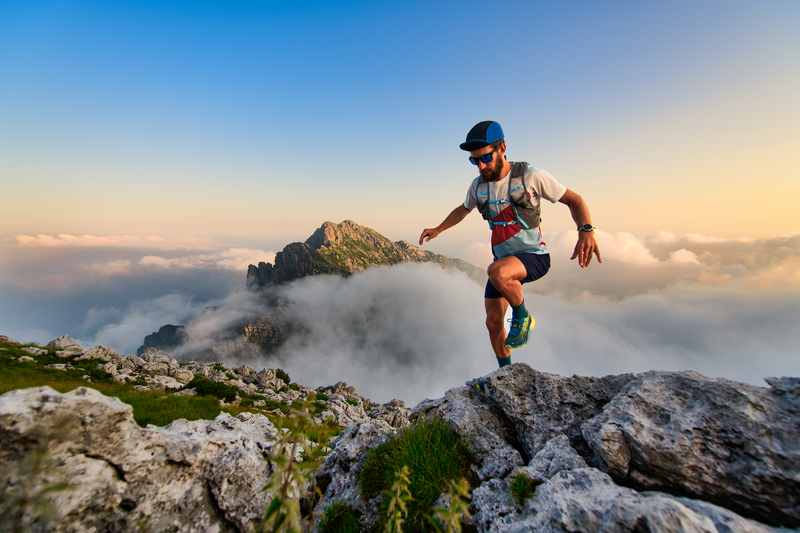
95% of researchers rate our articles as excellent or good
Learn more about the work of our research integrity team to safeguard the quality of each article we publish.
Find out more
REVIEW article
Front. Earth Sci. , 11 July 2023
Sec. Biogeoscience
Volume 11 - 2023 | https://doi.org/10.3389/feart.2023.1211574
This is a systematic literature review of quantitative sustainability assessments, focusing on life cycle sustainability assessment (LCSA), of bio-mediated and bio-inspired ground improvement technologies applied to geologic hazard mitigation. The aims of the systematic review are to 1) compare the sustainability of various ground improvement techniques and, 2) to evaluate the rigor and consistency of sustainability assessment methods applied to these techniques. The literature review considers studies identified through keyword searches of bibliographic databases. After selection criteria were applied to ensure identified articles were within scope, a total of 8 articles were found which assessed bio-mediated and bio-inspired ground improvement technologies. The technologies represented in the literature include enzyme induced carbonate precipitation (EICP), microbially induced carbonate precipitation (MICP), and microbially induced desaturation and carbonate precipitation (MIDP). While sustainability is typically conceived to include environmental, economic and social impacts, most studies examined only life cycle environmental impacts, three included life cycle cost accounting, and none included social impacts. Analysis of the studies’ system boundaries show inconsistencies across studies, making comparison of results inaccurate. The most common environmental impact categories included in the identified studies are global warming and eutrophication. Raw materials production and field emissions from the biogeochemical reactions that drive the technologies are the largest contributors to these impacts. Based on the review, it is clear that a set of LCSA guidelines is needed to produce high-quality LCSAs that can be used in comparative assessments and to confidently identify processes where the impacts of bio-mediated and bioinspired technologies can be reduced.
Resilience is crucial when planning and designing infrastructure projects that holistically reduce geologic disaster risk, including effects exacerbated by climate change (Risken et al., 2015; Chang et al., 2019). Mitigating geologic hazards in our built environment (e.g., earthquake-induced liquefaction, landslides, coastal erosion, and subsidence) via geotechnical engineering techniques can improve infrastructure resiliency. Ground improvement techniques are one category of hazard mitigation technologies that have seen increased adoption. Ground improvement methods such as jet grouting (subsurface application of pressurized cement grout) and vibro compaction (vibrations to physically densify soil) can provide earthquake-induced soil liquefaction mitigation and landslide mitigation through soil reinforcement and densification mechanisms, respectively (Mitchell, 2012; Raymond et al., 2021a). However, hazard mitigation techniques impact social, environmental, and economic dimensions. Traditional ground improvement techniques can be resource-intensive and often lead to high life cycle greenhouse gas (GHG) emissions due to their high rates of cement use and fuel consumption (Jefferis, 2008; Basu et al., 2015; Raymond et al., 2021a). However, ground improvement plays a significant role in improving the resilience of critical lifelines such as electricity and transportation systems which are crucial in providing support to communities after a geologic event and throughout potential evacuation procedures (Andrus and Chung, 1995).
The need to continue investing in civil infrastructure coupled with the need to mitigate GHG emissions and other environmental impacts has led to development of a number of sustainability rating systems and benchmarks for use across civil design, but to date, they lack guidance for geotechnical engineering applications such as geologic hazard mitigation (Kendall et al., 2018; Raymond et al., 2021a). In addition, these rating systems and benchmarks do not require evaluation of projects from a life-cycle standpoint. Previous research has concluded that life cycle-based methods can improve both the sustainability and technical feasibility of geotechnical projects (Shillaber et al., 2016; Kendall et al., 2018; Lee and Basu, 2018). Life cycle sustainability assessment (LCSA) is one such method. It evaluates the social, environmental and economic impacts of a system or project over its entire supply chain and life cycle and can be used to guide the development of more sustainable geotechnical solutions (Kendall et al., 2018; Raymond et al., 2021a).
LCSA builds on existing life cycle assessment (LCA) and life cycle cost assessment (LCCA) frameworks—two methods with long histories and broad acceptance in civil engineering (Harvey et al., 2011; Cabeza et al., 2014; Parrish and Chester, 2014). Traditional LCSAs of civil infrastructure projects typically assess only environmental and economic impacts, excluding social impacts such as human rights, working conditions, or impacts on cultural heritage (Jørgensen et al., 2007; United Nations Environment Programme, 2020). However, a complete LCSA should include a social life cycle assessment (S-LCA) to understand the sometimes competing social, environmental, and economic impacts of a project. Throughout this article, we use the term LCSA to describe any study which includes at least LCA, and may also include LCCA, but recognize that a true LCSA must include all three (LCA, LCCA, and S-LCA).
LCSA has previously been applied to evaluate geotechnical engineering projects (e.g., Raymond et al., 2021a), but it remains in its nascent stages for the geotechnical community. Previous reviews of geotechnical LCSAs have shown that the methods used to perform geotechnical LCSA vary, producing data that are not harmonized and that cannot be used in project or technology comparisons across studies (Kendall et al., 2018; Song et al., 2020; Samuelsson et al., 2021).
Biogeotechnics has emerged as a promising solution to improve the sustainability of geotechnical infrastructure for geologic hazard mitigation, by reducing the environmental impacts and cost of ground improvement (Dejong et al., 2014; Wang et al., 2017; Sharma et al., 2021). Biogeotechnics are typically classified in two ways, as bio-mediated technologies and bio-inspired technologies. Bio-mediated technologies rely on microbial activity to achieve geologic hazard mitigation while bio-inspired technologies mimic natural systems to reduce geologic risks (Khodadadi et al., 2017). Bio-mediated and bio-inspired technologies often replace cement-intensive traditional grouting methods, which have high costs associated with them (Jefferis, 2008; Basu et al., 2015; Raymond et al., 2021a).
Biogrouting, or biocementation, is a group of methods currently under development for ground improvement applications, such as foundational support and earthquake-induced liquefaction mitigation (DeJong et al., 2010; Khodadadi et al., 2017). Biogrouting techniques include enzyme-induced carbonate precipitation (EICP), microbially induced carbonate precipitation (MICP), and microbially induced desaturation and precipitation (MID and MIDP) (van Paassen et al., 2010a; Almajed et al., 2018; Moug et al., 2022). MICP and MIDP rely on microbial activity to produce carbonate precipitates, whereas EICP relies on enzymatic activity to produce these precipitates (Khodadadi et al., 2017). Carbonate precipitation improves the strength and dilatancy of soil (Cui et al., 2021). MIDP also involves another mechanism, microbially induced desaturation, through which microbial activity produces nitrogen gas desaturating the soil thereby providing liquefaction mitigation (Dejong et al., 2014; O’Donnell et al., 2017).
While a key driving factor behind the development of bio-mediated and bio-inspired geotechnical technologies has been their assumed environmental benefits, such as reduced cement consumption, these technologies carry their own environmental impacts (Raymond et al., 2021b). As these technologies develop, quantitative sustainability assessments can be used to verify their performance regarding social, environmental, and economic impacts and to guide sustainable bio-mediated and bio-inspired technology development (Raymond et al., 2020). At the same time, traditional geologic hazard mitigation technologies must also be assessed so that fair comparative assessments can be made.
To understand the current state of practice for LCSA applied to bio-mediated and bioinspired ground improvement technologies, a systematic literature review of quantified sustainability assessments, such as LCSA, of EICP, MICP, and MIDP technologies, is undertaken. In addition to understanding the current state of practice, the literature review:
Investigates the frequency of bio-mediated and bio-inspired LCSA studies within broader ground improvement literature
Summarizes the sustainability potential of bio-mediated and bio-inspired technologies at their current stage of development
Recommends future directions for bio-mediated and bio-inspired technology development to achieve sustainability goals
LCA can be used to determine the environmental and human health impacts of any product or system, over its entire life cycle. Examples of impact categories assessed include global warming, eutrophication, acidification, and human health impacts. LCA is also used to quantify the life cycle water consumption and cumulative energy demand of a product or system. LCAs are typically completed with a “cradle-to-grave” scope, or system boundary, where each of the following life cycle stages is modeled: extraction and processing of raw materials, manufacturing, transportation of materials and equipment, construction, operation and maintenance, and end-of-life. For a geotechnical engineering project, the whole life cycle includes these stages over the entire project life including site investigation, construction, potential remedial measures in the case of a geologic event, and decommissioning.
The International Organization for Standardization (ISO) 14040 and 14044 standards are the most commonly cited standard for LCA (ISO, 2006a; ISO, 2006b). The ISO standard is conceived in four phases. The goal and scope phase includes determining the purpose of the study, the system boundary of analysis, and the application and its function and functional unit, among other criteria. In the life cycle inventory (LCI) analysis phase, all environmental inflows, including energy and material inputs, and outflows, including water and air emissions as well as solid waste outputs, across the modeled system are quantified and aggregated. A life cycle impact assessment (LCIA) is then performed through which characterisation factors are applied to relevant flows to estimate the environmental and human health impacts of the system. Conclusions from the LCI analysis and LCIA are then drawn during the interpretation phase and possible recommendations for technology use or development, or future LCAs are provided.
LCCA accounts for the capital, operation and maintenance, potential replacement, and end-of-life phases of a product or system and can also include external costs associated with the system. In an LCSA, these costs are estimated for the same goal and scope outlined in the LCA. In an LCCA, all future costs are discounted to account for the change in monetary value over time. While less common, LCCA can also be used to estimate the external costs of a system such as the environmental or social cost of environmental emissions. For example, the social cost of carbon (SCC) has been applied to geotechnical projects where the economic damages that would result from 1 ton of carbon emissions are evaluated (Reddy and Kumar, 2018; Raymond et al., 2021b).
Social life cycle assessment is a method to assess the direct and indirect social impacts of a project. Direct impacts refer to those stemming from the project itself, such as the benefits of geologic hazard mitigation on a community, while indirect impacts refer to those that occur further up the supply chain. One method to assess the indirect social impacts of a project are through existing social hotspot databases such as the Product Social Impact Life Cycle Assessment (PSILCA) database and the Social Hotspot Database (SHDB) (Benoit-Norris et al., 2012; Maister et al., 2020). However, these databases currently do not comprehensively cover geotechnical-related construction materials or assess emerging alternative construction methods (e.g., bio-inspired and bio-mediated technologies). Tools such as Design for Freedom have also recently been developed to highlight the resource intensity and equity of building construction material supply chains predominantly across the buildings sector (Grace Farms, 2022). These supply chains are analyzed and evaluated to provide a qualitative assessment of a variety of social sustainability indicators (e.g., labor practices, resource exploitation). While the ground improvement materials can differ from conventional building construction materials, Design for Freedom presents a social and environmental sustainability framework that can be applied to understand the sustainability of materials-intensive industries. Direct impacts such as those on construction workers and the local community should similarly be assessed through qualitative means.
MICP and MIDP are considered bio-mediated technologies since they directly use microbial activity in the treatment process. However, EICP is considered a bio-inspired technology because it uses a manufactured enzyme for treatment and does not involve microorganism activity (Khodadadi et al., 2017). Common benefits across each technology include the omission of carbon-emitting cement and the non-disruptive nature of treatment allowing, in many cases, ground improvement to be implemented below existing structures (Dejong et al., 2014; Khodadadi et al., 2017). Concerns across each technology include obtaining uniform treatment throughout the targeted soil volume and groundwater and soil contamination via unreacted process inputs or intermediary products resulting from the incomplete conversion of inputs (Hall et al., 2022b). In addition, due to the novelty of each technology, the duration and durability of treatment have not been robustly tested.
The applications, process inputs, and technological and environmental concerns of EICP, MICP, MIDP, and MID are summarized in Table 1. The technical feasibility and sustainability impacts of each technology are determined by factors including:
The bacterial and material inputs required for the biogeochemical process
The equipment installation and treatment methods required for implementation
The biogeochemical reaction rate
The durability of treatment, and whether treatment needs to be repeated over the service life of the system
Reagent utilization (i.e., the efficiency of each biogeochemical reaction)
The products and by-products of the overall biogeochemical process
Whether each biogeochemical reaction is performed to completion
EICP uses plant-derived (e.g., Jack beans) urease enzymes to induce urea hydrolysis, and when combined with calcium chloride calcium carbonate precipitation occurs (Eqs 1–5) (Khodadadi et al., 2017; Almajed et al., 2018). Non-fat milk powder can also be used as an additive in the EICP process to stabilize the enzymes (Martin et al., 2021). EICP can be applied as a treatment to the soil via three mechanisms depending on application purpose and soil properties: mechanical mixing and compaction, solution injection using traditional grouting methods, and surface spraying (Martin et al., 2020; Woolley et al., 2020; Arab et al., 2021). EICP improves the strength of soils, such as the shear strength, by cementing soil grains together (Almajed et al., 2021).
The reaction rate of EICP is governed by the concentration of inputs, the source of the urease enzyme, which influences enzyme activity, the temperature and pH of the system and the soil type (Saif et al., 2022). These parameters must be optimized to obtain a slow reaction rate to reduce precipitate clogging near the injection points (van Paassen et al., 2010b). Experimental studies have presented varied optimal concentrations for urea and calcium chloride due to differences in urease concentration and enzymatic activity, as well as environmental conditions (Almajed et al., 2019; Ahenkorah et al., 2021).
While EICP eliminates the need for large volumes of cement, cost and environmental concerns still exist. For example, the cost of the urease enzyme has been highlighted as a concern (Khodadadi et al., 2017). Environmental concerns of EICP are the production of ammonium, which contributes to eutrophication, and chloride ions which contribute to salinisation and can corrode steel (Eqs 2, 4). If the overall reaction does not occur to completion, ammonia may also be introduced as an intermediary product to the groundwater (Eq. 1). When applied to the surface layer of the soil, the introduction of urea may result in nitrous oxide and ammonia emissions to the air (Raymond et al., 2021b). However, at depth, it is unlikely for these emissions to occur.
MICP via urea hydrolysis has been the most widely researched MICP pathway. MICP relies on introducing cultivated microorganisms, most often Sporoscarcina pasteurii which carry the urease enzyme, urea, and calcium chloride to the soil that is to be treated through augmentation (van Paassen et al., 2010b). Stimulation of microorganisms native to the soil to be treated can also be conducted to achieve MICP (Burbank et al., 2011; Burbank et al., 2012; Gomez et al., 2014; Gomez et al., 2017). MICP can be applied below the surface through injection of the microorganisms and substrates. MICP can improve the load-bearing capacity of soils and provide liquefaction mitigation (Whiffin et al., 2007; Montoya et al., 2013).
The MICP process via urea hydrolysis is as follows:
Similar to EICP, the rate of substrate injection must be controlled to avoid precipitate clogging at the injection points (Mortensen et al., 2011). The efficiency of the MICP process is dependent on the rate of ureolysis and treatment formula used (Martinez et al., 2013). This influences the applicability of MICP as a method to produce uniform treatment over large areas (van Paassen et al., 2010a).
Among the environmental concerns of MICP, ammonium chloride is produced as a by-product of the MICP process and must be removed or treated to reduce the risk of eutrophication and salinisation of groundwater. Rinsing processes have been introduced to remove ammonium from the treated soil, however, this produces wastewater which must be treated (Lee et al., 2019; San Pablo et al., 2020). Regarding costs, bio-stimulation of native organisms may be favored over bio-augmentation as this requires the construction and maintenance of on-site bioreactors (Sharma et al., 2022).
MIDP is a two-mechanism hazard mitigation process that relies on microbial denitrification to produce biogenic gas for desaturation and calcium carbonate precipitation for biocementation. MIDP uses the user-supplied substrate recipe of calcium acetate and calcium nitrate. Acetate acts as the electron donor (provides energy to the microbes) to facilitate microbial reduction of the nitrate to inert nitrogen gas through denitrification. Dissolved inorganic carbon (i.e., CO2, CO32−, HCO3−, and H2CO3) is also produced during denitrification, which when combined with the provided calcium leads to calcium carbonate precipitation. Biogenic gasses desaturate the soil, thereby reducing the liquefaction potential of soil by dampening pore pressure build-up during a cyclic shaking event (Eqs 11–16) (Dejong et al., 2014; O’Donnell et al., 2017). The precipitation of calcium carbonate mitigates liquefaction through the same hazard mitigation method as MICP, by improving the strength and dilatancy of soil (Eq. 17) (O’Donnell et al., 2017; Hall, 2021).
When aiming to mitigate liquefaction, MID may be preferred over MIDP since treatment via desaturation is reached before sufficient carbonate precipitation occurs to provide liquefaction mitigation (Hall et al., 2022b). Additionally, a greater amount of calcium acetate and calcium nitrate are required for carbonate precipitation, increasing costs and environmental impacts.
An advantage of MID and MIDP is that microorganisms do not need to be cultivated and injected into the soil since denitrifying microorganisms are widely found across various soil types, reducing costs (Hall et al., 2018). Another advantage of the processes is that users extract groundwater from the treatment site to dissolve and mix the substrates then, this water is re-introduced to the ground in a closed system with no external input of water. Existing groundwater technology, including injection and extraction wells, can be used to implement MID and MIDP (Moug et al., 2022).
Ongoing field trials of MID have demonstrated a desaturation persistence of at least 92 days, the length of the experiment when monitoring ceased (Moug et al., 2022). While longer-term trials of MID have not been conducted yet and the durability of desaturation has not been robustly tested, previous studies have indicated that abiotic desaturation can persist for up to 26 years (Okumura, 2006).
Benefits of MID and MIDP are the lack of waste products generated. For example, when the MID and MIDP reactions occur to completion, the products, nitrogen gas and carbon dioxide, do not need to be removed as is with EICP and MICP. It is unlikely that a significant amount of carbon dioxide will be released to the atmosphere through this process because of the typical pH levels of application sites and the high solubility of CO2, leading the majority of produced CO2 to remain in solution (Hall, 2021).
Complications of MID and MIDP include the impact that the primary and intermediary products can have on technical feasibility and environmental impacts. For example, the hydraulic conductivity of the soil may be reduced by the production of nitrogen gas and carbon dioxide gas and this may impact flow of the substrates through the soil (Stallings Young et al., 2021). Further, if the reaction is incomplete, process intermediates (primarily nitrite, but potentially nitrous and nitric oxide) may accumulate (Hall, 2021). The accumulation of these intermediates are potentially toxic and harmful to the environment. Intermediates also inhibit denitrification and can lead to reduced precipitation and desaturation (Eqs 13, 15) (van Paassen et al., 2010a; Hall, 2021)).
We conducted a systematic literature review of published ground improvement studies, including journal articles, conference papers, book chapters and industry reports. We completed a literature search following the Preferred Reporting Items for Systematic Reviews and Meta-Analyses (PRISMA) framework (Page et al., 2021). The databases utilized for the literature search were Web of Science, Scopus, and the American Society of Civil Engineers (ASCE) databases. We identified articles by searching abstracts using the search term: (“sustainability” OR “life cycle” OR “cost” OR “economic” OR “carbon” OR “energy”) AND (“EICP” OR “MICP” OR “carbonate precipitation” OR “microbially induced desaturation” OR “biocement” OR “biogrout” OR “bio-grout” OR “bio-mediated” OR “bio-mediated” OR “bioinspired” OR “bio-inspired”) AND (“assessment” OR “analysis” OR “footprint”). The resulting studies were then reviewed for relevance and duplication to arrive at a final set of studies that met our review criteria. We further sought to identify studies that may have been overlooked in the database searches by screening the references cited in the relevant studies that were found via database.
To respond to the research goals of this study, which include an assessment of the relative frequency of sustainability assessment of bio-mediated and bio-inspired ground improvement technologies compared to conventional methods, we also conducted a literature review of quantitative sustainability assessments applied to conventional ground improvement techniques. The flow chart for this literature search is presented in Supplementary Figure S1.
We reviewed each of the identified sustainability assessment studies with specific focus on the following parameters:
Application (e.g., EICP, MICP, MIDP)
Functional unit
System boundary
Life cycle inventory
Biogeochemical reactions
Life cycle impact assessment
Results
Uncertainty assessment
The goal of examining these parameters is to examine variability across the methods and modeling choices in existing studies, which determines the comparability and comprehensiveness of studies that reflect the current state of practice. The greater the variability in methods and key assumptions, the less comparable study findings are likely to be.
We also document the findings of the reviewed studies, with the goal of making recommendations for reducing the environmental impacts and cost of bio-mediated applications.
Scopus returned 21 references, Web of Science returned 18 references, and the ASCE database returned two references. As shown in Figure 1, we screened these references for relevance to identify ground improvement-related EICP, MICP, MID or MIDP studies such as those that reference soil stabilization or liquefaction mitigation. Only studies that completed a quantitative sustainability assessment, such as an LCA, an LCCA, S-LCA were chosen for this review. One study identified is a techno-economic analysis (TEA), however, this is considered as an LCCA in this review since the methods followed align with those of an LCCA. Studies that assessed bio-mediated or bio-inspired methods that can be applied to ground improvement even when they were not used in ground improvement applications were also included; however, those applied to structures, such as carbonate precipitation within concrete, were not included since the function of these technologies differs to ground improvement. After screening relevant studies for duplicates, five unique studies remained. After screening the citations of these studies, an additional two studies were identified that met the search criteria, one of which was an industry report. An additional paper was identified outside of the systematic review via knowledge of the authors. We identified eight sustainability assessment studies in total. Seven of these studies are life cycle assessments, two of which also include a life cycle cost assessment, and one is the TEA considered as an LCCA.
Eight studies published between 2009 and 2023 were identified, three of which evaluate EICP, three evaluate MICP, one evaluates MID and MIDP, and one evaluates MID only. A summary of these studies is provided in Table 2. Six of these studies assess either one or multiple bio-mediated technologies or pathways and compare them to a traditional, cement-based ground improvement technique. Meanwhile, Martin et al. (2020) only provides a hotspot analysis of EICP. Each study is a process-based LCA considering environmental impacts or cost. None of the identified studies includes a social life cycle assessment.
TABLE 2. Summary of identified sustainability assessment studies of bio-mediated and bio-inspired geologic hazard mitigation techniques.
The life cycle studies considered ground improvement applications, including soil stabilization for roadways and railways, foundation reinforcement, and liquefaction mitigation. Porter et al. (2021) did not provide a specific application for MICP, instead focusing on the production of calcium carbonate only. Each EICP and MICP life cycle study considered a urea hydrolysis pathway. Porter et al. (2021) also evaluated another five pathways for MICP including denitrification (MIDP).
The frequency of LCSA applied to biotechnologies compared to conventional technologies has been uneven over time, but biotechnologies are over-represented in the literature relative to their frequency of application in real world projects. Figure 2 shows the results of literature reviews for both biotechnologies and conventional based on the number of studies published per year between 2009 (the first year an LCSA of a ground improvement technology was published) and 2023. What is evident, is that there has been uneven growth in LCSA-related studies since 2009, but that biotechnology LCSAs are growing in popularity alongside conventional ground improvement LCSAs.
FIGURE 2. LCSA studies of conventional and bio-mediated or bio-inspired ground improvement technologies.
The functional unit of an LCSA serves as the basis for which technologies are evaluated over and compared (ISO, 2006a). The functional unit should specify the service provided by a ground improvement project and include relevant performance requirements such as the target strength of a soil structure (Kendall et al., 2018). Further, for bio-mediated technologies in particular, because they often require multiple treatments over the service life of a project, the functional unit of a study should specify the duration of the project.
The functional unit of each reviewed study varies between those focused only on mass of carbonate produced or an area or volume of soil treated and those that also included performance requirements for the technologies considered (Table 2). Martin et al. (2020) and Salemans & Blauw (2010), use the most comprehensive functional units, specifying the volume of soil treated, target strength, and location of the study. Salemans and Blauw (2010) also specify soil grain size in their functional unit. No study considers service life in the functional unit. As such, no study evaluates impacts from potential re-applications of bio-treatment required over the service life of the projects.
Poorly defined functional units can result in an unfair comparison across technologies. For example, Porter et al. (2021), compare the production of 1 kg of precipitated calcium carbonate from various different pathways; however, leaving performance requirements out of the functional unit omits potential differences in the service provided by each pathway. For example, MIDP produces calcium carbonate as well as nitrogen which provides mitigation against liquefaction through MID. For a fair comparison, each system must provide the same services.
Each reviewed study only considers the construction stage of the assessed ground improvement projects and within this stage, raw materials supply is the most commonly assessed process (Table 3). Suer et al. (2009), provide the most comprehensive system boundary considering materials transportation, equipment mobilization, on-site equipment use, biogeochemical emissions, and construction waste transportation in addition to raw materials supply. The authors only, however, provide a qualitative assessment of biogeochemical emissions. No studies assess the impacts of quality assurance and quality control and Salemans and Blauw were the only authors to evaluate the impacts of biogeochemical emissions treatment, considering wastewater treatment to remove ammonium chloride from the system. Raymond et al. evaluate impacts of rinsing to remove ammonium, however, they do not include wastewater treatment in their analysis. All studies except Deng et al. (2021), Hall et al. (2022a), and Suer et al. (2009) include a quantitative assessment of the impact that bio-mediated process emissions have on the environment. Deng et al. (2021) and Salemans and Blauw (2010), are the only authors to evaluate the impacts of bacteria cultivation on total MICP impacts.
TABLE 3. Life cycle processes for the construction stage considered in the system boundary of each reviewed study.
By omitting the use stage of ground improvement projects, the impact of treatment durability can not be assessed and the total sustainability impacts may be underestimated if potential treatment re-applications are not evaluated. While the durability of treatment is currently unknown for EICP, MICP and MIDP, sensitivity analysis can be employed to assess the potential impact of treatment re-applications over the service life of the infrastructure.
Transparency of methods used to develop a life cycle inventory (LCI) for each technology vary, with Suer et al. (2009) providing the most detailed information including primary data sources. Hall et al. (2022a) also use primary data from industry and provide transparent unit costs and calculations for their cost assessment. Due to lack of transparency regarding the models used by the other authors, reproducibility of these studies is low. The LCI databases utilized also vary and it should be noted that many studies utilize datasets from old databases which may not reflect current industry practices (Table 4). Further, life cycle inventory datasets are not currently available for key material inputs such as jack beans for EICP, with Martin et al. using a dataset on soybean production as a proxy for Jack Beans (Martin et al., 2020). None of the studies provide a complete life cycle inventory for the technologies assessed and this in addition to low reproducibility means that it is difficult to use this data in other sustainability assessments such as extensions of those published or applications to broader construction projects.
Consideration of the factors that influence the sustainability and technical feasibility of bio-mediated technologies, as outlined in Section 2, is limited for each study. All studies assume that reagent utilization is 100% efficient, omitting impacts from potentially unreacted material inputs, and only one study evaluates the impact of intermediary products on the environment. Salemans and Blauw (2010) assume that incomplete denitrification takes place for MID where 10% of the nitrogen input into the system for MID is converted to NOx and N2O. No study evaluates the reaction rate of the relevant biogeochemical processes and none address durability of the treatment provided. As such, the studies potentially underestimated impacts as it is likely that treatment will need to be repeated over the service life of the infrastructure project. Further, the studies do not allow for a comparison of technical feasibility since the reaction rate and durability of each treatment is unknown.
Global warming potential and eutrophication potential are the most commonly assessed impact categories in the identified studies with six studies quantifying each (Table 5). Four studies evaluate energy use and only two assess the cost of the evaluated technologies. No study assesses the social impacts of bio-mediated or bio-inspired ground improvement techniques. Salemans and Blauw (2010) assess the most comprehensive list of impact categories. However, the impacts are presented as a normalized Eco-Indicator 99 value making it difficult to discern the individual impacts and compare them with other technologies. Eco-Indicator 99 is a method used to weight various environmental impacts, producing a single impact score which can be used to compare different technologies (Goedkoop, 2007). While Suer et al. (2009) only quantify the energy use and cost of MICP, the authors do provide a qualitative assessment of the additional impact categories that each system input contributes to. The impact assessment models used vary across the studies making it difficult to complete direct comparisons of results (Table 4).
A direct comparison of results cannot be achieved since the functional unit of each study varies and not enough information has been provided by the authors to normalize results to a consistent functional unit. For the studies that provide a hotspot analysis of biomedated or bio-inspired technologies, while they do not evaluate impacts relative to traditional technologies, the results can be used to determine the scale of possible impact reductions. For example, the impact of process emissions on the total global warming potential and eutrophication potential of each technology is reported to be significant compared to material production impacts which suggests that notable reductions could be achieved by capturing, avoiding, or treating these emissions. Additionally, data from these studies can be used to determine the potential impact reductions achieved by replacing commercial material inputs with alternative materials such as waste products from other industries. Further, some conclusions can be drawn regarding the ideal study scope for bio-mediated and bio-inspired studies as described below.
Project scale assessments are preferred over laboratory-scale studies, because laboratory-scale assessments can distort performance and cost estimates. For example, LCCA calculations based on laboratory-scale data typically overestimate costs due to use of high quality reagents that are not bought in bulk. Suer et al. (2009) report the cost of MICP at $2,554 per m3 of soil treated for a project-scale assessment and note that raw materials account for about 20% of the total cost. However, for treatment of a 0.14 m3 lab-scale soil column, Raymond et al. (2023) report a materials cost of $1,800. Scaling the Raymond et al. (2023) results leads to a cost of $12,857 per m3 suggesting that lab-scale cost data does not scale well to project applications.
Project-scale assessments are also more ideal because processes such as equipment rental and mobilization can only be modeled at this scale. While all other studies omit these processes, Suer et al. (2009), and Hall et al. (2022a) show the importance of including equipment rental, mobilization and use emissions in LCSA. These can have high impacts on the energy use and cost of ground improvement techniques. Suer et al. (2009) find that these processes contribute to 61.4% of the energy use of jet grouting and 22% of the energy use of MICP. For cost, these contributions are 62.6% and 77.1% for jet grouting and MICP, respectively. Hall et al. (2022a) find that equipment and labor for installation and mobilization account for 57.8% of the total cost of MID and 44.5% of the total cost of permeation grouting.
For MICP, bacteria cultivation is another stage that should be evaluated since Deng et al. (2021) find that this accounts for 20% of materials production impacts. The system boundary for MICP must also include treatment of the effluent that remains after ammonium rinsing since this can present a high contribution to total results (Salemans and Blauw, 2010).
Only two studies complete a sensitivity analysis of model input parameters and two studies performed scenario analyses. Alotaibi et al. investigate the impact of field emission assumptions and using waste non-fat milk as an input to the EICP process. By eliminating field emissions and using waste milk, the GWP of EICP can be reduced by 54% from a case where virgin milk powder is used and an upper bound of field emissions is considered. The eutrophication potential can be reduced by 92% for the same change in scenario. The authors do not, however, provide recommendations for how to eliminate EICP field emissions. The authors also provide a sensitivity analysis on the target unconfined compressive strength (UCS) of the soil demonstrating an exponential increase in environmental impacts as target UCS increases. The authors compare EICP to MICP using data from Deng et al. (2021) and show that at a target UCS of less than 1.5 MPa, EICP is favorable over MICP with regards to GWP. Deng et al. (2021), also demonstrate an exponential increase in GWP and energy demand as the target UCS of MICP-treated soil is increased. Deng et al. (2021) evaluate the relationship between MICP treatment, quantified as calcium carbonate content, and UCS based on a review of ten experimental MICP studies.
Raymond et al. (2023) provide the most comprehensive scenario analyses for the pathways of MICP assessing the impact of: reducing urea inputs, eliminating sodium acetate inputs, rate of ureolytic stimulation and augmentation, and ammonium rinsing. The authors demonstrate that a reduced urea input can reduce the GWP of MICP by roughly 20% and this in addition to removing sodium acetate as an input to the process can reduce the GWP by 29%. For this second scenario, the cost of MICP is reduced by 55%. Experimental work has shown that the urea reduction modeled by Raymond et al. (2023) can provide the same level of MICP treatment as provided by the baseline study (Gomez et al., 2018). The authors do not provide any potential differences in performance due to the removal of sodium acetate from the MICP inputs. Raymond et al. (2023) also report that a case with low ureolytic stimulation results in the lowest impacts while high ureolytic augmentation creates the highest impacts across GWP, eutrophication potential, and cost. While ammonium rinsing has a low impact on the GWP and cost of MICP, it provides significant reductions in eutrophication potential. For a case with low ureolytic stimulation, ammonium rinsing can reduce the eutrophication potential of MICP by 66%. Raymond et al. (2023), do not, however, discuss treatment solutions, or their environmental impacts, for the rinsed ammonium.
To develop and implement sustainable geologic hazard mitigation techniques, critical issues highlighted above for bio-mediated and bio-inspired solutions must be resolved through alternative methods such as those presented in Table 6. This includes using substitutes for materials such as urea and urease enzymes, and reducing transportation impacts to reach the full potential of environmental benefits of EICP, MICP, and MIDP.
TABLE 6. Alternative, sustainable design recommendations for bio-mediated and bio-inspired ground improvement technologies.
There are also barriers to implementation at the project scale. An example relates to the requirement of MICP bacteria cultivation on site which is yet to be demonstrated on a large-scale (Terzis and Laloui, 2019). Another barrier is the disconnect between existing engineering design codes and the design methods that relate to bio-mediated and bio-inspired technologies. Related to this is the lack of formal education of engineers and scientists in biogeotechnics (DeJong et al., 2010). Further, for successful implementation of these solutions, a shift in how hazard mitigation projects are currently managed is needed since these projects require assessment from a biological perspective which is not current practice in geotechnical engineering. Finally, use of bio-mediated and bio-inspired geotechnical technologies requires interdisciplinary collaboration such as between geotechnical engineers and ecologists across each project stage of a biogeotechnical hazard mitigation project to ensure success (DeJong et al., 2015).
With regards to impact, each potential bio-mediated or bio-inspired alternative must be evaluated for its sustainability and technological performance to assess whether they reduce social, environmental, and economic impacts on a life cycle basis. Quantitative sustainability assessment, such as through LCSA, must be adopted to assess the technological and sustainability impacts of such technologies and drive their sustainable development. Clear LCSA guidelines are needed to facilitate the production of high-quality sustainability data on traditional, bio-mediated and bio-inspired technologies to allow for useful comparisons and to guide decision-making (Kendall et al., 2018; Song et al., 2020; Samuelsson et al., 2021). One issue regarding the adoption of LCA for ground improvement applications is the high cost of reference life cycle assessment data, which are often derived from commercial databases and are crucial for conducting an LCA (Cho et al., 2017). A standardized approach to geotechnical LCSA can assist in developing an open database of comparable and transparent life cycle data. Guidelines for standardizing geotechnical LCSA would also improve reproducibility of studies, would allow for comparison across studies, could facilitate integration into the geotechnical design process, and could lower the cost and barriers to conducting LCSA by supporting the development of open source reference life cycle inventories for geotechnical processes and technologies.
Social assessment is important in holistically assessing the sustainability of a project and such guidelines must facilitate the assessment of the direct and indirect social impacts across ground improvement projects. While this is yet to be done for geotechnical projects, some construction-related studies have presented social impact assessments. For example, in one study comparing concrete and steel for use as building materials in Iran, the authors found that for impacts in many categories including health and safety and cultural heritage, steel resulted in more impacts, while concrete materials resulted in higher impacts for working conditions (Hosseinijou et al., 2014). Further, Dong and Ng (2015) present a social impact assessment methodology, the Social-Impact Model of Construction (SMoC), to evaluate construction projects based on expert surveys to determine the most important social impacts. The authors find that social impacts are higher during materials procurement than on-site construction processes.
For the successful project-scale and sustainable implementation of bio-mediated and bio-inspired geotechnical solutions, the barriers listed above must be addressed, including the lack of existing guidance regarding completion of LCSA. Adoption of LCSA across geologic hazard mitigation has the potential to reduce the social, environmental and economic impacts that infrastructure and the built environment have on communities. Facilitating this adoption through a clear and comprehensive methodology for LCSA is crucial in realizing the influence that LCSA can have on sustainable development.
Emerging biotechnologies, including EICP, MICP and MIDP, are steadily gaining popularity as alternative ground improvement techniques for geologic hazard mitigation. While sustainability gains have been a key driving factor behind the development of these technologies, each technology still carries concerns regarding environmental impact, and the social impacts of these technologies are currently unknown. Further, current sustainability assessment methods do not allow for the production of high-quality and comparable data. For example, existing studies do not use comprehensive functional units which address the service provided by each technology. Existing studies also do not assess the whole life cycle of ground improvement projects from site investigation to construction, use, and end-of-life, though this is needed for a comprehensive assessment. Thesestudies are largely not reproducible or transparent in their data collection methods and outdated datasets are commonly used which limits applicability of these studies to existing and future projects. A major drawback of the studies examined in this review is the lack of assessment of uncertainty across the project life cycles and potential by-products regarding the core biogeochemical processes that are induced by bio-mediated and bio-inspired technologies.
This review shows that quantitative sustainability assessment, such as LCSA, should be used to understand the impacts of bio-mediated technologies and identify areas where impact reductions can be made. Clear guidelines are needed to produce open, reproducible, and comparable LCSA for geotechnical applications such as ground improvement. These guidelines should provide clear advice about the selection of functional unit and system boundaries. Further, guidelines are needed to assist in the development of a life cycle inventory and completion of a life cycle impact assessment and uncertainty assessment. For emerging technologies, uncertainty assessment is particularly crucial since there is limited data available regarding the project-scale performance of such technologies as well as the industrial-scale production of bacterial and material inputs.
LCSA must include a social assessment and must be completed alongside a technological assessment. This must include an evaluation of impacts due to biogeochemical reactions as well as an assessment of performance such as through reagent utilization, degree of ground improvement provided for the given function, reaction rate, and durability. In tandem with these comprehensive biogeotechnical LCSAs, those of conventional ground improvement technologies must continue in development to provide baseline sustainability metrics against which emerging technologies can be compared.
AF, CH, and AK conceptualized the study. AF conducted the literature review and wrote the initial draft. CH and AK reviewed and edited the draft. All authors contributed to the article and approved the submitted version.
This material is based upon work supported by the Engineering Research Center Program of the National Science Foundation (NSF) under NSF Cooperative Agreement EEC-1449501. Any opinions, findings, and conclusions or recommendations expressed in this material are those of the authors and do not necessarily reflect those of the NSF. Funds were received from the University of California, Davis Library to support open access publication.
The authors declare that the research was conducted in the absence of any commercial or financial relationships that could be construed as a potential conflict of interest.
All claims expressed in this article are solely those of the authors and do not necessarily represent those of their affiliated organizations, or those of the publisher, the editors and the reviewers. Any product that may be evaluated in this article, or claim that may be made by its manufacturer, is not guaranteed or endorsed by the publisher.
The Supplementary Material for this article can be found online at: https://www.frontiersin.org/articles/10.3389/feart.2023.1211574/full#supplementary-material
Ahenkorah, I., Rahman, M. M., Karim, M. R., Beecham, S., and Saint, C. (2021). A review of enzyme induced carbonate precipitation (EICP): The role of enzyme kinetics. Sustain. Chem. 2, 92–114. doi:10.3390/suschem2010007
Almajed, A., Khodadadi Tirkolaei, H., and Kavazanjian, E. (2018). Baseline investigation on enzyme-induced calcium carbonate precipitation. J. Geotech. Geoenvironmental Eng. 144, 04018081. doi:10.1061/(ASCE)GT.1943-5606.0001973
Almajed, A., Tirkolaei, H. K., Kavazanjian, E., and Hamdan, N. (2019). Enzyme induced biocementated sand with high strength at low carbonate content. Sci. Rep. 9, 1135. doi:10.1038/s41598-018-38361-1
Almajed, A., Lateef, M. A., Moghal, A. A. B., and Lemboye, K. (2021). State-of-the-Art review of the applicability and challenges of microbial-induced calcite precipitation (MICP) and enzyme-induced calcite precipitation (EICP) techniques for geotechnical and geoenvironmental applications. Crystals 11, 370. doi:10.3390/cryst11040370
Alotaibi, E., Arab, M. G., Abdallah, M., Nassif, N., and Omar, M. (2022). Life cycle assessment of biocemented sands using enzyme induced carbonate precipitation (EICP) for soil stabilization applications. Sci. Rep. 12, 6032. doi:10.1038/s41598-022-09723-7
Andrus, R. D., and Chung, R. M. (1995). Ground improvement techniques for liquefaction remediation near existing lifelines. Gaithersburg, MD: National Institute of Standards and Technology. doi:10.6028/NIST.IR.5714
Arab, M. G., Alsodi, R., Almajed, A., Yasuhara, H., Zeiada, W., and Shahin, M. A. (2021). State-of-the-Art review of enzyme-induced calcite precipitation (EICP) for ground improvement: Applications and prospects. Geosciences 11, 492. doi:10.3390/geosciences11120492
Basu, D., Misra, A., and Puppala, A. J. (2015). Sustainability and geotechnical engineering: Perspectives and review. Can. Geotech. J. 52, 96–113. doi:10.1139/cgj-2013-0120
Benoit-Norris, C., Cavan, D. A., and Norris, G. (2012). Identifying social impacts in product supply chains:overview and application of the social hotspot database. Sustainability 4, 1946–1965. doi:10.3390/su4091946
Burbank, M. B., Weaver, T. J., Green, T. L., Williams, B. C., and Crawford, R. L. (2011). Precipitation of calcite by indigenous microorganisms to strengthen liquefiable soils. Geomicrobiol. J. 28, 301–312. doi:10.1080/01490451.2010.499929
Burbank, M. B., Weaver, T. J., Williams, B. C., and Crawford, R. L. (2012). Urease activity of ureolytic bacteria isolated from six soils in which calcite was precipitated by indigenous bacteria. Geomicrobiol. J. 29, 389–395. doi:10.1080/01490451.2011.575913
Cabeza, L. F., Rincón, L., Vilariño, V., Pérez, G., and Castell, A. (2014). Life cycle assessment (LCA) and life cycle energy analysis (lcea) of buildings and the building sector: A review. Renew. Sustain. Energy Rev. 29, 394–416. doi:10.1016/j.rser.2013.08.037
Chang, I., Lee, M., and Cho, G.-C. (2019). Global CO2 emission-related geotechnical engineering hazards and the mission for sustainable geotechnical engineering. Energies 12, 2567. doi:10.3390/en12132567
Chen, H.-J., Huang, Y.-H., Chen, C.-C., Maity, J. P., and Chen, C.-Y. (2019). Microbial induced calcium carbonate precipitation (MICP) using pig urine as an alternative to industrial urea. Waste Biomass Valorization 10, 2887–2895. doi:10.1007/s12649-018-0324-8
Cho, H.-T., Kwon, S.-H., and Han, J.-G. (2017). Estimation of environmental load of geotechnical structure using multiple regression analysis. KSCE J. Civ. Eng. 21 (5), 1581–1586. doi:10.1007/s12205-017-0419-y
Crane, L., Ray, H., Hamdan, N., and Boyer, T. H. (2022). Enzyme-induced carbonate precipitation utilizing fresh urine and calcium-rich zeolites. J. Environ. Chem. Eng. 10, 107238. doi:10.1016/j.jece.2022.107238
Cui, M. J., Zheng, J. J., Chu, J., Wu, C. C., and Lai, H. J. (2021). Bio-mediated calcium carbonate precipitation and its effect on the shear behaviour of calcareous sand. Acta Geotech. 16, 1377–1389. doi:10.1007/s11440-020-01099-0
DeJong, J. T., Mortensen, B. M., Martinez, B. C., and Nelson, D. C. (2010). Bio-mediated soil improvement. Ecol. Eng. 36, 197–210. doi:10.1016/j.ecoleng.2008.12.029
DeJong, J., Tibbett, M., and Fourie, A. (2015). Geotechnical systems that evolve with ecological processes. Environ. Earth Sci. 73 (3), 1067–1082. doi:10.1007/s12665-014-3460-x
Dejong, J. T., Soga, K., Kavazanjian, E., Burns, S., Van Paassen, L. A., Al Qabany, A., et al. (2014). “Biogeochemical processes and geotechnical applications: Progress, opportunities and challenges,” in Bio- and chemo-mechanical Processes in geotechnical engineering conference proceedings (London: ICE Publishing), 143–157. doi:10.1680/bcmpge.60531.014
Deng, X., Li, Y., Liu, H., Zhao, Y., Yang, Y., Xu, X., et al. (2021). Examining energy consumption and carbon emissions of microbial induced carbonate precipitation using the life cycle assessment method. Sustainability 13, 4856. doi:10.3390/su13094856
Dong, Y. H., and Ng, S. T. (2015). A social life cycle assessment model for building construction in Hong Kong. Int. J. Life Cycle Assess. 20, 1166–1180. doi:10.1007/s11367-015-0908-5
Goedkoop, M. (2007). The eco-indicator 99 methodology. J. Life Cycle Assess. Jpn. 3, 32–38. doi:10.3370/lca.3.32
Gomez, M. G., Anderson, C. M., DeJong, J. T., Nelson, D. C., and Lau, X. H. (2014). “Stimulating in situ soil bacteria for bio-cementation of sands,” in Geo-congress 2014: Geo-characterization and modeling for sustainability (American Society of Civil Engineers), 1674–1682. doi:10.1061/9780784413272.164
Gomez, M. G., Anderson, C. M., Graddy, C. M. R., DeJong, J. T., Nelson, D. C., and Ginn, T. R. (2017). Large-scale comparison of bioaugmentation and biostimulation approaches for biocementation of sands. J. Geotech. Geoenvironmental Eng. 143, 04016124. doi:10.1061/(ASCE)GT.1943-5606.0001640
Gomez, M. G., Graddy, C. M. R., DeJong, J. T., Nelson, D. C., and Tsesarsky, M. (2018). Stimulation of native microorganisms for biocementation in samples recovered from field-scale treatment depths. J. Geotech. Geoenvironmental Eng. 144, 04017098. doi:10.1061/(ASCE)GT.1943-5606.0001804
Grace Farms (2022). Design for FREEDOM. Des. Freedom. Available at: https://www.designforfreedom.org/ (Accessed September 5, 2022).
Hall, C. A., Hernandez, G., Darby, K. M., van Paassen, L., DeJong, J., Wilson, D., et al. (2018). Centrifuge model testing of liquefaction mitigation via denitrification-induced desaturation. Göttingen: American Society of Civil Engineers, 117–126. doi:10.1061/9780784481455.011
Hall, C. A., van Paassen, L. A., Kamalzare, S., Parmantier, D., and Kavazanjian, E. (2022a). Techno-economic assessment of liquefaction mitigation by microbially induced desaturation. American Society of Civil Engineers, 91–100. doi:10.1061/9780784484449.008
Hall, C. A., van Turnhout, A., van Paassen, L., Kavazanjian, E., and Rittmann, B. (2022b). Multi-phase biogeochemical model for microbially induced desaturation and precipitation. EGUsphere Prepr, 1–22. doi:10.5194/egusphere-2022-1419
Hall, C. A. (2021). Biogeochemical modeling of microbially induced desaturation and precipitation. Available at: https://keep.lib.asu.edu/_flysystem/fedora/c7/Hall_asu_0010E_20719.pdf.
Harvey, J., Kendall, A., Lee, I.-S., Santero, N., Van Dam, T., and Wang, T. (2011). Pavement life-cycle assessment workshop: Discussion summary and guidelines.
Hosseinijou, S. A., Mansour, S., and Shirazi, M. A. (2014). Social life cycle assessment for material selection: A case study of building materials. Int. J. Life Cycle Assess. 19, 620–645. doi:10.1007/s11367-013-0658-1
ISO (2006a). ISO 14040:2006: Environmental management – life cycle assessment – principles and framework. Geneva, Switzerland: International Organization for Standardization.
ISO (2006b). ISO 14044:2006: Environmental management – life cycle assessment – requirements and guidelines. Geneva, Switzerland: International Organization for Standardization.
Javadi, N., Khodadadi, H., Hamdan, N., and Kavazanjian, E. (2018). “EICP treatment of soil by using urease enzyme extracted from watermelon seeds,” in Ifcee 2018 (American Society of Civil Engineers), 115–124. doi:10.1061/9780784481592.012
Jefferis, S. A. (2008). “Moving towards sustainability in geotechnical engineering,” in GeoCongress 2008: Geosustainability and geohazard mitigation (New Orleans: American Society of Civil Engineers), 844–851. doi:10.1061/40971(310)105
Jørgensen, A., Le Bocq, A., Nazarkina, L., and Hauschild, M. (2007). Methodologies for social life cycle assessment. Int. J. Life Cycle Assess. 13, 96–103. doi:10.1065/lca2007.11.367
Kendall, A., Raymond, A. J., Tipton, J., and DeJong, J. T. (2018). Review of life-cycle-based environmental assessments of geotechnical systems. Proc. Inst. Civ. Eng. - Eng. Sustain. 171, 57–67. doi:10.1680/jensu.16.00073
Khodadadi, H. T., Kavazanjian, E., van Paassen, L., and DeJong, J. (2017). Bio-Grout Materials: A Review, 1–12. doi:10.1061/9780784480793.001
Khodadadi, T. H., Javadi, N., Krishnan, V., Hamdan, N., and Kavazanjian, E. (2020). Crude urease extract for biocementation. J. Mat. Civ. Eng. 32, 04020374. doi:10.1061/(ASCE)MT.1943-5533.0003466
Lee, M., and Basu, D. (2018). An integrated approach for resilience and sustainability in geotechnical engineering. Indian Geotech. J. 48, 207–234. doi:10.1007/s40098-018-0297-3
Lee, S., and Kim, J. (2020). An experimental study on enzymatic-induced carbonate precipitation using yellow soybeans for soil stabilization. KSCE J. Civ. Eng. 24, 2026–2037. doi:10.1007/s12205-020-1659-9
Lee, M., Gomez, M. G., San Pablo, A. C. M., Kolbus, C. M., Graddy, C. M. R., DeJong, J. T., et al. (2019). Investigating ammonium by-product removal for ureolytic bio-cementation using meter-scale experiments. Sci. Rep. 9, 18313. doi:10.1038/s41598-019-54666-1
Maister, K., Di Noi, C., Ciroth, A., and Srocka, M. (2020). Psilca V.3 - database documentation. Berlin: GreenDelta.
Martin, K. K., Khodadadi, T. H., Chester, M., and Kavazanjian, E. (2020). “Hotspot life cycle assessment for environmental impacts of EICP for ground improvement,” in Geo-congress 2020: Biogeotechnics (American Society of Civil Engineers), 321–329. doi:10.1061/9780784482834.035
Martin, K., Tirkolaei, H. K., and Kavazanjian, E. (2021). Enhancing the strength of granular material with a modified enzyme-induced carbonate precipitation (EICP) treatment solution. Constr. Build. Mat. 271, 121529. doi:10.1016/j.conbuildmat.2020.121529
Martinez, B. C., DeJong, J. T., Ginn, T. R., Montoya, B. M., Barkouki, T. H., Hunt, C., et al. (2013). Experimental optimization of microbial-induced carbonate precipitation for soil improvement. J. Geotech. Geoenvironmental Eng. 139, 587–598. doi:10.1061/(ASCE)GT.1943-5606.0000787
Mitchell, J. K. (2012). “Mitigation of liquefaction potential of silty sands,” in Rom research to practice in geotechnical engineering (American Society of Civil Engineers), 433–451. doi:10.1061/40962(325)15
Montoya, B. M., Dejong, J. T., and Boulanger, R. W. (2013). Dynamic response of liquefiable sand improved by microbial-induced calcite precipitation. Géotechnique 63, 302–312. doi:10.1680/geot.SIP13.P.019
Mortensen, B. M., Haber, M. J., DeJong, J. T., Caslake, L. F., and Nelson, D. C. (2011). Effects of environmental factors on microbial induced calcium carbonate precipitation. J. Appl. Microbiol. 111, 338–349. doi:10.1111/j.1365-2672.2011.05065.x
Moug, D. M., Sorenson, K. R., Khosravifar, A., Preciado, M., Stallings Young, E., van Paassen, L., et al. (2022). Field trials of microbially induced desaturation in low-plasticity silt. J. Geotech. Geoenvironmental Eng. 148, 05022005. doi:10.1061/(ASCE)GT.1943-5606.0002890
O’Donnell, S. T., Kavazanjian, E., and Rittmann, B. E. (2017). Midp: Liquefaction mitigation via microbial denitrification as a two-stage process. II: Micp. J. Geotech. Geoenvironmental Eng. 143, 04017095. doi:10.1061/(ASCE)GT.1943-5606.0001806
Omoregie, A. I., Ngu, L. H., Ong, D. E. L., and Nissom, P. M. (2019). Low-cost cultivation of Sporosarcina pasteurii strain in food-grade yeast extract medium for microbially induced carbonate precipitation (MICP) application. Biocatal. Agric. Biotechnol. 17, 247–255. doi:10.1016/j.bcab.2018.11.030
Page, M. J., McKenzie, J. E., Bossuyt, P. M., Boutron, I., Hoffmann, T. C., Mulrow, C. D., et al. (2021). The PRISMA 2020 statement: An updated guideline for reporting systematic reviews. BMJ 372, n71. doi:10.1136/bmj.n71
Parrish, K., and Chester, M. (2014). Life-cycle assessment for construction of sustainable infrastructure. Pract. Period. Struct. Des. Constr. 19, 89–94. doi:10.1061/(ASCE)SC.1943-5576.0000187
Porter, H., Mukherjee, A., Tuladhar, R., and Dhami, N. K. (2021). Life cycle assessment of biocement: An emerging sustainable solution? Sustainability 13, 13878. doi:10.3390/su132413878
Raymond, A. J., Kendall, A., and DeJong, J. T. (2020). Life cycle sustainability assessment (LCSA): A research evaluation tool for emerging geotechnologies. Geo-congress 2020. 330–339. doi:10.1061/9780784482834.036
Raymond, A. J., DeJong, J. T., Kendall, A., Blackburn, J. T., and Deschamps, R. (2021a). Life cycle sustainability assessment of geotechnical ground improvement methods. J. Geotech. Geoenvironmental Eng. 147, 04021161. doi:10.1061/(ASCE)GT.1943-5606.0002646
Raymond, A. J., Kendall, A., DeJong, J. T., Kavazanjian, E., Woolley, M. A., and Martin, K. K. (2021b). Life cycle sustainability assessment of fugitive Dust control methods. J. Constr. Eng. Manag. 147, 04020181. doi:10.1061/(ASCE)CO.1943-7862.0001993
Raymond, A. J., DeJong, J. T., and Kendall, A. (2023). “Life cycle sustainability assessment: A tool for civil engineering research prioritization and project decision making,” in Sustainable geo-technologies for climate change adaptation springer transactions in civil and environmental engineering. H. Hazarika, S. K. Haigh, H. Kanaya, B. Chaudhary, Y. Kochi, M. Muraiet al. (Singapore: Springer Nature), 175–184. doi:10.1007/978-981-19-4074-3_11
Reddy, K. R., and Kumar, G. (2018). “Addressing sustainable technologies in geotechnical and geoenvironmental engineering,” in Geotechnics for natural and engineered sustainable technologies: GeoNEst developments in geotechnical engineering. Editors A. M. Krishna, A. Dey, and S. Sreedeep (Singapore: Springer), 1–26. doi:10.1007/978-981-10-7721-0_1
Risken, J. L., Fraser, J. G., Rutter, H., and Gadsby, M. (2015). “Implications of sea level rise on liquefaction vulnerability in christchurch,” in Proceeding of the 6th International Conference on Earthquake Geotechnical Engineering, Christchurch, New Zealand, November 2015.
Saif, A., Cuccurullo, A., Gallipoli, D., Perlot, C., and Bruno, A. W. (2022). Advances in enzyme induced carbonate precipitation and application to soil improvement: A review. Materials 15, 950. doi:10.3390/ma15030950
Salemans, J. W. M., and Blauw, M. (2010). Life Cycle Analysis for four different ground improvement techniques. Delft: Deltares.
Samuelsson, I., Larsson, S., and Spross, J. (2021). Life cycle assessment and life cycle cost analysis for geotechnical engineering: Review and research gaps. IOP Conf. Ser. Earth Environ. Sci. 710, 012031. doi:10.1088/1755-1315/710/1/012031
San Pablo, A. C. M., Lee, M., Graddy, C. M. R., Kolbus, C. M., Khan, M., Zamani, A., et al. (2020). Meter-scale biocementation experiments to advance process control and reduce impacts: Examining spatial control, ammonium by-product removal, and chemical reductions. J. Geotech. Geoenvironmental Eng. 146, 04020125. doi:10.1061/(ASCE)GT.1943-5606.0002377
Sharma, M., Satyam, N., and Reddy, K. R. (2021). State of the art review of emerging and biogeotechnical methods for liquefaction mitigation in sands. J. Hazard. Toxic. Radioact. Waste 25, 03120002. doi:10.1061/(ASCE)HZ.2153-5515.0000557
Sharma, M., Satyam, N., and Reddy, K. R. (2022). Large-scale spatial characterization and liquefaction resistance of sand by hybrid bacteria induced biocementation. Eng. Geol. 302, 106635. doi:10.1016/j.enggeo.2022.106635
Shillaber, C. M., Mitchell, J. K., and Dove, J. E. (2016). Energy and carbon assessment of ground improvement works. I: Definitions and background. J. Geotech. Geoenvironmental Eng. 142, 04015083. doi:10.1061/(ASCE)GT.1943-5606.0001410
Song, X., Carlsson, C., Kiilsgaard, R., Bendz, D., and Kennedy, H. (2020). Life cycle assessment of geotechnical works in building construction: A review and recommendations. Sustainability 12, 8442. doi:10.3390/su12208442
Stallings Young, E. G., Mahabadi, N., Zapata, C. E., and Van Paassen, L. A. (2021). Microbial-induced desaturation in stratified soil conditions. Int. J. Geosynth. Ground Eng. 7, 37. doi:10.1007/s40891-021-00276-9
Suer, P., Hallberg, N., Carlsson, C., Bendz, D., and Holm, G. (2009). Biogrouting compared to jet grouting: Environmental (LCA) and economical assessment. J. Environ. Sci. Health Part A 44, 346–353. doi:10.1080/10934520802659679
United Nations Environment Programme (2020). Guidelines for social life cycle assessment of products and organisations 2020. Available at: https://wedocs.unep.org/20.500.11822/34554.
van Paassen, L. A., Daza, C. M., Staal, M., Sorokin, D. Y., van der Zon, W., and van Loosdrecht, M. C. M. (2010a). Potential soil reinforcement by biological denitrification. Ecol. Eng. 36, 168–175. doi:10.1016/j.ecoleng.2009.03.026
van Paassen, L. A., Ghose, R., van der Linden, T. J. M., van der Star, W. R. L., and van Loosdrecht, M. C. M. (2010b). Quantifying bio-mediated ground improvement by ureolysis: Large-scale biogrout experiment. J. Geotech. Geoenvironmental Eng. 136, 1721–1728. doi:10.1061/(ASCE)GT.1943-5606.0000382
Wang, Z., Zhang, N., Cai, G., Jin, Y., Ding, N., and Shen, D. (2017). Review of ground improvement using microbial induced carbonate precipitation (MICP). Mar. Georesources Geotechnol. 35, 1135–1146. doi:10.1080/1064119X.2017.1297877
Whiffin, V. S., van Paassen, L. A., and Harkes, M. P. (2007). Microbial carbonate precipitation as a soil improvement technique. Geomicrobiol. J. 24, 417–423. doi:10.1080/01490450701436505
Woolley, M. A., van Paassen, L., and Kavazanjian, E. (2020). Impact on surface hydraulic conductivity of EICP treatment for fugitive Dust mitigation. Geo-congress 2020. 132–140. doi:10.1061/9780784482834.015
Keywords: carbon footprint, life cycle sustainability assessment, LCA, geotechnics, MICP, MIDP, EICP
Citation: Faruqi A, Hall CA and Kendall A (2023) Sustainability of bio-mediated and bio-inspired ground improvement techniques for geologic hazard mitigation: a systematic literature review. Front. Earth Sci. 11:1211574. doi: 10.3389/feart.2023.1211574
Received: 24 April 2023; Accepted: 23 June 2023;
Published: 11 July 2023.
Edited by:
Nitin Tiwari, Purdue University, United StatesReviewed by:
Arif Ali Baig Moghal, National Institute of Technology Warangal, IndiaCopyright © 2023 Faruqi, Hall and Kendall. This is an open-access article distributed under the terms of the Creative Commons Attribution License (CC BY). The use, distribution or reproduction in other forums is permitted, provided the original author(s) and the copyright owner(s) are credited and that the original publication in this journal is cited, in accordance with accepted academic practice. No use, distribution or reproduction is permitted which does not comply with these terms.
*Correspondence: Alissa Kendall, YW1rZW5kYWxsQHVjZGF2aXMuZWR1
Disclaimer: All claims expressed in this article are solely those of the authors and do not necessarily represent those of their affiliated organizations, or those of the publisher, the editors and the reviewers. Any product that may be evaluated in this article or claim that may be made by its manufacturer is not guaranteed or endorsed by the publisher.
Research integrity at Frontiers
Learn more about the work of our research integrity team to safeguard the quality of each article we publish.