- 1Departamento de Geología, Facultad de Ciencias Físicas y Matemáticas, Universidad de Chile, Santiago, Chile
- 2Departamento de Geografía, Universidad de Concepción, Concepción, Chile
- 3Desert Research Institute, Reno, NV, United States
- 4Geoluminescence Dating Research Laboratory, Department of Geosciences, Institute of Archaeology, Baylor University, Waco, TX, United States
Andean mountain glaciers in central Chile are in a transitional zone between the seasonal influence of the mid-latitude westerlies and subtropical semiarid conditions to the north. Long-term glacial dynamics for these glaciers and their relationship with the paleoclimate during the late Quaternary are poorly known despite their relevancy. We estimate here the timing and extent of late Pleistocene–early Holocene glaciers in the Andes of Santiago (33°50′S) from geomorphological and geochronological analyses. Our observations evidence that a glacial stage occurred before the Last Glacial Maximum (ELGM) at the San Gabriel drift (1,300 m a.s.l.), dated as ∼46–36 ka. Glacial stages during the latest Pleistocene–early Holocene transition period, partially concomitant with the Antarctic Cold Reversal (ACR) and with the Younger Dryas (YD) chronozones, were identified and dated at La Engorda drift (2,450–2,570 m a.s.l.) at ∼15–10 ka. We propose that the San Gabriel drift represents a prolonged glacial advance driven by increased precipitation and cold conditions off central Chile during glacial times. In La Engorda drift, late glacial advances occurred associated with increased regional precipitation, in the context of a transition from humid to arid climate in central Chile, concomitantly with a general warming trend of sea surface temperatures offshore in the southeastern Pacific and with reduced austral summer insolation. The results support the sensitivity of the Andean mountain glaciers to precipitation and paleoclimate conditions, most possibly associated with periods of increased northward influence of the mid-latitude westerlies during glacial and late glacial times, in addition to the El Niño/Southern Oscillation (ENSO) impact since the mid-Holocene, driving late Quaternary glacier advances in central Chile. We estimate a maximum variation of ∼1,200 m in the position of the late Quaternary Equilibrium Line Altitude (ELA), inferred at ∼3,400 m and ∼3,600 m a.s.l. at the time of the San Gabriel and La Engorda drifts, respectively, with respect to its modern location close to 4,600 m a.s.l.
1 Introduction
Mountain glaciers in the Andes of central Chile are in a transitional situation between the influence of the southeastern Pacific Subtropical Anticyclone to the north and the seasonal influence of the mid-latitude west winds belt–the westerlies-to the south. Seasonal to millennial climate regime changes in the subtropical region of central and north-central Chile are mostly related to variations in the strengthening and position of the subtropical anticyclone, the influence of mid-latitude storms associated with the westerlies, and the ocean-climate variability associated with the El Niño/Southern Oscillation phenomenon (Rutllant and Fuenzalida, 1991; Jenny et al., 2002; 2003; Valero-Garcés et al., 2005; Vargas et al., 2006; Garreaud, 2007; Garreaud, 2013; Latorre et al., 2007; Ortega et al., 2012). As a result, central Chile experiences a strong latitudinal precipitation gradient from ∼87 mm/year at 30°S to more than 650 mm/year at 35°S. In addition, a zonal precipitation gradient is present due to orographic effects (McPhee et al., 2014), with the city of Santiago receiving an annual rainfall of about 345 mm/year, compared to more than 650 mm/year in the Andean mountains located to the east (Figure 1). Glaciers in this region strongly depend on snow events mostly during the austral winter season in the Andean watersheds and serve as temporary water reservoirs during the rest of the year and along multiannual drought periods (McPhee et al., 2014).
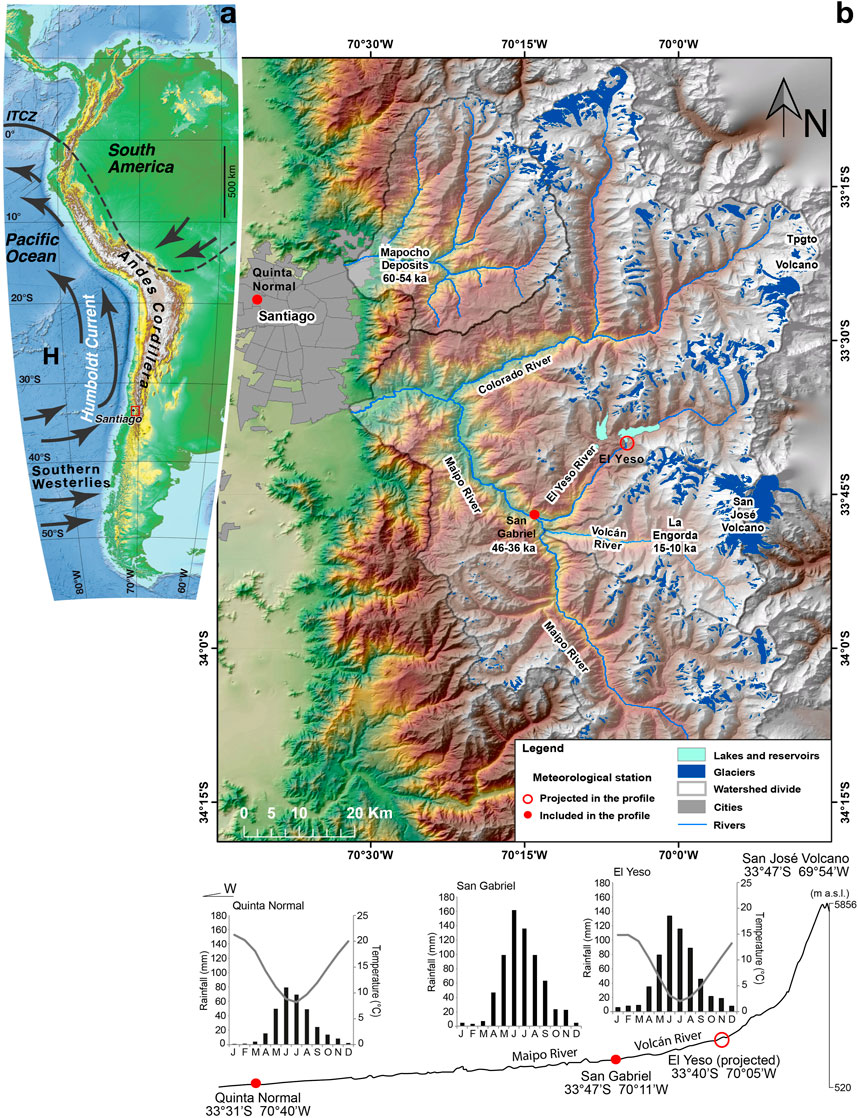
FIGURE 1. (A) Regional climatic and geomorphological context of the Maipo River Andean catchment and Mapocho River watershed in the Andes in front of Santiago, central Chile, showing the main atmospheric circulation patterns affecting western South America (black arrows), including the southern westerlies, the southeastern Pacific Subtropical Anticyclone (H), the Intertropical Convergence Zone (ITCZ), and the oceanic Humboldt Current System mostly transporting cold Subantarctic Waters northwards from higher latitudes. (B) Location of the Maipo River catchment and study sites in the Andes Cordillera in front of Santiago, showing a strong orographic precipitation gradient.
Climate trends in the Andes of central Chile reveal a strong east–west contrast between coastal cooling (−0.20°C/decade) and warming of the western and eastern slopes of the main Andean cordillera (+0.25°C/decade; Falvey and Garreaud, 2009). Even though regional precipitation between 30° and 34°S has not shown significant statistical variation during the last decades (Quintana and Aceituno, 2006), the general trend toward west–east differentiation in the behavior of the Equilibrium Line Altitude (ELA; Brenning, 2005; Carrasco et al., 2005) has been associated with a decrease in total precipitation and increased temperatures in high mountains (Cortés et al., 2012; McPhee et al., 2014). A trend toward rapid glacial mass loss was observed in the Andean glaciers during the last decades of the 20th century and the first 2 decades of the 21st century (Rivera et al., 2000; Dussaillant et al., 2019). Interannual variability in positive/negative precipitation anomalies and snow cover associated with warm/cold phases of the ENSO phenomenon (Rutllant and Fuenzalida, 1991) are superimposed on this negative multidecadal precipitation trend, which includes extensive megadrought in central Chile (Boisier et al., 2016).
A clear grasp of the relationship between major climate variations and glacier dynamics–advances and retreats-over long-term time scales is critical for improving our understanding of the response of subtropical mountain glaciers to global and regional climate changes. In the Andes of Santiago, this response has critical societal implications because the region is inhabited by more than 7 million people who depend upon snow cover and glaciers as reservoirs for their present and future water supply (McPhee et al., 2014; Figure 2). Despite this, knowledge on the chronology of Quaternary glaciations and glacier dynamics is scarce.
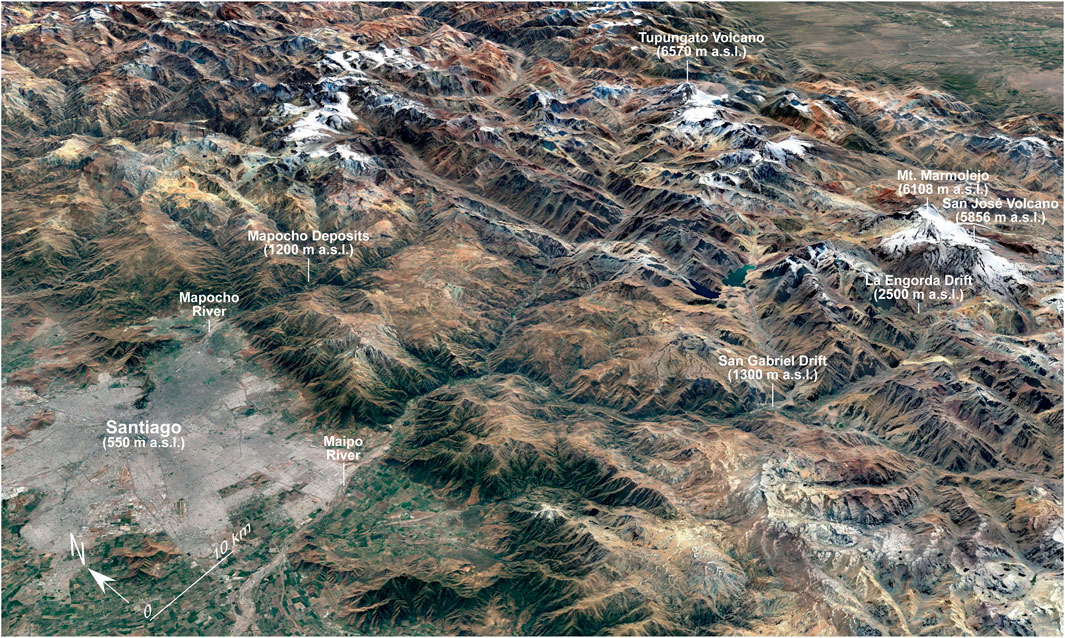
FIGURE 2. Google Earth oblique image view of the Andes Cordillera in front of Santiago, showing the location of the drifts -geomorphological glacial units-identified and dated in the Maipo River Andean watershed (La Engorda and San Gabriel drifts), together with the paraglacial deposits identified in the Mapocho River watershed.
Here, we provide geomorphological and chronological observations from the Maipo River Andean catchment in central Chile that constrains late Quaternary glacier dynamics in terms of major advances and retreats. We define two main drifts–geomorphologic glacial units-from which we interpret the glacial stages during the late Quaternary. Millennial-scale variations of glacier advances defined by the position of these drifts along this mountain valley are associated with paleoclimate conditions and changes in the position of the regional ELA.
2 Methods
2.1 Site and previous work
The Principal Cordillera in the Andes of Santiago is drained by the Maipo River and its tributary, the Mapocho River, whose watersheds reach up to more than 6,000 m a.s.l. (Figure 1). The Maipo River Andean catchment includes the tributaries of the Yeso River and Volcán River, where we focused our investigation due to the identification of a well-preserved geomorphological record as well as its preferential zonal orientation, suitable for a regional paleoclimate comparison (Figure 1).
The Volcán River catchment encompasses the mountain relief located from ∼1,200 m a.s.l. up to ∼6,100 m a.s.l. and between 33°40′-33°57′ S and 70°13′-69°50′ W. It is part of the Maipo River Andean watershed, bound on the east by the Volcán San José (5,856 m a.s.l.) and Cerro Marmolejo (6,108 m a.s.l.) peaks (Figures 1, 2). La Engorda drift, as defined in this work, is located at 2,450–2,570 m a.s.l. in the namesake river valley that directly drains the San José volcano (Figure 2). Downstream, the Volcán River flows from the highest mountains toward the confluence of three fluvial systems near the town of San Gabriel, located at 1,200 m a.s.l. There, the Maipo River, coming from the south, joins the Volcán and Yeso tributary rivers (Figure 1).
Although evidence for Quaternary glaciations in central Chile has been reported since the mid-20th century, chronological constraints for glacier advancements and paleoclimatic implications are scarce. Brüggen (1950), Borde (1966), and Chiu (1991) performed geomorphological mapping in the area, partially including sediments from Quaternary glaciations in the Andean watershed of the Maipo River. More recently, Ormeño (2007), Rauld (2011), and Herrera (2016) reported geomorphological records of late Quaternary glacial advances in the localities of San Gabriel and La Engorda in the Maipo River Andean watershed (Figure 1).
From a regional perspective, evidence of glacier advances in the subtropical western slope of the Andes from 10Be exposure ages in moraines has been documented from Cordón de Doña Rosa (∼30°S), simultaneous with the global LGM and at 43 ka (Zech et al., 2006; Zech et al., 2007; Zech et al., 2008; Zech et al., 2011; Zech et al., 2017), and at 20–18 ka, ∼33–25 ka, and ∼40 ka at the El Encierro River valley (∼29°S; Aguilar et al., 2022). At Laguna del Maule (∼36°S), Singer et al. (2000) concluded that the last ice retreat in the area started between 25.6 and 23.3 ka, partly concomitant with respect to the timing of the global LGM. At the Cachapoal River valley (∼34°S), glacier stages have been proposed from 10Be exposure ages in lateral moraines at 3.8 ka, 13.5 ka, and 21.9–20.3 ka (Charrier et al., 2019).
In the Mendoza River valley located on the eastern slope of the Andes at ∼32° 45′S, Espizúa (1993); Espizúa (1999) provided ages to constrain the occurrence of glacial stages. From U-series and 230Th/232Th ages on travertines overlying glacial geomorphological features, and from thermoluminescence (TL) dating of non-glacial sediments, Espizúa (1993); Espizúa (1999) estimated minimum ages of 38.3 ± 5.3–31.0 ± 3.1 ka and between 24.2 ± 2.0 and 22.8 ± 3.1 ka for travertines found in between two till deposits constituting the “Penitentes Drift”; of 15.0 ± 1.5 and 15.0 ± 2.1 ka for laminated and non-glaciated sediments overlying the “Horcones Drift,” considered roughly synchronous with the global LGM; and of 14 ka BP or 11–10 ka BP for the “Almacenes Drift”. Similarly, from radiocarbon dating of non-glaciated sediments in Valle Hermoso (−35°S), Espizúa and Bigazzi (1998) and Espizúa (2004) provided minimum ages for three tills named “Hermoso I, II and III”, located at 1970, 2,170, and 2,500 m a.s.l., respectively, apparently comparable with the oldest three tills described at Mendoza River valley, with ca. 15.9 ± 0.1 ka BP and 13.6 ± 0.1 ka BP as minimum ages for the “Hermoso II” and ca. 10.6 ± 0.1 ka BP as a minimum age for the “Hermoso III” tills. In addition, Espizúa (1993); Espizúa (1999) inferred younger advances described as neoglacial (“Confluencia Drift”; 3,300 m a.s.l.) in the Mendoza River valley.
2.2 Identification and characterization of glacial geomorphological units
The identification and mapping of the drifts defined here were performed using aerial photographs (1:50,000), LANDSAT (15 m pixel resolution) and SPOT (5 m pixel resolution) satellite images, a Digital Elevation Model (DEM, 15 m resolution), and extensive fieldwork supported by handheld GPS location, together with sedimentological and geochronological analyses.
We described geomorphological features located in paleo-ablation zones, specifically corresponding to terminal, frontal, and lateral moraines, and outwash terraces and lacustrine deposits, following the sedimentary facies analysis associated with glacial landforms to interpret the glacial advances and related morphodynamics (Church and Ryder, 1972; Edwards, 1986; Miller, 1996). The state of conservation of these morphologies is evidence that they have not been surpassed by any further glacial development (Edwards, 1986; Miller, 1996), and therefore, terminal moraines are considered to represent glacial advances during the last glaciation. Interpretation from facies analysis is included together with the description of each corresponding drift.
We have identified four drifts named San Gabriel, in the confluence of the Yeso, Volcán, and Maipo rivers, and La Engorda, Colina River, and Las Arenas, in the Volcán River catchment within the Andean watershed of the Maipo River (Figures 1, 2), providing geochronological constraints and detailed characterization for the first three of them. We have also identified and dated well-stratified fine-grained (silt and clay) and sandy layers that we interpret as paraglacial deposits located in the watershed of the Mapocho River (Figure 1). Geochronological analyses performed in this study include optically stimulated luminescence (OSL) dating from quartz grains in sediments (Supplementary Tables SA1−A3), cosmogenic 36Cl exposure dating from boulders on moraine surfaces (Supplementary Tables SB1−B4), and AMS 14C dating of organic sediment (Supplementary Table SD1). We have also collected samples for 10Be exposure dating, but the amount of quartz was not enough for the AMS analysis (Supplementary Table SC1), consistent with the dominancy of andesitic and basaltic volcanic rocks in the area.
2.3 Optically stimulated luminescence dating of sediments from San Gabriel drift
Geochronological determination of the San Gabriel drift was based upon OSL dating performed on sandy sediments interbedded between gravel- and boulder-rich layers, constituting an outwash terrace developed downstream from a terminal moraine cut by El Yeso River at San Gabriel (Figure 3; 4). A similar geochronological analysis was performed on well-sorted sandy sediments interpreted as paraglacial facies in the Mapocho River catchment (Figure 5), reflecting low-energy sedimentary environments that enhance solar resetting (e.g., Schaetzl and Forman, 2008).
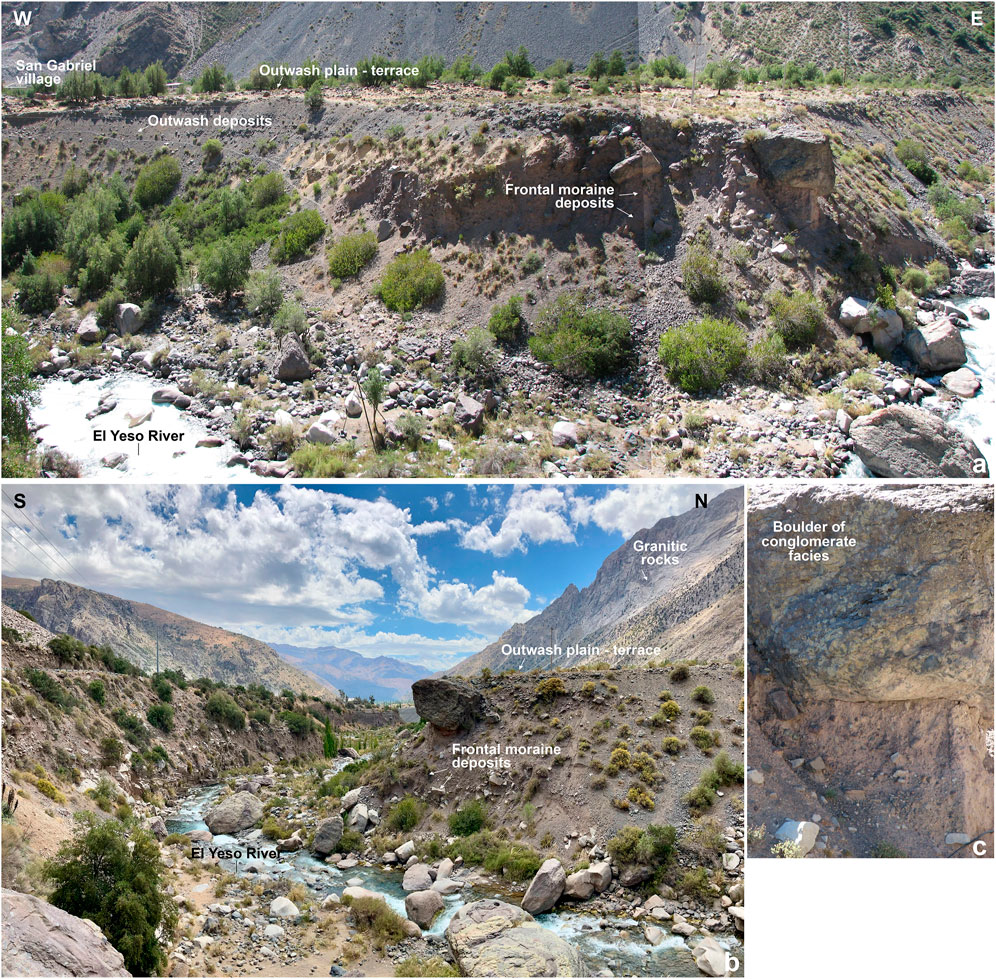
FIGURE 3. (A), (B) Panoramic view of a frontal moraine overlaid by glaciofluvial sediments forming a terrace in El Yeso River valley in the confluence area with the Maipo River at San Gabriel, highlighting outwash deposits with laminated sands sampled for OSL analyses (sample RM07-03; see Supplementary Tables SA1−A3). (C) Detail of a boulder of conglomerate facies (likely from the Jurassic Río Damas Formation, exposed around 20 km upstream in the valley; Thiele, 1980) immersed within a diamicton deposit interpreted as a till constituting the frontal moraine.
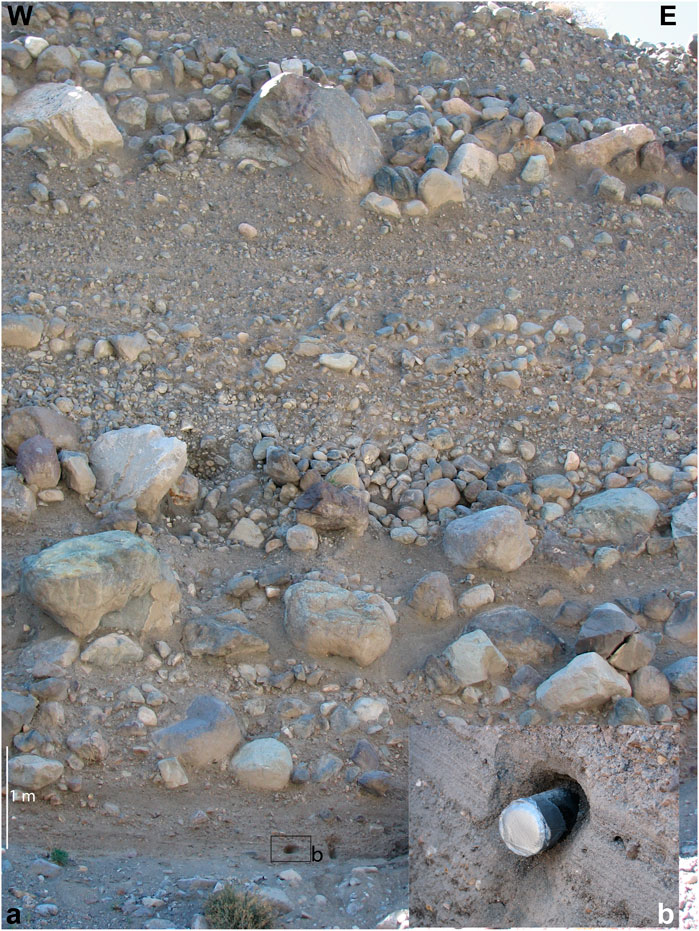
FIGURE 4. (A) Glaciofluvial sediments corresponding to the outwash terrace at San Gabriel near the confluence of El Yeso and Maipo rivers. (B) Laminated sands sampled for OSL analyses (samples RM07-01 and 02; see Supplementary Tables SA1−A3).
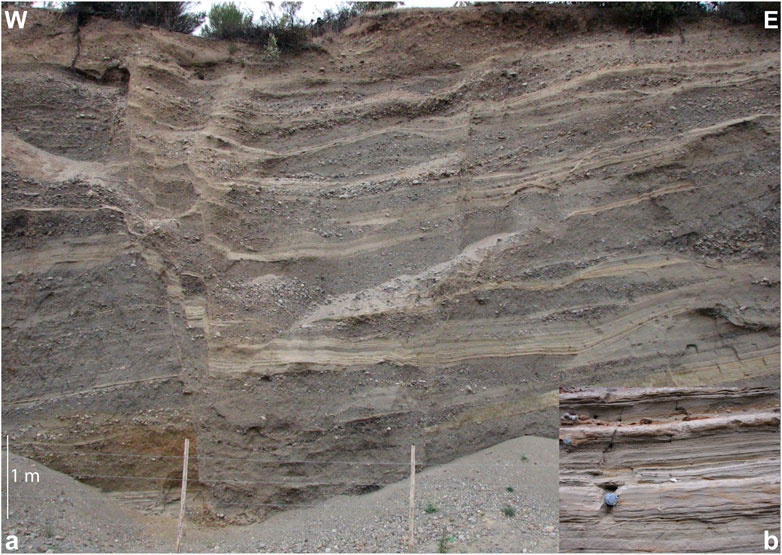
FIGURE 5. (A) Well-stratified sandy and fine (silt and clay) sediments interpreted as paraglacial facies at La Ermita in the Mapocho River catchment. (B) Deposits sampled for OSL analyses (samples RM07-15 and 16; see Supplementary Tables SA1−A3).
The sampling collection process for OSL dating required the insertion of tubes of 5 cm in diameter and approximately 10–15 cm in length in the sediment after the exposed outcrop had been removed. This ensured a minimum sample weight of 300 g. The sample extraction required the tube to be completely inserted into the sediment, a process that could be assisted by a rubber hammer. After extracting the tube with the sediments, the edges had to be sealed with opaque tape to ensure the protection of the sample from further light exposure. The outermost 1 cm of the sediment sampled in the tube was removed, leaving unexposed sediment in the center of the tube for optical dating. We choose the OSL method due to its advantage to obtain reliable age results from quartz- and/or feldspar-rich sediments formed in glacifluvial environments during the late Quaternary using single aliquot regeneration (SAR) and multiple-aliquot regeneration (MAR) protocols (Annex A; Supplementary Tables SA1−A3).
2.4 La Engorda drift 36Cl cosmogenic geochronology boulders dating and radiocarbon dating
Geochronological determinations on morphostratigraphic units at La Engorda area were obtained by cosmogenic 36Cl exposure, dating from the top of boulders located at the surface of ice-contact moraines (Supplementary Tables SB1−B4), and 14C, dating from organic material from sediments overlaying or embedded within the moraines (Supplementary Table SD1).
Sampled boulders were characterized by a low degree of weathering, with flat and isolated surfaces located on mounds corresponding to terminal moraines and in an intermorainic zone (Figure 6). The main lithology of the boulders corresponded to breccia and conglomerates in the sandy matrix (Figure 7), most possibly associated with the Jurassic Río Damas Formation (Thiele, 1980). A hammer and chisel were used to extract about 1 kg of the most superficial layer (∼5 cm) from each boulder, with the aim of maximizing the recovery of cosmogenic isotopes. A detailed explanation of the methodology is provided in the Supplementary Material (Annex B).
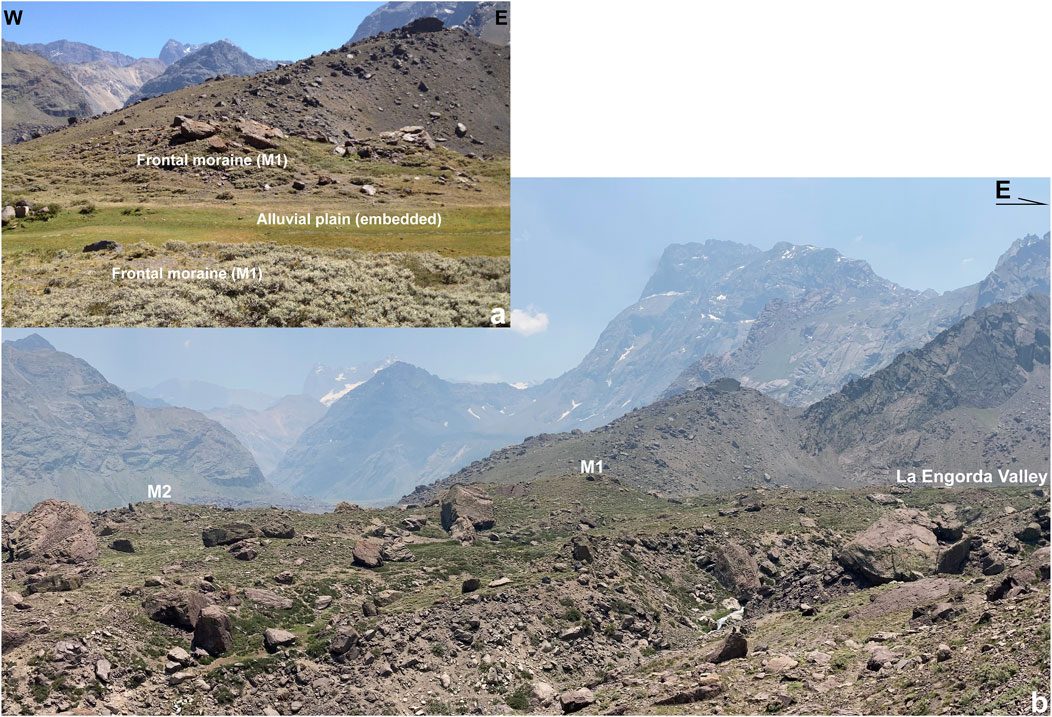
FIGURE 6. (A), (B) View of moraine M1 and panoramic view of frontal moraine system at La Engorda valley, showing moraines M1 and M2 together with an intermorainic depression in between, with boulders on top of the moraines, sampled for 36Cl cosmogenic analyses.
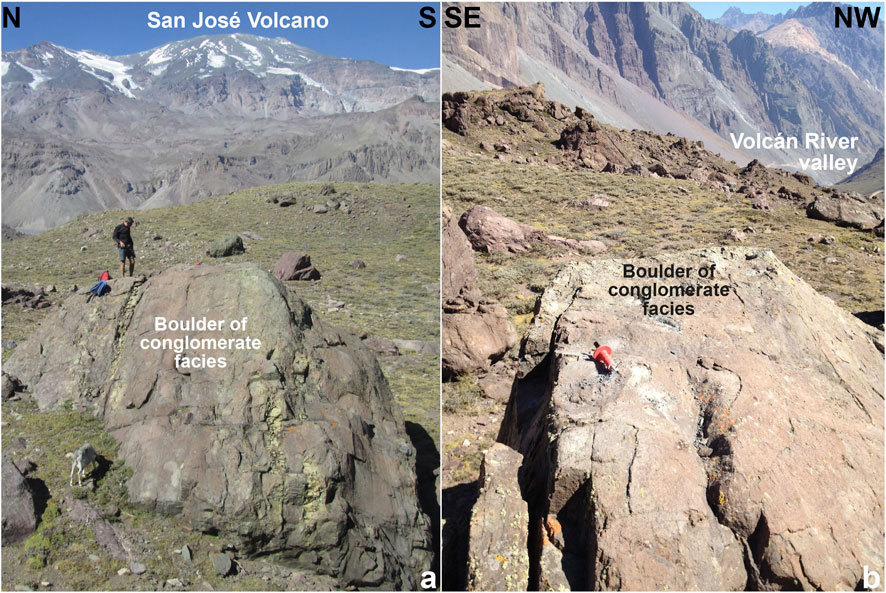
FIGURE 7. Views toward the east (A) and toward the SW (B) of boulders of conglomerate facies sampled from frontal moraines at La Engorda valley for 36Cl cosmogenic analyses.
Radiocarbon dating was performed on bulk sedimentary organic matter obtained from the samples collected from the layers overlaying the moraines, which are interpreted to represent the average age of disseminated carbon within each sample. Samples were acid-washed to remove secondary carbonate and pretreated to eliminate labile organic matter to analyze the more resistant organic material. Conventional radiocarbon results from bulk organic matter in acidified sediments were then calibrated using Calib Radiocarbon Calibration Program (Stuiver and Reimer, 1993; Stuiver et al., 2005), and SHCal20 atmospheric database (Hogg et al., 2020). We report a 2σ calibrated age range together with the corresponding highest relative probability (Supplementary Table SD1).
Samples were extracted from stratigraphic sections observed in pits and dug from the surface of both terminal moraines M1 and M2 (Figure 6) and from the surface of the alluvial plain composed of glaciofluvial and glaciolacustrine sediments inset in these moraines at La Engorda. These radiocarbon ages are considered as minimum ages for the stabilization of the landform.
3 Results
3.1 San Gabriel drift and Mapocho deposits
The San Gabriel drift is composed of remains of lateral and frontal moraines located at the confluence of the Maipo, Volcán, and Yeso rivers, together with an extensive outwash terrace developed downstream from this last area (Figure 8). Alluvial fans, alluvial plains, colluvium, and landslides overlay these last features. Fluvial terraces and modern rivers are inset within glacial units.
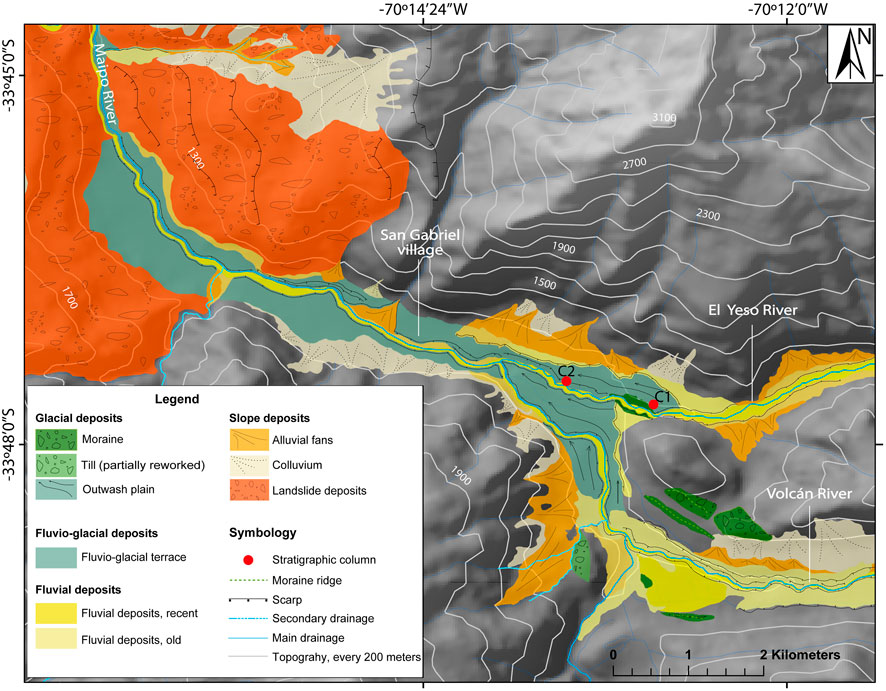
FIGURE 8. Geomorphological map of the San Gabriel drift, showing the location of stratigraphic sections (C1 and C2), characterizing the outwash terrace.
Lateral moraines are well exposed around the confluence between the Maipo and Volcán rivers, reaching altitudes of ∼1,370–1,500 m a.s.l. In the Volcán River, distal lateral moraines of east–west orientation are located on both sides of the valley close to the confluence with the Maipo River. These moraines are composed of boulders and angular gravels containing granitoids as a dominant -but not exclusive-lithology, most probably of the San Gabriel stock (Thiele, 1980), immersed within a sandy and fine-grained matrix. The moraines appear to be well preserved from erosive fluvial processes and exhibit a characteristic elongated form (Figure 8). In the confluence area of the Volcán and Maipo rivers, moraine remnants are located on the western side of the Maipo River valley, overlaying the rock substratum and reaching ∼1,400 m a.s.l. Other diamictons associated with the frontal position of the Quaternary glaciers can be recognized as remnants of frontal or bottom moraines eroded by the Volcán and Maipo rivers.
In the Yeso River valley, 2 km upstream of the confluence with the Maipo River, remains of an eroded frontal moraine area located close to the modern Yeso River at an altitude of 1,300 m a.s.l. There, a polymictic diamicton is exposed. The deposit is composed of boulders and angular–subangular gravel within a sandy and fine matrix, including boulders of breccia and conglomerate of 5–10 m in diameter that are most likely associated with the Río Damas Formation (Thiele, 1980), whose outcrops are located more than 20 km upstream in this valley (Figures 3, 8). Downstream of this moraine, an outwash terrace can be morphostratigraphically correlated with the same kind of surfaces developed at the confluence of the Volcán and Maipo rivers (Figure 8).
Outwash terraces are located more than 20 m above the thalweg of the rivers. These are composed of stratified deposits of boulders and are well rounded to subrounded gravels within the coarse and fine sandy matrix, exhibiting well-developed reverse-normal grain size grading and incipient lamination (Figure 4). These sedimentary facies can be interpreted as a product of hyperconcentrated flows produced by floods associated with ice melting in a proglacial environment. Outwash terrace deposits are normally related to episodes or pulses of glacial meltwater discharge, which generate sediment discharge that alters the fluvial bed load (Church and Ryder, 1972; Edwards, 1986; Miller, 1996).
Three OSL ages were obtained from the laminar sands located near the base and close to the top of the sediments constituting the outwash terrace in El Yeso River, close to the confluence with the Volcán and upper Maipo rivers (Supplementary Tables SA1−A3). An age of 46.0 ± 4.7 ka was obtained from the sediments in section C1, located just above the terminal moraine (Figure 9). Ages of 44.7 ± 3.7 ka and 36.4 ± 3.0 ka were obtained from the sediments in section C2, located approximately 1.5 km downstream (Figure 9), suggesting progradation of the outwash plain downstream since the frontal moraine was deposited (Figure 8). We interpret these ages as the occurrence of fluctuating melting of glaciers between ∼46 and ∼36 ka, defining an early local Last Glacial Maximum stage (ELGM) before the global Last Glacial Maximum (LGM; Clark et al., 2009; Hughes, 2021).
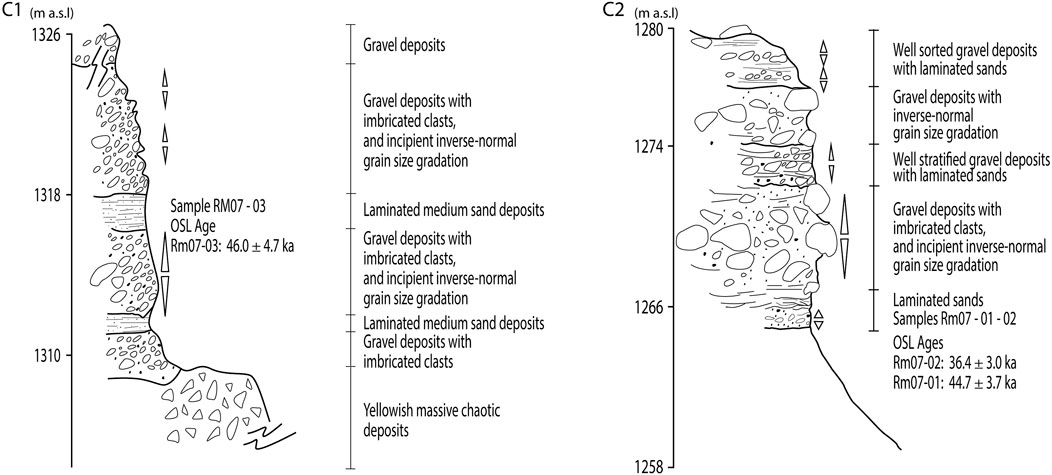
FIGURE 9. Stratigraphic sections C1 and C2 from the outwash terrace at San Gabriel drift (see Figure 8 for location) and OSL age results obtained from fine sandy laminated facies.
We interpret the San Gabriel drift as resulting from glacial advances during the Late Pleistocene. Once the glaciers reached their maximum advance within the Maipo, Volcán, and Yeso river valleys, which is registered by the lateral and frontal terminal moraines in this area (Figure 8), glacial melting began, providing meltwater that transported debris coming from the moraines. This process was aided by subglacial and supra- and intraglacial flow, as well as from the adjacent slopes, providing discharge that contributed to the formation of fluvial terraces downstream and upstream when the glaciers rapidly retreat (Edwards, 1986; Miller, 1996; Bennett and Glasser, 2009). This can explain the conspicuous terraces that developed downstream and upstream of the frontal moraine in the Maipo, Yeso, and Volcán rivers in the San Gabriel area (Figure 8).
In the Mapocho River catchment, well-stratified sandy and fine sediments are located at an altitude of approximately 1,200 m a.s.l., preserved on a terrace above the modern river thalweg (Figures 2, 5). These sedimentary facies are completely different to those of the modern deposits in the river and ravines in the area, mostly composed of gravel and coarse-to-medium sand facies. No moraines can be recognized in the area, possibly due to erosion. The position of this terrace, along with the well-stratified fine material that constitutes it and the occurrence of collapse features in the sedimentary package, suggest a paraglacial or proglacial environment (Church and Ryder, 1972). Two OSL ages from these sediments yielded 60.4 ± 5.1 and 53.9 ± 5.0 ka, suggesting glacial activity during MIS4 (Supplementary Tables SA1−A3), i.e., before the glacier advances documented in this study for the San Gabriel drift in the Maipo River Andean catchment.
3.2 La Engorda drift
La Engorda drift is composed of N-S-oriented frontal moraines named M1 and M2, which are located at 2,570 and 2,450 m a.s.l. for the inner and outer ridges, respectively, with an inboard outwash plain and alluvium overlying glaciolacustrine sediments (Figures 6, 10). Alluvial fans, glaciofluvial plains, colluvium, and landslides overlay glacial landforms in the area (Figure 10). The modern river is inset in the moraines, showing a relatively recent change in the local base level due to the rupturing of the natural dam composed of the latter deposits (Figure 11).
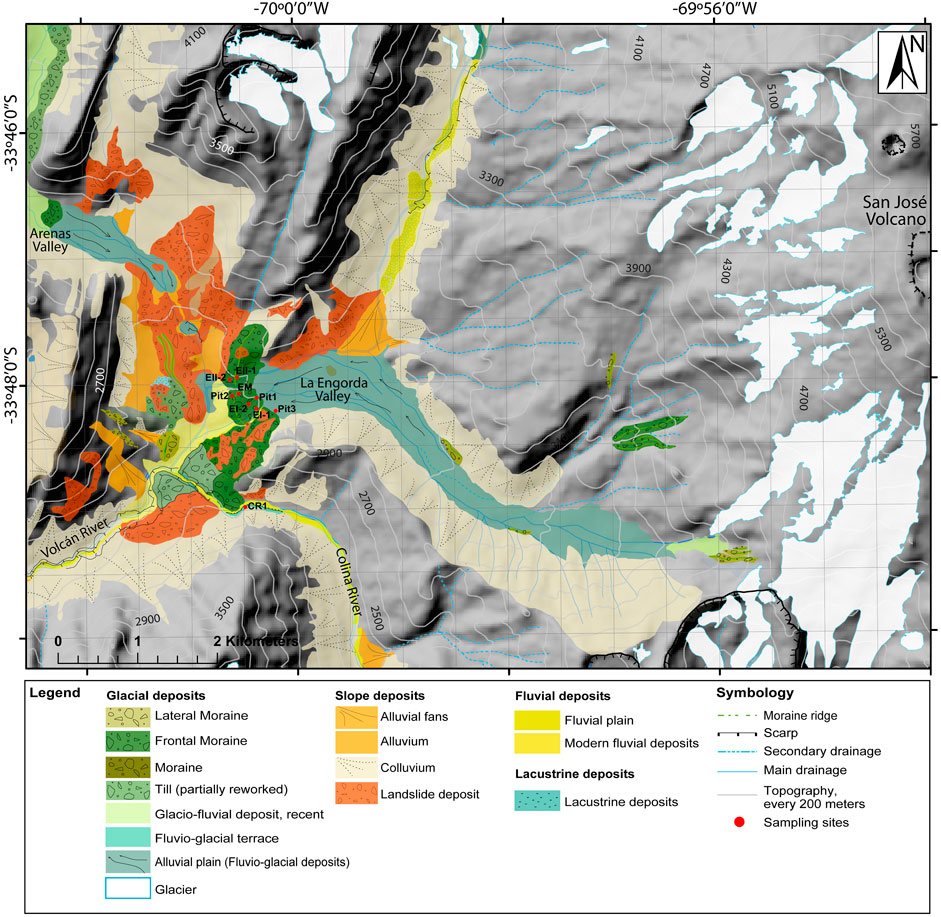
FIGURE 10. Geomorphological map of La Engorda valley and surrounding area, showing the location of frontal moraines and sampling sites.
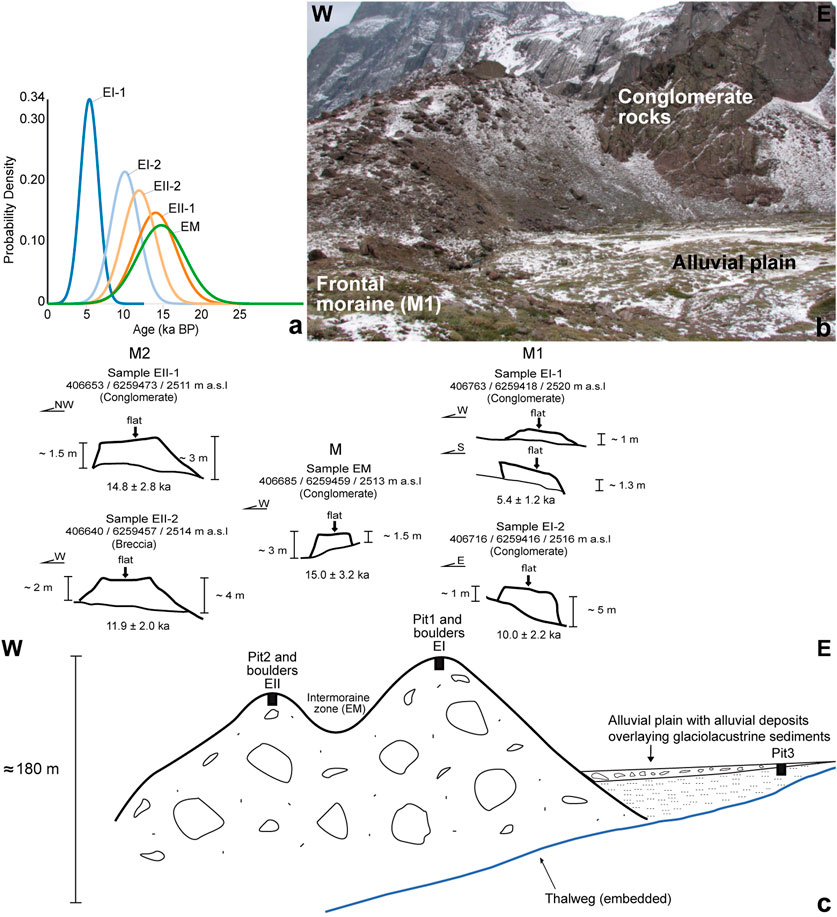
FIGURE 11. (A–C) Schematic geomorphologic profile and sampling sites from La Engorda drift in the Volcán River catchment within the Maipo Andean watershed (see Figure 6). The location of boulders on top of moraines M1 and M2 (B,C), dated sampled for 36Cl exposure dating (A), is shown, along with the location of pits excavated for radiocarbon sampling (C).
Frontal moraines reach approximately 180 m in height from the river thalweg, crossing the western limit of La Engorda valley perpendicularly (Figure 11). The inner ridge -M1- corresponds to a well-defined hill located to the east of the terminal moraine system, and the outer ridge -M2- corresponds to a less defined hill that is nonetheless easily observable in the field to the west of the same moraine system (Figure 6).
Moraines at La Engorda are composed of massive diamictons interpreted as till facies, with angular and rounded clasts of variable sizes ranging from some centimeters to large boulders several meters in size, embedded within a fine-to-medium sandy matrix that forms more than 50% of the deposits. Polymictic clasts are chaotically distributed within the deposit. Among these, sedimentary rocks predominate, mainly conglomerates and sandstones from the Río Damas Formation (Thiele, 1980), together with volcanic breccia associated with igneous material from the nearby San José volcano.
Exposure 36Cl dating from boulders yielded ages of 14.8 ± 2.8 ka and 11.9 ± 2.0 ka for those coming from the outer -M2- ridge, 15.0 ± 3.2 ka from the one sampled in the intermoraine depression (M), and 10.0 ± 2.2 ka and 5.4 ± 1.2 ka in the case of those sampled on top of the inner–M1- ridge (Figure 11; Supplementary Tables SB1−B4). We interpret most boulders as eroded and transported rapidly during late glacial advances. The recorded boulder ages fall within an interval spanning the Antarctic Cold Reversal (ACR) and Younger Dryas (YD) chronozones and into the early Holocene. The spread in ages for most of the ∼15–10 ka set is like the spread observed when cosmogenic dating is applied to late Holocene glacier advances after taking into consideration the large uncertainty values (e.g., Fernández-Navarro et al., 2023). Radiocarbon dating of inboard paludal, fluvial, and alluvial sediments onlapping the moraine ridge to the east do not record sediment older than ∼10 ka at the base (Pits 1, 2, and 3; Figure 12; 13), being consistent with the boulders ages, except for the youngest. The younger boulder age (∼5.4 ka) can be attributed to re-exposure of the surface of the boulder, potentially covered by loose sediment, to a rockfall associated with nearby rockslides (Chiu, 1991) or to a Holocene glacial readvance; further research is needed to elucidate these possibilities.
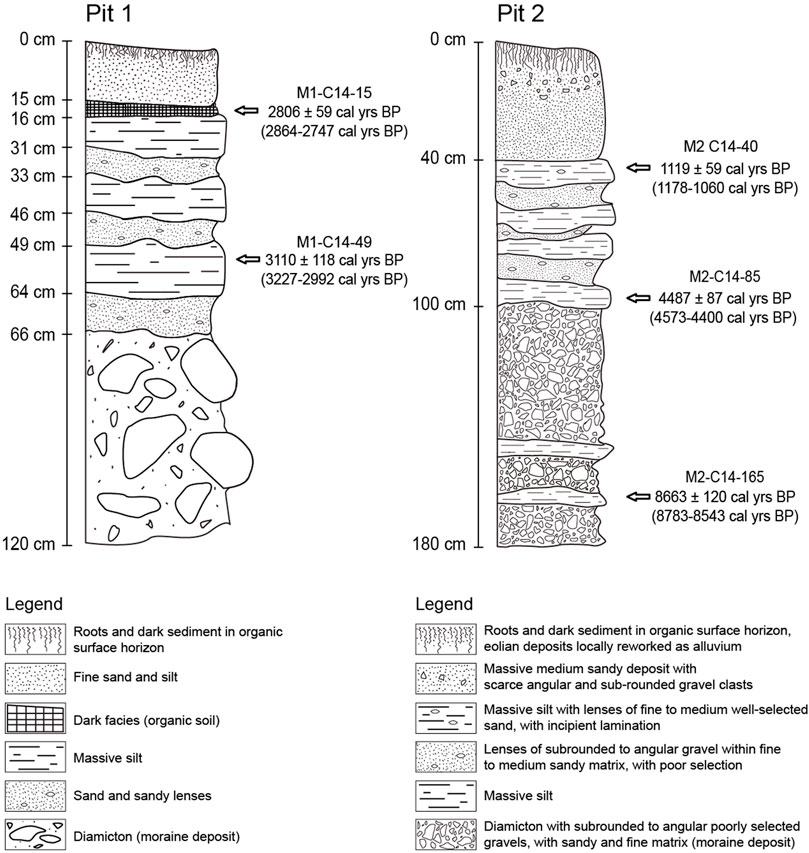
FIGURE 12. Stratigraphic sections from the surface of moraines M1 and M2 at La Engorda Drift. The normal grain size gradation from the diamicton at the base toward sandy and fine sediments with organic horizons on top is shown, which is consistent with the local low-energy alluvial reworking of eolian material. Radiocarbon age results are reported associated with the corresponding sampled strata.
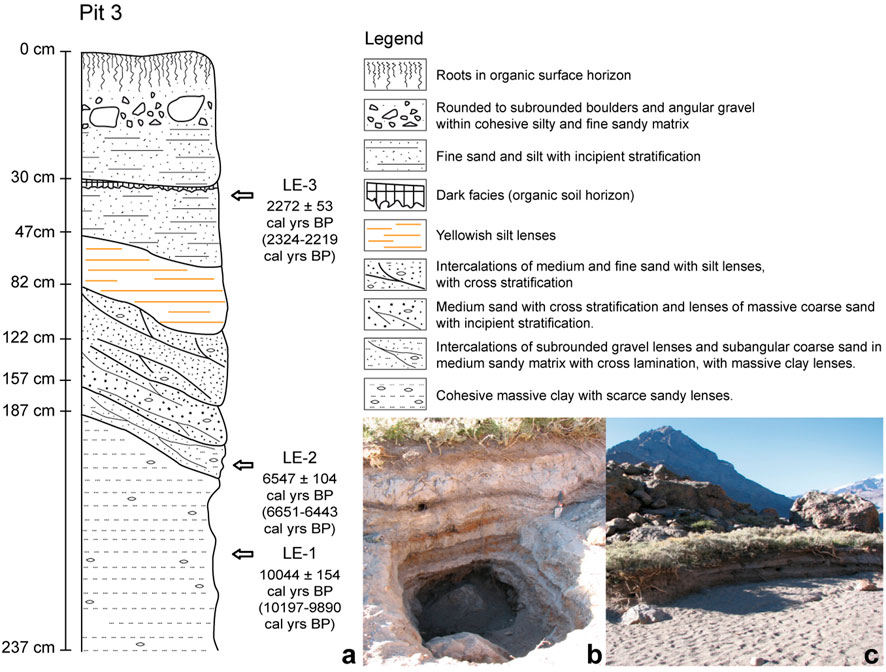
FIGURE 13. (A) Stratigraphic section of glaciolacustrine cohesive massive silt and clay overlaid by sandy lenses with organic rich horizons and alluvial sediments on top, onlapping the frontal moraine M1 at La Engorda drift. Radiocarbon age results are reported in association with the corresponding sampled strata (Pit 3). (B), (C) Photos showing the stratigraphy and local geomorphological context of Pit 3 at La Engorda drift.
We considered the possibility that all the boulders could be simply exhumed global LGM blocks that, at some point, were buried under a large lake. All dated boulders, however, are located on ridges at least 100 m above the floor of the valleys to the west and south. It is improbable that the boulders were exhumed after being covered by a large lake covering several km2, which should have extended towards the southwest. There are no remains associated with a drainage obstacle or lacustrine sediments suggesting a lake with these dimensions existed at some point between the LGM and ∼10 ka. Only at the junction with the Colina River, about 1.5 km south of the M1 and M2 moraines on the south hillslope of the valley, an outcrop of lacustrine sediments suggests a short blockage of the Colina fluvial system, probably associated to a frontal moraine during the early LGM (Figure 14), which was later partially covered by rockslides coming from the south and the east (Chiu, 1991). This early moraine probably never reached the elevation of the ridges (more than 100 m above this point). Radiocarbon ages obtained from these lacustrine facies overlaying a diamicton deposit at this site yielded ages between 32.5 and 35.8 cal ka BP (Figure 14), which are comparable with a radiocarbon age of 24.5 ± 0.4 ka BP (28.7 ± 0.9 cal ka BP), previously obtained at the base of these lacustrine sediments by Moreno et al. (1991).
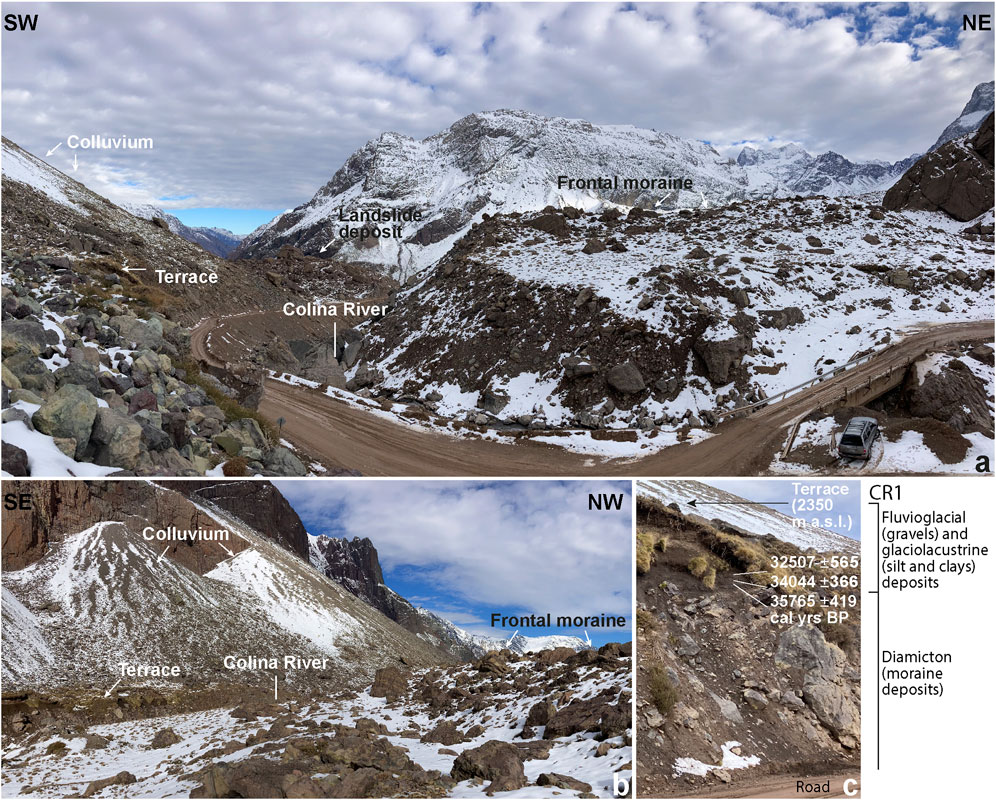
FIGURE 14. (A), (B) Geomorphological context of a diamicton deposit interpreted as a till constituting a frontal moraine in the Colina River valley, with a hanging terrace composed of glacio-fluvial sediments overlain by colluvium. (C) Lacustrine sediments overlying the diamicton deposit, from which radiocarbon ages between 32.5 and 35.8 cal ka BP were obtained (see location in Figure 10).
The surface of the ridges associated with the main body of each moraine is partially overlain by sandy sediments interstratified with well-sorted fine deposits exhibiting incipient lamination, which we interpret as the local reworking of material by alluvial and eolian processes at the surface of the moraines. Dark organic-rich layers and soil development are observed on top (Figure 12). All these sediments are younger than ∼8.7 cal ka BP and as recent as ∼2.8–1.1 cal ka BP, demonstrating the Holocene evolution of the surface of these moraines following the last ice retreat in this area, as suggested by the cosmogenic dates (Figures 11, 12). The lack of an obvious buried LGM surface below these Holocene sediments is consistent with the idea of only late glacial advances recorded at this elevation in the catchment, even after considering a potential lag of organic material deposition at this elevation after the LGM (Figure 11).
After deposition of the frontal moraines and initial ice retreat up the valley, a proglacial lake developed, evidenced by glaciolacustrine sediments situated below the younger glaciofluvial and alluvial material constituting the outwash plain inset, inboard the moraines (sensu Edwards, 1986; Miller, 1996; Figure 11). Glaciolacustrine deposits correspond to massive cohesive mud with interstratified incipiently laminated sandy facies. A radiocarbon date obtained at the base of the massive glaciolacustrine sediments, in this position of the landscape, yielded an age of 10.2 ± 0.2 cal ka BP (Figure 13), which is consistent with the ∼10 ka cosmogenic age determination obtained from a boulder on top of the inner moraine ridge (Figure 11). Two radiocarbon ages obtained from bulk sedimentary organic matter from fine sandy layers and organic horizons, interpreted as glacio-fluvial and alluvial sediments discordantly overlying the massive glaciolacustrine deposits, yielded ages of 6.5 ± 0.1 cal ka BP and 2.3 ± 0.5 cal ka BP, respectively, suggesting the prevalence of a proglacial environment during most of the Holocene (Figures 11, 13).
Modern fluvial and alluvial sediments inset in the moraines are composed of rounded, sub-rounded, and angular boulders, gravel, and pebbles arranged chaotically in a sandy and fine cohesive matrix, with some of these deposits exhibiting inverse-normal grain size gradation that can be interpreted as bed load and debris flow deposits, different from those constituting the outwash plain (Figure 11).
4 Discssion
4.1 Paleoclimate implications from San Gabriel drift
Modern glaciers in the Andes in front of Santiago are located at altitudes above 3,200 m a.s.l. in the Volcán, Yeso, and Maipo rivers watersheds (Figure 1). These glaciers are located more than 30 km to the east from the San Gabriel drift (Figures 1, 2). We interpret the San Gabriel drift moraines as a frontal moraine system, which includes lateral moraines marking the maximum altitude reached by the late Quaternary glacial tongues at that place (∼1,500 m a.s.l.), while the location of the frontal moraines determines the lowest position of the glaciers coming through the three river valleys (∼1,300 m a.s.l.). Because glacial deposits have not been observed downstream, we interpret the frontal moraines in the San Gabriel area as a terminal system, which is the largest preserved glacial advance during the late Quaternary within the Maipo Andean catchment (Figures 1, 2). This occurred between ∼46 and 36 ka according to the OSL ages obtained from outwash sediments in this drift (Figure 9), which suggest an early local Last Glacial Maximum (ELGM) in the region.
As previously shown (Figure 5), well-stratified silty and sandy sediments interpreted as paraglacial facies and dated as ∼60–54 ka are located at ∼1,200 m a.s.l. in the Mapocho River valley, at a similar altitude to the San Gabriel drift. Since no lateral or frontal moraines have been observed in this zone, additional evidence is needed to rule out the origin of these deposits to other processes, such as, for example, an ephemeral dam produced by local landslides. If those sedimentary layers have a glacial origin as we propose, it could imply the occurrence of a MIS4 glacial advance in the Mapocho River catchment before any observed in the Maipo Andean catchment.
From the study of the sedimentary record at Laguna Tagua-Tagua, located in the central valley of central Chile (∼34°S), Valero-Garcés et al. (2005) suggested wet conditions during the last glaciation before 43.5 cal ka BP and between 40 and 21.5 cal ka BP, evidenced by increased lake levels and the presence of pollen corresponding to Valdivian rainforest taxa, which appears only south of 37°S during modern times. The period between 42.1 and 40.1 cal ka BP was characterized by relatively lower temperatures and decreased moisture conditions, concomitantly with a trend toward minimum summer insolation in the southern hemisphere (Valero-Garcés et al., 2005). Regional wet conditions are consistent with paleoceanographic interpretations from ocean sediment cores obtained off central and central-northern Chile (33°S and 27°S), which suggest more humid and cold conditions being responsible for the increased runoff between ∼46 and 36 cal ka BP (Lamy et al., 1998; Lamy et al., 1999; Lamy et al., 2000; Kim et al., 2002; Stuut and Lamy, 2004; Figure 15). Thus, a period of increased precipitation and cold conditions could explain an earlier advancement of the Andean mountain glaciers in the Maipo River catchment, previous to the global LGM, which occurred between ∼46 and 36 ka BP according to the San Gabriel drift record (Figure 5). This suggests glacier advances partly contemporaneous with the earlier development of the Patagonian glaciers during the late Quaternary (Denton et al., 1999; Zech et al., 2017; García et al., 2018; Davies et al., 2020).
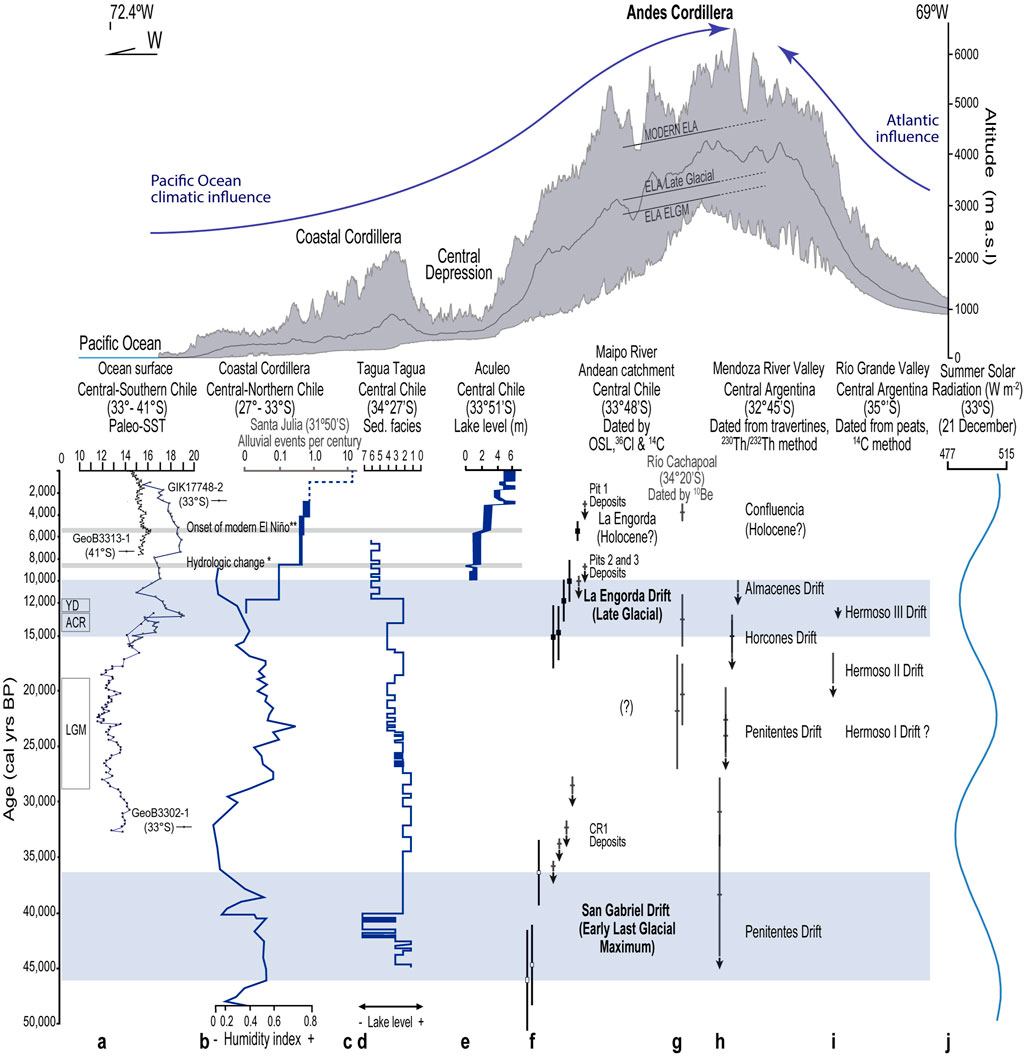
FIGURE 15. Swath profile showing the regional relief of the Andes at the latitude of Santiago valley, regional chronostratigraphic comparison between the drifts defined in this work at the Maipo Andean catchment, and late Pleistocene–Holocene glacier and paleoclimate records from central Chile and Argentina. (A) Paleo-SST reconstructions off central Chile from Kim et al. (2002). (B) Humidity index along the coast of central-northern Chile from Lamy et al. (1999); Lamy et al. (2000) and Stuut and Lamy (2004). (C) Paleoclimate record from alluvial episodes inferred from the Santa Julia site in central-northern Chile (Ortega et al., 2012). (D), (E) Lake level variations inferred from Tagua-Tagua and Aculeo records according to Valero-Garcés et al. (2005) and Jenny et al. (2002), respectively. (F) Late Quaternary glacial advances inferred from the San Gabriel and La Engorda drifts (this work). (G) Late Quaternary glacial advances inferred from geomorphological records of the Cachapoal River catchment. (H, I) Late Pleistocene and Holocene glacial advances on the eastern slope of the Andes from Espizúa (1999); Espizúa (2004); see Supplementary Table SD1, for calibrated radiocarbon ages from Hermoso II and III drifts. (J) Estimated summer insolation during the late Quaternary at 33°S. (*) Hydrologic change from Ortega et al. (2012). (**) Onset of modern ENSO tropical–extratropical climate teleconnections from Vargas et al. (2006). Modern Equilibrium Line Altitude (ELA) and paleo-ELA estimates at the time of the San Gabriel drift and La Engorda drift are also shown (this work). LGM: Last Glacial Maximum; ACR: Antarctic Cold Reversal; YD: Younger Dryas.
The age range determined from the San Gabriel drift is comparable with the 10Be cosmogenic age data of ∼50–33 ka obtained from glacial landforms in the Cordón de Doña Rosa, located at ∼30°S on the western slope of the Andes by Zech et al. (2007); Zech et al. (2008); recalculated in Zech et al., 2017; according to recent production rate data), and with the older ages of ∼35 and ∼40 ka reported in the El Encierro valley (∼29°S; recalculated from Zech et al., 2008, in Zech et al., 2017; Aguilar et al., 2022). In addition, the San Gabriel drift appears relatively contemporaneous to the Pleistocene glaciations in the Mendoza River and Río Grande catchments on the eastern slope of the Andes (32.45–35.30°S), corresponding to the older Penitentes and eventually Valle Hermoso I glacial units, respectively (Espizúa, 1993; Espizúa, 2004; Espizúa and Bigazzi, 1998; Figure 15), suggesting the zonal response of Andean mountain glaciers to regional climate changes during the Late Pleistocene at these latitudes.
It is possible that increased precipitation accompanied by cold conditions at the time of the San Gabriel drift would have been driven by a northward shift of the mid-latitude westerlies during glacial times, as previously suggested (Lamy et al., 1998; Lamy et al., 1999; Lamy et al., 2000; Stuut and Lamy, 2004; Valero- Garcés et al., 2005; Zech et al., 2007; Zech et al., 2008; Zech et al., 2011; Zech et al., 2017, 2018), which could also explain the older Penitentes drift, from which minimum ages of ∼38–31 ka BP have been reported in the Mendoza River valley (∼32° 45′S; Espizúa, 1993; Espizúa, 1999).
4.2 Paleoclimate implications from La Engorda drift
La Engorda drift is composed of two moraine ridges, which are located approximately 200–250 m above the Volcán River valley (Figure 10). This difference in altitude allows classifying this valley as a paleo-hanging glacier valley, since the paleoglacier of La Engorda was probably a tributary at the time of this drift and most possibly not directly connected with the main glacier filling the valley of the Volcán River.
Our geochronological data from La Engorda drift suggest two main pulses of glacial advance during late glacial times. Moraines M1 and M2 record ages between ∼15 and ∼10 ka, with a younger (single) dated boulder on the innermost, eastern edge of this drift and adjacent to the outwash-alluvial plain (Figure 11). The two younger ages in the older set of the drift fall, within 1-sigma, into the Younger Dryas (YD) chronozone, a millennial-duration cold period that punctuated the last termination between 12.9 and 11.7 ka (Broecker et al., 2010; Sweatman, 2021), and the early Holocene. In addition, our data suggest a local readvance of the glaciers in the area concomitantly with the ACR, and there are late glacial advances reported from the Patagonian Andes (Sagredo et al., 2011; Sagredo et al., 2018; Strelin et al., 2011; García et al., 2012; García et al., 2014; Reynhout et al., 2019; Davies et al., 2020). Radiocarbon ages obtained from glaciolacustrine deposits onlapping the younger moraine are close to ∼10.0 cal ka BP (minimum ages), consistent with the age inferred for this inner moraine using our cosmogenic dataset and supporting the late glacial advances at ∼15–10 ka followed by a local ice retreat afterward. The presence of fine eolian sediments locally reworked by alluvial processes on top of the moraines (Figures 11, 12) is also consistent with a local glacial advance during the late glacial advances at this locality.
Our data do not record any drift associated with the global LGM in the region, contrasting with other records such as the Cachapoal (Charrier et al., 2019) and the Encierro Valley, Cordón Doña Rosa, and Las Leñas valley (Zech et al., 2017). Between the San Gabriel and La Engorda drifts, a glacial advance that can possibly be traced to the LGM might be recorded at the confluence area of the Volcán River valley with Las Arenas valley (Figure 10). Sediments from this advance appear to be mostly covered by a large rockslide. A potential glacial advance during the global LGM in central Chile could have occurred in synchrony with generally wet regional conditions, inferred from the Laguna Tagua-Tagua sedimentary record, which is in the central valley south of the study region at 34°30′S (Valero-Garcés et al., 2005; Figure 15). The above-mentioned advance would have also occurred concomitantly with higher coastal humidity along central-northern Chile and with cold sea surface temperatures (SSTs) prevailing in the southeastern Pacific during the LGM off central Chile until ∼18 ka (Lamy et al., 1998; Lamy et al., 1999; Lamy et al., 2000; Kim et al., 2002; Stuut and Lamy, 2004; Figure 13).
Glacier advances recorded at La Engorda drift between ∼15 and 10 ka occurred concomitantly with a change in regional climate from humid to arid conditions at the late Pleistocene–Holocene transition, as inferred from the Tagua-Tagua record and the Aculeo record, located also in the central valley at 33°50′S (Valero-Garcés et al., 2005; Jenny et al., 2002; Jenny et al., 2003; Figure 15). The Tagua-Tagua record in central Chile suggests a period of increased precipitation between 13.5 and 11.5 cal ka BP, most possibly due to the northward influence of mid-latitude westerlies at that time, followed by the most arid period -inferred for the last 46 ka-during the early and mid-Holocene (Valero-Garcés et al., 2005), which is consistent with the extremely arid to arid conditions prevailing since the beginning of the Holocene until 5.7 cal ka BP, as inferred from the Aculeo record (Jenny et al., 2002). Thus, it is possible that the intensified influence of the mid-latitude westerlies would have driven increased precipitation under relatively cold conditions in central Chile, favoring positive mass balance and glacier advances at La Engorda during ∼15–10 ka, partly concomitantly with the ACR and YD periods. This happened in the context of a general warming trend of SSTs off central Chile during the latest Pleistocene, followed by relatively low SSTs during the early Holocene (Kim et al., 2002; Figure 15). Low coastal paleo-SSTs have been inferred from isotope composition of mollusk shells in northern Chile during the late Pleistocene–early Holocene transition (23°S and 25°S; Vargas et al., 2006; Flores et al., 2018). Meanwhile, similar to modern coastal paleo-SSTs have been inferred from mollusk shells during the early Holocene in central-northern Chile (31°S; Carré et al., 2012), suggesting a strong latitudinal gradient and reinforced atmospheric circulation associated with the southeastern Pacific Subtropical Anticyclone consistent with La Niña-like conditions at that time (Vargas et al., 2006), which would have favored increased aridity during the early Holocene in central Chile.
Non-glacial sediments stratigraphically located between the Horcones and Almacenes drift deposits on the eastern slope of the Andes yield U-series and 230Th/232Th ages of 15.0 ± 1.5 and 15.0 ± 2.1 ka (Espizúa, 1993; Espizúa, 1999). This is interpreted as a non-glacial interval during this period, which indicates that the Horcones drift deposits would correspond to a glacial advance that occurred prior to ∼15 ka. The Almacenes moraines would be associated with glacier advancements that occurred after ∼15 ka (Espizúa, 1993; Espizúa, 1999). In the Río Grande valley (∼35°S), radiocarbon ages from peats in a swamp located within the limits of the moraine system of Valle Hermoso II yield minimum values of ∼15.9–13.6 ka BP (i.e., ∼19.2–16.3 cal ka BP; Espizúa and Bigazzi, 1998; Espizúa, 2004; Supplementary Table SD1). A minimum radiocarbon age of ca. 10.6 ka BP (i.e., ∼12.4 cal ka BP; Supplementary Table SD1) was obtained from the Hermoso III tills in the Río Grande valley (Espizúa and Bigazzi, 1998; Espizúa, 2004). Although further research is needed on both sides of the Andes to increase the precision of the geochronological data, the comparison between our findings from the western slope, with previous data from the eastern slope of the Andes in central Chile and Argentina, supports the generally consistent regional trends in glacier advances during the latest Pleistocene–early Holocene transition period (Figure 15).
La Engorda drift is the first reported evidence of regional manifestations for a readvance of mountain glaciers at a time interval spanning the ACR and YD chronozones, and the early Holocene, in the Andes of Santiago and in central Chile. In the Cachapoal River catchment (∼34°20′S), located immediately south of the Maipo Andean watershed, the boulders on top of the lateral moraines exposed to 10Be exposure ages from boulders on top of lateral moraines show values of 20.3 ± 2.9 ka, 21.9 ± 5.3 ka, and one date of 13.5 ± 2.4 ka (Charrier et al., 2019). Although none of the ages in our study record the global LGM, deposits potentially associated with it might be covered with rockslide sediments just downstream of La Engorda. If this is the case, frontal moraines ridges concomitant with the global LGM at this latitude might have reached 2000 m a.s.l. in the Volcán River catchment in the Maipo Andean watershed, while in the Cachapoal River catchment, lateral moraines with similar ages are located at ∼950 m a.s.l. (Charrier et al., 2019). This sharp change in the altitudinal location of the moraines in neighboring Andean catchments could imply a strong latitudinal gradient in the precipitation pattern between ∼33.5°S and ∼34.33°S on the western slope of the Andes in central Chile at the time of the global LGM. Additional research is needed to confirm this inference. The presence of Valdivian rainforest taxa in the corresponding chronostratigraphic horizons in the Laguna Tagua-Tagua record supports the inference of increased precipitation in the central valley at ∼34.5°S concomitantly with the global LGM period (Valero-Garcés et al., 2005), while conspicuous eolian sediments dated between ∼21.6 and 17.3 ka in a paleoseismological trench in the west Andean piedmont would suggest arid conditions in addition to aeolian sediment supply from distal or abandoned alluvial systems in the Santiago valley at that time (Vargas et al., 2014). On the contrary, the similar altitudinal location of the frontal moraine dated as ∼15–10 ka at La Engorda in the Maipo Andean watershed (2,570 m a.s.l.), compared to the altitudinal location of the lateral moraine of similar age in the Cachapoal catchment (2,700 m a.s.l.), supports the idea of similar factors driving readvances of mountain glaciers at that time in the Andes of central Chile. This effect was probably driven by the intensified northward influence of the mid-latitude westerlies during the latest Pleistocene, as previously proposed for glacier advances concomitantly with the ACR period, followed by more variable conditions during the YD chronozone at Patagonia (Sagredo et al., 2018).
Glacier advances during the ELGM and the LGM, reported here in the Andean catchment of the Maipo River and in the Cachapoal River catchment (Charrier et al., 2019), respectively, occurred concomitantly with relatively higher austral summer insolation (Figure 15), with low SSTs prevailing offshore in the Southeastern Pacific (Kim et al., 2002), and during globally persistent cold conditions, as revealed by ice cores from Antarctica (Petit et al., 1999; Labeyrie et al., 2003; Villalba et al., 2009). On the contrary, the glacier advances during late glacial times in central Chile occurred concomitantly with lower austral summer insolation in the context of a general warming trend of SSTs in the Southeastern Pacific (Kim et al., 2002; Figure 15). In both scenarios, increased precipitation seems to have played a driving role for glacier advances in the Andean catchments in central Chile, as in modern times (McPhee et al., 2014). In this last sense, if a mid-to-late Holocene glacier advance is confirmed, as suggested by the youngest boulder dated from La Engorda drift and by Cachapoal catchment data (Charrier et al., 2019; Figure 15), it would be interesting to compare these mid-Holocene glacier advances with the onset of modern El Niño (Rodbell et al., 1999) and modern El Niño tropical–extratropical teleconnection patterns (Vargas et al., 2006; Figure 15), which include episodes of increased precipitation in central Chile (Rutllant and Fuenzalida, 1991).
4.3 Paleo-equilibrium line altitude estimations
Paleo-ELA estimates in the Andes are extremely scarce. On the eastern slope of the subtropical Andean mountains in Argentina, (Espizúa, 1993; Espizúa, 2004), estimated the altitude of the modern ELA at ∼4,500 m a.s.l. Using the accumulation–area ratio (AAR) method (Benn and Gemmell, 1997), the depression in the elevation of the ELA has been estimated at 1,000, 1,200, and 1,250 m a.s.l. at the time of Horcones, Penitentes, and Punta de Vacas drift, respectively (Espizúa, 1993; Espizúa, 1999). Altitudes of ∼3,250 ± 50 and 3,350 ± 50 m a.s.l. have been estimated for the modern ELA in the case of the Azufre and El Peñón glaciers (∼35°S).
The study of paleo-ELAs can be carried out through the application of different methods that consider hypsometric differences between glaciers. The AAR method assumes that the accumulation area of a glacier encompasses a fixed proportion of the total glacier area (Benn and Gemmell, 1997). This method requires the mapping of the extent of paleo-glaciers by means of geomorphological observations (Porter, 1981). Observations of the variability of modern ELAs on the western slope of the Andes suggest that this altitude strongly depends on the amount of snowfall during the austral winter (Masiokas et al., 2006; Garreaud et al., 2008). Precipitation during the cold austral season is therefore a key factor for the development of glacial mountain systems in central Chile.
Estimating the ELA of an ancient glacier using any morphometric method provides results for the glacier in steady state (whereby the balance state of the paleo-ELA is similar to the current ELA), which is associated with a particular climatic condition (Osmaston, 2005). According to the AAR method (Porter, 1975; Porter, 1981; Porter, 2001; Benn and Gemmell, 1997), and considering an accumulation area/total glacier area ratio of 0.6 ± 0.1 with the aim of characterizing glacier valley conditions on a global scale (Singh et al., 2011), we estimated the location of the modern ELA in the study region close to ∼4,600 m a.s.l. ELA estimates inferred from atmospheric (temperature and precipitation) variables for the Andes of central Chile (between 33°-34°S), for the period of 1958–2018, show mean values in the range of about 3,700–4,300 m a.s.l., with a significant increasing trend in the first 2 decades of the 21st century (Barria et al., 2019). Through the AAR method (with a similar ratio of 0.6 ± 0.1), we estimate that the ELA would have been situated close to 3,400 m a.s.l. and 3,600 m a.s.l. at the time of San Gabriel and La Engorda drifts, i.e., ∼1,200 m and ∼1,000 m lower than their current positions in the region, respectively.
For comparison, we also estimated the paleo-ELA using the area–altitude balance ratio (AABR) method, which is most suitable for white, snow-fed glaciers (Benn and Lehmkuhl, 2000; Osmaston, 2005). This method should not be applied to glaciers that receive massive avalanche inputs, where mass balances are non-linear or where there is extensive debris cover, as in the case of the Andean glaciers studied here. The application of this method shows ELA values estimated between ∼3,100 and ∼3,000 m a.s.l., in the case of the San Gabriel drift and between ∼3,600 and ∼3,500 m a.s.l. for La Engorda drift (Herrera, 2016).
5 Conclusion
Detailed geological mapping and geochronology of glacial landforms allowed the definition of two drifts representing the late Quaternary glacial stages in the Maipo Andean watershed, which is located on the western slope of the Andes in central Chile.
The San Gabriel drift is located at 1,300 m a.s.l., and it is composed of lateral and terminal moraines situated at the confluence of the Maipo River with the Volcán and Yeso tributary rivers, along with an extensive outwash terrace. Considering the OSL ages of ∼46–36 ka obtained from the glaciofluvial deposits constituting the outwash terrace, this drift would represent a glacial advance before the global Last Glacial Maximum. In accordance with regional paleoclimate and paleoceanographic records, this occurred concomitantly with increased precipitation and cold conditions favored by cold sea surface temperatures off central Chile during glacial times. Sediments interpreted as paraglacial facies in the neighboring Mapocho River catchment, located just to the north of the Maipo River watershed at an altitude of 1,200 m a.s.l., were dated as old at ∼60–54 ka, suggesting the occurrence of glacier advances early during the last glaciation before the Last Glacial Maximum in the region.
La Engorda drift, in the Volcán River catchment, is composed of frontal moraines with two ridges at 2,450–2,570 m a.s.l., with an outwash plain composed of glaciofluvial deposits and alluvium overlying glaciolacustrine sediments onlapping the moraines. Based on the 36Cl exposure ages from boulders and the radiocarbon data from lacustrine and glaciofluvial sediments, we interpret late glacial advances from this drift between ∼15 and 10 ka, concomitantly with the ACR and the YD chronozones and with the early Holocene. A glacial stage at that time was possibly associated with increased precipitation in the context of a climate transition from humid to arid conditions in central Chile, concomitantly with a general warming trend of SSTs offshore in the southeastern Pacific and with reduced austral summer insolation at this latitude.
When compared with regional-scale records from both the eastern and western sides of the Andes, these novel results illustrate the sensitivity of subtropical mountain glaciers to ocean-climate changes.
The results support a maximum variation of about 1,200 m in the position of the Equilibrium Line Altitude (ELA) during the late Quaternary glacier advances evidenced here, inferred at ∼3,400 and ∼3,600 m a.s.l. at the time of the San Gabriel and La Engorda drifts, respectively, with respect to its modern location at ∼4,600 m a.s.l. Once compared with records at a regional scale on both sides of the Andes, these novel results evidence the sensitivity of subtropical mountain glaciers to ocean-climate changes.
Data availability statement
The original contributions presented in the study are included in the article/Supplementary Material, further inquiries can be directed to the corresponding authors.
Author contributions
All the authors MH-O, GE, JA, and SF contributed to the research, field work, sampling, interpretation, and writing of the manuscript. GE: Research strategy and funding. MH-O: Research, PhD Thesis. JA: 36Cl cosmogenic age determinations. SF: OSL age determinations. All authors contributed to the article and approved the submitted version.
Funding
This work was supported by the Department of Geology and CEGA (Andean Geothermal Centre), University of Chile, and by the Chilean Science Council (ANID) through the Program Anillo (ACT210080). The first author has benefited from a PhD grant from CEGA and, presently, from a postdoctoral grant from the Anillo ACT210080.
Acknowledgments
We thank Antonio Ormeño, Rodrigo Rauld, Sergio Sepúlveda, Christian Nievas, Sebastian Carretier, Angelo Villalobos, José González-Alfaro, and Iván Aracena and his family for their field support, laboratory support, and useful suggestions. Antonio Ormeño was a brilliant student who conducted his MSc. thesis at the University of Chile on the Quaternary Geology of the Maipo River, including the geological mapping and stratigraphy of the San Gabriel drift. We remember him as a committed geologist, kind person, and dear friend. Susan Zimmerman and Alan Hidy (Center for Accelerator Mass Spectrometry, CAMS, at Lawrence Livermore National Laboratory) aided with cosmogenic Cl-36 analysis at CAMS and the interpretation of data. Julie Johnson and Elizabeth Huenupi (Desert Research Institute) prepared the cosmogenic Cl-36 target AgCl from the rock samples. We acknowledge the reviewers for useful suggestions and the editor Dr. Joseph Licciardi for handling this manuscript.
Conflict of interest
The authors declare that the research was conducted in the absence of any commercial or financial relationships that could be construed as a potential conflict of interest.
Publisher’s note
All claims expressed in this article are solely those of the authors and do not necessarily represent those of their affiliated organizations, or those of the publisher, the editors and the reviewers. Any product that may be evaluated in this article, or claim that may be made by its manufacturer, is not guaranteed or endorsed by the publisher.
Supplementary material
The Supplementary Material for this article can be found online at: https://www.frontiersin.org/articles/10.3389/feart.2023.1192812/full#supplementary-material
References
Aguilar, G., Riquelme, R., Lohse, P., Cabré, A., and García, J. L. (2022). Chronology of glacial advances and deglaciation in the Encierro River Valley (29° lat. S), southern atacama desert, based on geomorphological mapping and cosmogenic 10Be exposure ages. Front. Earth Sci. 10, 878318. doi:10.3389/feart.2022.878318
Barria, I., Carrasco, J., Casassa, G., and Barria, P. (2019). Simulation of long-term changes of the equilibrium line altitude in the central Chilean andes mountains derived from atmospheric variables during the 1958–2018 period. Front. Environ. Sci. 7, 161. doi:10.3389/fenvs.2019.00161
Benn, D. I., and Gemmell, A. M. D. (1997), Calculating equilibrium line altitudes of former glaciers by the balance ratio method: A New computer spreadsheet. Glacial Geology and geomorphology. Available at: http://ggg.qub.ac.uk/papers/full/1997/tn011997/tn01.html.
Benn, D. I., and Lehmkuhl, F. (2000). Mass balance and equilibrium line altitudes of glaciers in high mountain environments. Quat. Int. 65/66, 15–29. doi:10.1016/S1040-6182(99)00034-8
Bennett, M., and Glasser, N. (2009). Glacial Geology, ice sheets and landforms. Second Edition. Wiley- Blackwell, 400.
Boisier, J. P., Rondanelli, R., Garreaud, R. D., and Muñoz, F. (2016). Anthropogenic and natural contributions to the Southeast Pacific precipitation decline and recent megadrought in central Chile. Geophys. Res. Lett. 43, 413–421. doi:10.1002/2015GL067265
Borde, J. (1966). Les Andes de Santiago et leur avant pays: etude de géomorphologie. Bordeaux, France: Union Franc. d‟ Impress, 559.
Brenning, A. (2005). Combining statistical modelling and field Mapping. Humboldt Universitätzu Berlin: Dissertation Mathematisch-NaturwissenschaftlicheFakultät II. Climatic and geomorphological controls of rock glaciers in the Andes of Central Chile.
Broecker, W. S., Denton, G. H., Edwards, L., Cheng, H., Alley, R. B., and Putnam, A. E. (2010). Putting the Younger Dryas cold event into context. Quat. Sci. Rev. 29, 1078–1081. doi:10.1016/j.quascirev.2010.02.019
Carrasco, J. F., Casassa, G., and Quintana, J. (2005). Changes of the 0°C isotherm and the equilibrium line altitude in central Chile during the last quarter of the 20th century. Hydrological Sci. J. 50 (6), 933–948. doi:10.1623/hysj.2005.50.6.933
Carré, M., Azzoug, M., Bentaleb, I., Chase, B. M., Fontugne, M., Jackson, D., et al. (2012). Mid–holocene mean climate in the south–eastern pacific and its influence on south America. Quat. Int. 253, 55–66. doi:10.1016/j.quaint.2011.02.004
Charrier, R., Iturrizaga, L., Carretier, S., and Regard, V. (2019). Geomorphologic and glacial evolution of the cachapoal and southern Maipo catchments in the andean principal cordillera, Central Chile (34°-35°S). Andean Geol. 46 (2), 240–278. doi:10.5027/andgeoV46n2-3108
Chiu, D. (1991). Geología del relleno Cuaternario de las hoyas de los ríos Yeso, Volcán y Maipo, este último entre las localidades de Guayacán y Los Queltehues, Región Metropolitana. Santiago: Memoria de título de Geólogo, Departamento de Geología, Universidad de Chile, 111.
Church, M., and Ryder, J. M. (1972). Paraglacial sedimentation: A consideration of fluvial processes conditioned by glaciation. Geol. Soc. Am. Bull. 83, 3059–3072. doi:10.1130/0016-7606(1972)83[3059:psacof]2.0.co;2
Clark, P. U., Dyke, A. S., Shakun, J. D., Carlson, A. E., Clark, J., Wohlfarth, B., et al. (2009). The last glacial maximum. Science 325, 710–714. doi:10.1126/science.1172873
Cortés, G., Schaller, S., Rojas, M., Garcia, L., Descalzi, A., Vargas, L., et al. (2012). UFZ-Report 03/2012. Leipzig: Helmholtz Center for Environmental Research UFZ. Assessment of the current climate and expected climate changes in the Metropolitan Region of Santiago de Chile.
Davies, B., Darvill, Ch.M., Lowell, H., Bendle, J. M., Dowdeswell, J. A., Fabel, D., et al. (2020). The evolution of the Patagonian Ice Sheet from 35 ka to the present day (PATICE). Earth-Science Rev. 204, 103152. doi:10.1016/j.earscirev.2020.103152
Denton, G. H., Lowell, T. V., Heusser, C. J., Schlchter, C., Andersen, B. G., Heusser, L. E., et al. (1999). Geomorphology, stratigraphy, and radiocarbon chronology of llanquihue drift in the area of the southern lake district, seno reloncaví, and isla Grande de Chiloé, Chile. Geogr. Ann. 81, 167–229. doi:10.1111/j.0435-3676.1999.00057.x
Dussaillant, I., Berthier, E., Brun, F., Masiokas, M., Hugonnet, R., Favier, V., et al. (2019). Two decades of glacier mass loss along the Andes. Nat. Geosci. 12, 802–808. doi:10.1038/s41561-019-0432-5
Edwards, M. B. (1986). “Glacial environmments,” in Sedimentary environmments and facies. Editor H. G. Reading (Blackwell Scientific Publications), 445–470.
Espizúa, L. (1999). Chronology of late pleistocene glacier advances in the Rı́o Mendoza valley, Argentina. Glob. Planet. Change 22, 193–200. doi:10.1016/s0921-8181(99)00036-3
Espizúa, L., and Bigazzi, G. (1998). Fission-track dating of the punta de vacas glaciation in the Rı́o Mendoza valley, Argentina. Quat. Sci. Rev. 17, 755–760. doi:10.1016/s0277-3791(97)00056-5
Espizúa, L. (2004). “Pleistocene glaciations in the Mendoza andes, Argentina,” in Quaternary glaciations: extent and chronology. Part III: south America, asia, africa, australasia, Antarctica. Editors J. Ehlers, and P. Gibbard (Cambridge, MA: Elsevier), 69–73.
Espizúa, L. (1993). Quaternary glaciations in the Río Mendoza valley, Argentine andes. Quat. Res. 40, 150–162. doi:10.1006/qres.1993.1067
Falvey, M., and Garreaud, R. (2009). Regional cooling in a warming world: recent temperature trends in the southeast Pacific and along the west coast of subtropical South America (1979–2006). J. Geophys. Res. 114, D04102–D04116. doi:10.1029/2008JD010519
Fernández-Navarro, H., García, J. L., Nussbaumer, S. U., Tikhomirov, D., Pérez, F., Gärtner-Roer, I., et al. (2023). Fluctuations of the universidad glacier in the andes of central Chile (34° S) during the latest Holocene derived from a 10Be moraine chronology. Quat. Sci. Rev. 300, 107884. doi:10.1016/j.quascirev.2022.107884
Flores, C., Gayo, E. M., Salazar, D., and Boritman, B. R. (2018). δ18O of Fissurella maxima as a proxy for reconstructing Early Holocene sea surface temperatures in the coastal Atacama desert (25°S). Palaeogeogr. Palaeoclimatol. Palaeoecol. 499, 22–34. doi:10.1016/j.palaeo.2018.03.031
Garreaud, R. (2007). Precipitation and circulation covariability in the extratropics. J. Clim. 20, 4789–4797. doi:10.1175/JCLI4257.1
García, J. L., Hall, B. L., Kaplan, M. R., Vega, R. M., and Strelin, J. A. (2014). Glacial geomorphology of the torres del paine region (southern Patagonia): implications for glaciation, deglaciation and paleolake history. Geomorphology 204, 599–616. doi:10.1016/j.geomorph.2013.08.036
García, J. L., Hein, A. S., Binnie, S. A., Gómez, G., González, M. A., and Dunai, T. J. (2018). The MIS3 maximum of the Torres del Paine and Última Esperanza ice lobes in Patagonia and the pacing of southern mountain glaciation. Quat. Sci. Rev. 185, 9–26. doi:10.1016/j.quascirev.2018.01.013
García, J. L., Kaplan, M. R., Hall, B. L., Schaefer, J. M., Vega, R. M., Schwartz, R., et al. (2012). Glacier expansion in southern Patagonia throughout the Antarctic cold reversal. Geology 40 (9), 859–862. doi:10.1130/g33164.1
Garreaud, R. D., Vuille, M., Compagnucci, R., and Marengo, J. (2008). Present-day south American climate. Palaeogeogr. Palaeoclimatol. Palaeoecol. 281, 180–195. doi:10.1016/j.palaeo.2007.10.032
Garreaud, R. (2013). Warm winter storms in Central Chile. J. Clim. 14, 1515–1534. doi:10.1175/JHM-D-12-0135.1
Herrera, M. (2016). Estimación de las altitudes de las líneas de equilibrio en glaciares de montaña para el último ciclo glacial-interglacial en Los Andes de Santiago, Chile central. Tesis de Doctorado en Ciencias mención Geología. Santiago: Departamento de Geología, Universidad de Chile, 172.
Hogg, A. G., Heaton, T. J., Hua, Q., Palmer, J. G., Turney, C. S. M., Southon, J., et al. (2020). SHCal20 Southern Hemisphere calibration, 0-55,000 years cal BP. Radiocarbon 62, 759–778. doi:10.1017/RDC.2020.59
Hughes, P. D. (2021). “Concept and global context of the glacial landforms from the Last Glacial Maximum,” in European glacial landscapes: maximum extent of glaciations. Editors D. Palacios, P. D. Hughes, J. M. García-Ruiz, and N. Andrés (Elsevier), 355–358. doi:10.1016/B978-0-12-823498-3.00039-X
Jenny, B., Valero-Garcés, B. L., Villa-Martinez, R., Urrutia, R., Geyh, M. A., and Veit, H. (2002). Early to mid-holocene aridity in Central Chile and the southern westerlies: the Laguna Aculeo record (34°S). Quat. Res. 58, 160–170. doi:10.1006/qres.2002.2370
Jenny, B., Wilhelm, D., and Valero-Garcés, B. L. (2003). The Southern Westerlies in Central Chile: holocene precipitation estimates based on a water balance model for Laguna Aculeo (33°50′S). Clim. Dyn. 20, 269–280. doi:10.1007/s00382-002-0267-3
Kim, J. H., Schneider, R., Hebbeln, D., Müller, P. J., and Wefer, G. (2002). Last deglacial sea-surface temperature evolution in the Southeast Pacific compared to climate changes on the South American continent. Quat. Sci. Rev. 21, 2085–2097. doi:10.1016/s0277-3791(02)00012-4
Labeyrie, L., Cole, J., Alverson, K., and Stocker, T. (2003). The history of climate dynamics in the late quaternary. In Paleoclimate, global change and the futures (K. Alverson, R. Bradley, and T. Pedersen, Eds.), Ed. Springer.
Lamy, F., Hebbeln, D., and Wefer, G. (1999). High-resolution marine record of climatic change in mid-latitude Chile during the last 28,000 years based on terrigenous sediment parameters. Quat. Res. 51, 83–93. doi:10.1006/qres.1998.2010
Lamy, F., Hebbeln, D., and Wefer, G. (1998). Terrigenous sediment supply along the Chilean continental margin: modern regional patterns of texture and composition. Geol. Rundsch 87, 477–494. doi:10.1007/s005310050223
Lamy, F., Klump, J., Hebbeln, D., and Wefer, G. (2000). Late Quaternary rapid climate change in northern Chile. Terra nova. 12 (1), 8–13. doi:10.1046/j.1365-3121.2000.00265.x
Latorre, C., Moreno, P. I., Vargas, G., Maldonado, A., Villa-Martínez, R., Armesto, J. J., et al. (2007). “Late Quaternary environments and palaeoclimate,” in The Geology of Chile (Geological Society of London), 309–328.
Masiokas, M., Villalba, R., Luckman, B., Le Quesne, C., and Aravena, J. (2006). Snowpack variations in the central andes of Argentina and Chile, 1951–2005: large-scale atmospheric influences and implications for water resources in the region. J. Clim. 19, 6334–6352. doi:10.1175/jcli3969.1
McPhee, J., Cortés, G., Rojas, M., García, L., Delscalzi, A., and Vargas, L. (2014). “Downscaling climate changes for Santiago: what effects can be expected?,” in Climate adaptation Santiago. Editors K. Krellenberg, and B. Hansjurgens (Springer-Verlag), 19–41. doi:10.1007/978-3-642-39103-3_2
Miller, J. M. G. (1996). “Glacial sediments,” in Sedimentary environments: processes, facies and stratigraphy. Editor H. G. Reading (Blackwell Science), 454–484.
Moreno, H., Varela, J., and Thiele, R. (1991). Estudio Geológico y de Riesgos Volcánico y de Remoción en Masa del Proyecto Hidroeléctrico Alfalfal II y Las Lajas (Technical Report). Santiago: CHILGENER, S.A., 78.
Ormeño, A. (2007). Geodinámica de la hoya hidrográfica del río Maipo en la zona cordillerana de la Región Metropolitana: implicancias neotectónicas. Tesis de Magister en Ciencias mención Geología. Santiago: Departamento de Geología, Universidad de Chile, 177.
Ortega, C., Vargas, G., Rutllant, J. A., Jackson, D., and Méndez, C. (2012). Major hydrological regime change along the semiarid western coast of South America during the early Holocene. Quat. Reaserch 78, 513–527. doi:10.1016/j.yqres.2012.08.002
Osmaston, H. (2005). Estimates of glacier equilibrium line altitudes by the Area×Altitude, the Area×Altitude balance ratio and the Area×Altitude balance index methods and their validation. Quat. Int. 138, 22–31. doi:10.1016/j.quaint.2005.02.004
Petit, J. R., Jouzel, J., Raynaud, D., Barkov, N. I., Barnola, J.-M., Basile, I., et al. (1999). Climate and atmospheric history of the past 420000 years from the Vostok ice core, Antarctica. Nature 399, 429–436. doi:10.1038/20859
Porter, S. C. (1975). Equilibrium-line altitudes of late quaternary glaciers in the southern alps, New Zealand. Quat. Res. 5, 27–47. doi:10.1016/0033-5894(75)90047-2
Porter, S. C. (1981). “Glaciological evidence of Holocene climatic change,” in Climate and history. Editors T. M. L. Wigley, M. J. Ingram, and G. Farmer (Cambridge: Cambridge University Press), 82–110.
Porter, S. C. (2001). Snowline depression in the tropics during the last Glaciation. Quat. Sci. Rev. 20, 1067–1091. doi:10.1016/s0277-3791(00)00178-5
Quintana, J., and Aceituno, P. (2006). “Trends and interdecadal variability of rainfall in Chile,” in Proceedings of the 8th ICSHMO, Foz do Iguacu, 371–372.24-28
Rauld, R. (2011), Deformación Cortical y Peligro Sísmico Asociado a la Falla San Ramón en el frente Cordillerano de Santiago, Chile Central (33°S). Tesis de Doctor en Ciencias mención Geología. Santiago: Departamento de Geología, Universidad de Chile, 445.
Reynhout, S. A., Sagredo, E. A., Kaplan, M. R., Aravena, J. C., Martini, M. A., Moreno, P. I., et al. (2019). Holocene glacier fluctuations in Patagonia are modulated by summer insolation intensity and paced by Southern Annular Mode-like variability. Quat. Sci. Rev. 220, 178–187. doi:10.1016/j.quascirev.2019.05.029
Rivera, A., Casassa, G., Acuñaa, C., and Lange, H. (2000). Variaciones recientes de glaciares en Chile. Rev. Investig. Geográficas 34, 29–60. doi:10.5354/0719-5370.2000.27709
Rodbell, D. T., Seltzer, G. O., Anderson, D. M., Abbott, M. B., Enfield, D. B., and Newman, J. H. (1999). An ∼15,000-Year record of el nino-driven alluviation in southwestern Ecuador. Science 283, 516–520. doi:10.1126/science.283.5401.516
Rutllant, J., and Fuenzalida, H. (1991). Synoptic aspects of the central Chile rainfall variability associated with the Southern Oscillation. Int. J. Climatol. 11, 63–76. doi:10.1002/joc.3370110105
Sagredo, E. A., Kaplan, M., Araya, P., Lowell, T., Aravena, J., Moreno Moncada, P., et al. (2018). Trans-pacific glacial response to the Antarctic Cold Reversal in the southern mid-latitudes. Quat. Sci. Rev. 188, 160–166. doi:10.1016/j.quascirev.2018.01.011
Sagredo, E. A., Moreno, P. I., Villa-Martínez, R., Kaplan, M. R., Kubik, P. W., and Stern, C. R. (2011). Fluctuations of the Última Esperanza ice lobe (52ºS), Chilean Patagonia, during the last glacial maximum and termination 1. Geomorphology 125, 92–108. doi:10.1016/j.geomorph.2010.09.007
Schaetzl, R. J., and Forman, S. L. (2008). OSL ages on glaciofluvial sediment in northern Lower Michigan constrain expansion of the Laurentide ice sheet. Quat. Res. 70 (1), 81–90. doi:10.1016/j.yqres.2008.03.003
Singer, B., Hildreth, W., and Vincze, Y. (2000). 40Ar/39Ar evidence for early deglaciation of the central Chilean Andes. Geophys. Res. Lett. 27, 1663–1666. doi:10.1029/1999gl011065
Singh, V. P., Singh, P., and Haritashya, U. K. (2011). Encyclopedia of snow, ice and glaciers. Springer, 1254 p. ISBN: 978-90-481-2641-5.
Strelin, J. A., Denton, G. H., Vandergoes, M. J., Ninnemann, U. S., and Putnam, A. E. (2011). Radiocarbon chronology of the late-glacial puerto bandera moraines, southern patagonian icefield, Argentina. Quat. Sci. Rev. 30, 2551–2569. doi:10.1016/j.quascirev.2011.05.004
Stuiver, M., and Reimer, P. J. (1993). Extended 14C data base and revised CALIB 3.0 14C age calibration Program. Radiocarbon 35, 215–230. doi:10.1017/s0033822200013904
Stuut, J.-B. W., and Lamy, F. (2004). Climate variability at the southern boundaries of the Namib (southwestern Africa) and Atacama (northern Chile) coastal deserts during the last 120,000 yr. Quat. Res. 62 (3), 301–309. doi:10.1016/j.yqres.2004.08.001
Sweatman, M. B. (2021). The younger Dryas impact hypothesis: review of the impact evidence. Earth-Science Rev. 218, 103677. doi:10.1016/j.earscirev.2021.103677
Thiele, R. (1980). Hoja Santiago, región metropolitana, 39. Instituto de Investigaciones Geológicas, 51. Carta Geológica de Chile 1:250.000.
Valero-Garcés, B. L., Jenny, B., Rondanelli, M., Delgado-Huertas, A., Burns, S. J., Veit, H., et al. (2005). Palaeohydrology of Laguna de Tagua Tagua (34°30' S) and moisture fluctuations in Central Chile for the last 46 000 yr. J. Quat. Sci. 20, 625–641. doi:10.1002/jqs.988
Vargas, G., Klinger, Y., Rockwell, T. K., Forman, S. L., Rebolledo, S., Baize, S., et al. (2014). Probing large intraplate earthquakes at the west flank of the Andes. Geology 42, 1083–1086. doi:10.1130/g35741.1
Vargas, G., Rutllant, J., and Ortlieb, L. (2006). ENSO tropical-extratropical climate tele-connections and mechanisms for Holocene debris flows along the hyperarid coast of western South America (17°-24°S). Earth Planet. Sci. Lett. 249, 467–483. doi:10.1016/j.epsl.2006.07.022
Villalba, R., Grosjean, M., and Kiefer, T. (2009). Long-term multi-proxy climate reconstructions and dynamics in south America (LOTRED-SA): state of the art and perspectives. Palaeogeogr. Palaeoclimatol. Palaeoecol. 281, 175–179. doi:10.1016/j.palaeo.2009.08.007
Zech, J., Terrizzano, C., García-Morabito, E., Veit, H., and Zech, R. (2017). Timing and extent of late pleistocene glaciation in the arid central andes of Argentina and Chile (22°-41°s). Cuad. Investig. Geogr. 43 (2), 697–718. doi:10.18172/cig.3235
Zech, R., Kull, C., Kubik, P., and Veit, H. (2007). Exposure dating of late glacial and pre-LGM moraines in the cordon de Doña Rosa, northern/Central Chile (∼31° S). Clim. Past 3, 1–14. doi:10.5194/cp-3-1-2007
Zech, R., Kull, C., and Veit, H. (2006). Late Quaternary glacial history in the Encierro Valley, Northern Chile (29°S), deduced from 10Be surface exposure dating. Palaeogeogr. Palaeoclimatol. Palaeoecol. 234, 277–286. doi:10.1016/j.palaeo.2005.10.011
Zech, R., May, J., Kull, C., Ilgner, J., Kubik, P., and Veit, H. (2008). Timing of the late quaternary glaciation in the andes from ∼15 to 40° S. J. Quat. Sci. 23 (6-7), 635–647. doi:10.1002/jqs.1200
Keywords: late Quaternary glaciation, Antarctic Cold Reversal, Younger Dryas, glacial geomorphology, paleoclimate, Andes, central Chile
Citation: Herrera-Ossandón M, Easton G, Antinao JL and Forman SL (2023) Late Quaternary glacier advances in the Andes of Santiago, central Chile, and paleoclimatic implications. Front. Earth Sci. 11:1192812. doi: 10.3389/feart.2023.1192812
Received: 23 March 2023; Accepted: 20 September 2023;
Published: 12 October 2023.
Edited by:
Joseph Licciardi, University of New Hampshire, United StatesReviewed by:
Michael R. Kaplan, Columbia University, United StatesGordon Bromley, University of Galway, Ireland
Copyright © 2023 Herrera-Ossandón, Easton, Antinao and Forman. This is an open-access article distributed under the terms of the Creative Commons Attribution License (CC BY). The use, distribution or reproduction in other forums is permitted, provided the original author(s) and the copyright owner(s) are credited and that the original publication in this journal is cited, in accordance with accepted academic practice. No use, distribution or reproduction is permitted which does not comply with these terms.
*Correspondence: Gabriel Easton, Z2Vhc3RvbkB1Y2hpbGUuY2w=; Mariajosé Herrera-Ossandón, bWFoZXJyZXJhQHVkZWMuY2w=
†Present address: José Luis Antinao, Indiana Geological and Water Survey, Indiana University, Bloomington, IN, United States