by mining. Rock Mech. Rock Eng. 38 (2), 81–104. doi:10.1007/s00603-004-0041-4
Liu, F., Tang, C. A., Ma, T. H., and Tang, L. (2019a). Characterizing rockbursts along a structural plane in a tunnel of the Hanjiang-to-Weihe river diversion project by microseismic monitoring. Rock Mech. Rock Eng. 52 (6), 1835–1856. doi:10.1007/s00603-018-1649-0
Liu, Q. S., Wu, J., Zhang, X. P., Azhar, M. U., Yong, Z., and Fudong, C. (2022). Numerical simulation method for the process of rockburst. Eng. Geol. 306 (5), 106760. doi:10.1016/j.enggeo.2022.106760
Liu, Q. S., Wu, J., Zhang, X. P., Tang, L. X., Bi, C., Li, W. W., et al. (2020). Microseismic monitoring to characterize structure-type rockbursts: A case study of a TBM-excavated tunnel. Rock Mech. Rock Eng. 53 (16), 2995–3013. doi:10.1007/s00603-020-02111-5
Liu, X. Q., Xia, Y. Y., and Lin, M. Q., (2019b). Experimental study of rockburst under true-triaxial gradient loading conditions. Geomechanics Eng. 18 (5), 481–492. doi:10.12989/gae.2019.18.5.481
Manouchehrian, A., and Cai, M. (2017). Analysis of rockburst in tunnels subjected to static and dynamic loads. J. Rock Mech. Geotechnical Eng. 9 (6), 1031–1040. doi:10.1016/j.jrmge.2017.07.001
Manouchehrian, A., and Cai, M. (2018). Numerical modeling of rockburst near fault zones in deep tunnels. Tunn. Undergr. Space Technol. 80, 164–180. doi:10.1016/j.tust.2018.06.015
Meng, F., Zhou, H., Wang, Z., Zhang, L., and Kong, L., (2017). Experimental study of factors affecting fault slip rockbursts in deeply buried hard rock tunnels. Bull. Eng. Geol. Environ. 76, 1167–1182. doi:10.1007/s10064-016-0926-y
Pan, J., Ren, F., and Cai, M. (2021). Effect of joint density on rockburst proneness of the elastic-brittle-plastic rock mass. Shock Vib. 2021 (17), 1–9. doi:10.1155/2021/5574325
Qiu, S., Feng, X., Zhang, C., and Xiang, T. (2014). Estimation of rockburst wall-rock velocity invoked by slab flexure sources in deep tunnels. Can. Geotechnical J. 51 (5), 520–539. doi:10.1139/cgj-2013-0337
Ranjith, P. G., Zhao, J., Ju, M., De Silva, R. V., Rathnaweera, T. D., and Bandara, A. K. (2017). Opportunities and challenges in deep mining: A brief review. Engineering 3 (4), 546–551. doi:10.1016/j.eng.2017.04.024
Sun, L. J. (2021). Analytical solution for lined circular tunnels in deep viscoelastic burgers rock considering the longitudinal discontinuous excavation and sequential installation of liners. J. Eng. Mech. 147 (4), 04021009. doi:10.1061/(ASCE)EM.1943-7889.0001912
Tajdus, A., Cala, J. M., and Tajdus, K. (2019). Seismicity and rock burst hazard assessment in fault zones: A case study. Archives Min. Sci. 63 (3), 747–765. doi:10.24425/123695
Tang, C., Tham, L., Lee, P., Yang, T., and Li, L. (2002). Coupled analysis of flow, stress and damage (FSD) in rock failure. Int. J. Rock Mech. Min. Sci. 39 (4), 477–489. doi:10.1016/s1365-1609(02)00023-0
Tu, H., Zhou, H., and Gao, Y., (2021). Probability analysis of deep tunnels based on Monte Carlo simulation: case study of diversion tunnels at jinping-hydropower station, southwest China. Int. J. Geomechanics 12, 21. doi:10.1061/(ASCE)GM.1943-5622.0002146
Wang, C. S., Yong, R., Luo, Z. Y., Du, S., Karakus, M., and Huang, C. (2023). A novel method for determining the three-dimensional roughness of rock joints based on profile slices. Rock Mech. Rock Eng. 56, 4303–4327. doi:10.1007/s00603-023-03274-7
Wang, J., Chen, G., Xiao, Y., Li, S., and Qiao, Z. (2021). Effect of structural planes on rockburst distribution: case study of a deep tunnel in southwest China. Eng. Geol. 292 (4), 106250. doi:10.1016/j.enggeo.2021.106250
Wang, Y., He, M. C., Ren, F. Q., Zhu, C., and Faramarzi, L. (2019). Source analysis of acoustic emissions during granite strain burst. Geomatics, Nat. Hazards Risk 10 (1), 1542–1562. doi:10.1080/19475705.2019.1593888
Xiao, Y. X., Feng, X. T., Li, S. J., Feng, G., and Yu, Y. (2016). Rock mass failure mechanisms during the evolution process of rockbursts in tunnels. Int. J. Rock Mech. Min. Sci. 83, 174–181. doi:10.1016/j.ijrmms.2016.01.008
Xu, N. W., Li, T. B., Dai, F., Zhang, R., Tang, C. A., and Tang, L. X. (2016). Microseismic monitoring of strainburst activities in deep tunnels at the jinping II hydropower station, China. Rock Mech. Rock Eng. 49 (3), 981–1000. doi:10.1007/s00603-015-0784-0
Yang, F. J., Zhou, H., and Lu, J. J., (2015). Rockburst process of energy discriminatory indicators. J. Rock Mech. Eng. 34 (S1), 2706–2714. doi:10.13722/j.cnki.jrme.2013.1862
Yang, F. J., Zhou, H., Xiao, B., Tang, L. X., Bi, C., Li, W. W., et al. (2020). Microseismic monitoring to characterize structure-type rockbursts: A case study of a TBM-excavated tunnel. Rock Mech. Rock Eng. 53 (16), 2995–3013. doi:10.1007/s00603-020-02111-5
Yu, Y., Feng, X. T., Xu, C. J., Chen, B. r., Xiao, Y. X., and Feng, G. L. (2020). Spatial fractal structure of microseismic events for different types of rockburst in deeply buried tunnels. Int. J. Geomechanics 20 (4). doi:10.1061/(asce)gm.1943-5622.0001631
Zhang, C. Q., Feng, X. T., Zhou, H., Qiu, S. L., and Wu, W. P. (2012). Case histories of four extremely intense rockbursts in deep tunnels. Rock Mech. Rock Eng. 45 (3), 275–288. doi:10.1007/s00603-011-0218-6
Zhang, C. Q., Feng, X. T., Zhou, H., Qiu, S., and Wu, W. (2013). Rockmass damage development following two extremely intense rockbursts in deep tunnels at Jinping II Hydropower Station, southwestern China. Bull. Eng. Geol. Environ. 72 (2), 237–247. doi:10.1007/s10064-013-0470-y
Zhang, W., Feng, X. T., Yao, Z. B., Hu, L., and Xiao, Y. X., (2022). Development and occurrence mechanisms of fault-slip rockburst in a deep tunnel excavated by drilling and blasting: A case study. Rock Mech. Rock Eng. 55 (9), 5599–5618. doi:10.1007/s00603-022-02927-3
Zhao, Y. S. (2021). Some review of the development of rock mechanics and some unanswered centennial questions. J. Rock Mech. Eng. 40 (7), 1297–1336. doi:10.13722/j.cnki.jrme.2021.0617
Zhou, H., Meng, F. Z., and Zhang, C. Q., (2015b). Analysis of structural surface action mechanism of rock burst in deep buried hard rock tunnels. J. Rock Mech. Eng. 34 (4), 720–727.
Zhou, H., Meng, F. Z., and Zhang, C. Q., (2015c). Structural surface shear damage characteristics and its application to the study of slip-type rockburst. J. Rock Mech. Eng. 34 (9), 21. doi:10.13722/j.cnki.jrme.2014.0337
Zhou, H., Meng, F. Z., Zhang, C. Q., Hu, D., Yang, F., and Lu, J. (2015a). Analysis of rockburst mechanisms induced by structural planes in deep tunnels. Bull. Eng. Geol. Environ. 74 (4), 1435–1451. doi:10.1007/s10064-014-0696-3
Keywords: scientometric analysis, Citespace, structure rockburst, rockburst prediction, disaster
Citation: Zhang Y, Xia Y and Huang J (2023) A scientometric review of structure rockburst research: current trends and future directions. Front. Earth Sci. 11:1254041. doi: 10.3389/feart.2023.1254041
Received: 06 July 2023; Accepted: 22 August 2023;
Published: 31 August 2023.
Edited by:
Yi Xue, Xi’an University of Technology, ChinaReviewed by:
Kun Fang, China University of Geosciences Wuhan, ChinaLei Gao, Hohai University, China
Changshuo Wang, Ningbo University, China
Copyright © 2023 Zhang, Xia and Huang. This is an open-access article distributed under the terms of the Creative Commons Attribution License (CC BY). The use, distribution or reproduction in other forums is permitted, provided the original author(s) and the copyright owner(s) are credited and that the original publication in this journal is cited, in accordance with accepted academic practice. No use, distribution or reproduction is permitted which does not comply with these terms.
*Correspondence: Yuanhang Zhang, zhangyuanhang@whut.edu.cn; Yuanyou Xia, xiayy1965@whut.edu.cn