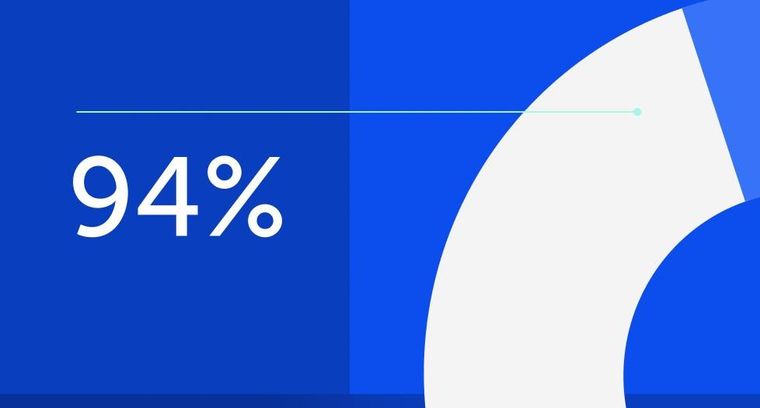
94% of researchers rate our articles as excellent or good
Learn more about the work of our research integrity team to safeguard the quality of each article we publish.
Find out more
ORIGINAL RESEARCH article
Front. Earth Sci., 23 June 2023
Sec. Structural Geology and Tectonics
Volume 11 - 2023 | https://doi.org/10.3389/feart.2023.1170791
This article is part of the Research TopicQuantitative Characterization and Engineering Application of Pores and Fractures of Different Scales in Unconventional Reservoirs, volume IIIView all 15 articles
Natural fractures act as critical flow channels and reservoir space in the Lucaogou Formation tight reservoir of the Jimsar Sag. It is essential to identify the main controlling factors of natural fractures in order to achieve efficient development of tight oil in this area. There are mainly three types of natural fractures, including tectonic fractures, diagenetic fractures, and abnormal overpressure-induced fractures. Diagenetic fractures are predominantly bedding seams. The fracture development is affected by multiple factors including brittle minerals, lithology, tectonic stress, bed thickness, and total organic carbon (TOC). Large tectonic stress, smaller bed thickness, and higher total organic carbon are all favorable for the development of tectonic fractures and bedding seams. The controls of brittle minerals and lithology on fracture development are different for tectonic fractures and bedding seams. Specifically, carbonate minerals stimulate the tectonic fracture development, while brittle minerals have no control over the bedding seam development; tectonic fractures are most developed in the dolomitic rocks, while bedding seams are most developed in the argillaceous rocks. The calculated fracture density variation coefficients reveal that tectonic stress and brittle minerals are the main controlling factors of tectonic fracture development; total organic carbon and lithology are the main control factors of bedding seam development.
Tight Reservoirs are typically composed of fine-grained sedimentary rocks with low matrix permeability (Nelson, 2009; Ghanizadeh et al., 2015; Zhang et al., 2021). Natural fractures play important roles in these tight reservoirs, because they act as flow channels for hydrocarbon migration and they can connect hydraulic fractures and matrix pores with the wellbore during production (Becker et al., 2010; Li et al., 2019; Zhao et al., 2021). Therefore, it is of great significance to investigate the controlling factors of natural fractures in order to evaluate the occurrence and distribution of oil and gas in tight reservoirs, thereby minimizing drilling and well-completion costs (Gale et al., 2007; Fall et al., 2012; Laubach et al., 2016).
The Permian Lucaogou Formation of the Junggar Basin is one of the main targets for tight oil exploration in China. Since 2010, breakthroughs have been made in the southeastern Junggar Basin, including industrial oil production in Wells J30, J174, and J251 (Du et al., 2014). This might be related to adjacent high-quality source rocks, which have a total organic carbon (TOC) content of >2%, a predominance of Type-II1 organic matter, and a maturity (Ro) of 0.8%–1.0%. In general, the Permian Lucaogou Formation source rocks in the Junggar Basin are in the low-mature to mature stage, presenting high oil generation potential that is estimated to be 380 million tons (Kuang et al., 2012; Cao et al., 2016).
The Jimsar Sag experienced multiple stages of tectonic activities during Late Paleozoic, Mesozoic, and Cenozoic, which resulted in complex stratigraphic configurations and structural characteristics as well as abundant tectonic fractures (Wu et al., 2013; Zhang et al., 2017). Moreover, complex diagenesis and extensive hydrocarbon generation and expulsion have vital effects on the opening of bedding seams. These fractures greatly impact the oil content in the Lucaogou Formation tight reservoirs. Nonetheless, previous research mainly focuses on the tectonic setting (Zheng et al., 2018), reservoir rock lithology (Cao et al., 2019), pore structure (Liu et al., 2019; Tian et al., 2019), and sedimentary characteristics (Ma et al., 2019), with insufficient attention paid to illustrating the main controlling factors of natural fractures. This knowledge gap restrains the efficient exploration and development of tight oil in the study area.
In this study, core description, Total Organic Carbon (TOC) analysis, Xray diffraction (XRD) analysis, and Focused Ion Beam Scamming Electron Microscopy (FIB-SEM; Wang et al., 2016) were used to study the fracture development characteristics and genesis in the tight reservoirs of the Lucaogou Formation in the Jimsar Sag. The objectives are four-fold: 1) to identify fractures in the tight oil reservoirs via several approaches; 2) to clarify the types, formation mechanisms, and development characteristics of the fractures; 3) to build a comprehensive system for fracture parameter characterization and evaluation; and 4) to reveal main factors controlling the fracture development in tight oil reservoirs.
The Junggar Basin is located in the southern part of the Central Asian orogeny (Figure 1A), covering an area of about 1.3×105 km2. It is a Mesozoic-Cenozoic sedimentary basin developing on the Paleozoic basement (Li et al., 2016; Zhang et al., 2017). The Jimsar Sag lies in the southeastern part of the basin (Figure 1B) and presents itself as a half-graben sag on a Middle Carboniferous fold basement. It is bounded by faults from all directions, including the Jimsar fault in the north, the Laozhuangwan Fault in the northwest, the Xidi fault in the southwest, the Santai fault in the south, and the Houbuzi fault in the southeast (Figure 1C).
FIGURE 1. (A) Geological map of the Central Asian orogeny (Zhang et al., 2017). (B) Structural units of the Junggar Basin and the location of the Jimsar Sag (Zhang et al., 2017). (C) Structural map of the Jimsar Sag (Zhang et al., 2017).
The Jimsar Sag has experienced multi-stage tectonic movements since the Paleozoic (Zhang et al., 2017). The Jimsar fault was formed during the Late Carboniferous, when the Shaqi Uplift in the north of the Jimsar Sag was formed. The Jimsar Sag experienced intensive tectonic subsidence during the early Middle Permian, followed by the deposition of lacustrine sediments during the Late Permian to the Early Triassic (Wu et al., 2013; Zhang et al., 2017). The latest exploration demonstrates that oil reservoirs occur in the Permian Lucaogou and Wutonggou Formations.
The Lucaogou Formation is divided into the first (P2l1) and second (P2l2) members from bottom to top, referred to as the Lu-1 and Lu-2 Members, respectively. The Lu-1 Member is composed of the upper first sand group (P2l11) and the lower second sand group (P2l12), while the Lu-2 Member consists of the first (P2l21) and second (P2l22) sand groups from top to bottom (Figure 2). The Permian Lucaogou Formation is seen with long-interval hydrocarbon shows in high-permeability and high-porosity “sweet spots”. There are broadly two sweet spots in the Lucaogou Formation, namely, the second sand group of the Lu-2 Member (P2l22) (the upper sweet spot) and the second sand group of the Lu-1 Member (P2l12) (the lower sweet spot). They are subdivided into 10 layers in accordance with their physical properties, including four layers of the upper sweet spot (STD 1–4) and six layers of the lower sweet spot (XTD 1–6).
FIGURE 2. Stratigraphic correlation of the Lucaogou Formation in the Jimsar Sag, the Junggar Basin (modified from an internal report of the PetroChina Karamay Oilfield).
To assess the main factors controlling fractures in tight oil reservoirs, we analyzed the mineral content and TOC content, and observed Focused Ion Beam Scamming Electron Microscopy of cores from seven wells (J30, J32, J174, J015, J5, J251, and J15). All experiments are performed in the State Key Laboratory of China University of Petroleum (Beijing).
A total of 130 samples from seven wells were selected for mineralogical composition analysis. Bulk minerology was determined via Xray diffraction (XRD) analysis. Crushed samples were mixed with ethanol, ground by hand and then smear-mounted on glass slides to create randomly oriented powder preparations (48 μm). Measurements were conducted on a Bruker D8 DISCOVER diffractometer, using Co. Kα-radiation at 45 kV and 35 mA. The diffffracted beam was measured with a scintillation detector. Quantitative phase analysis was performed using Rietveld refifinement, with customized clay mineral structure models (Wang et al., 2020).
A total of 36 samples from seven wells were selected for Total Organic Carbon (TOC) analysis. Powdered samples were weighed, then acidifified with hydrochloric acid to remove carbonates. After rinsing and drying, de-carbonated samples were reweighed and combusted at high temperature in a Leco C230 carbon analyzer. Total organic carbon content is expressed as a weight percentage. This analysis was conducted in accordance with the Chinese National Standard GB/T19145-2003 (Wang et al., 2020).
Focused Ion Beam Scamming Electron Microscopy (FIB-SEM) is a method to focus a beam of ions on and scanning over the samples (Wang et al., 2016). The atomic bombardment on the surfaces of the samples by the ion beam will sputter the atoms. It provides a neo technology for the research of the micro-nanopores. The instrument used was a FEI-HELIOS-NanoLab 650 manufactured by US FEI Company. As observed with the backscattering function, the appearance of the organic matter was black with lowest brightness, the appearance of the pyrite was white with the highest brightness, and the appearance of the matrix minerals such as quartz and calcite was light gray. The pores were black notably.
On an overall basis, the Permian Lucaogou Formation tight reservoir has well-developed natural fractures, as observed in outcrops, cores, and thin sections. These fractures are grouped into three types: tectonic fractures, diagenetic fractures, and abnormal overpressure-induced fractures.
Tectonic fractures refer to fractures whose formation and distribution are controlled by local tectonic events or the tectonic stress field. Types, development degrees, and occurrences of tectonic fractures are dependent on the stress field distribution and tectonic position (Hou, 1994; Zeng et al., 2007; Yu et al., 2016). Tectonic fractures are widely developed in field outcrops and mainly include shear and tensile fractures. Some shear fractures present themselves in a conjugate arrangement (typically, X-shaped) in field outcrops (Figures 3A, B), with stable occurrence and long extension. The fracture surface is flat and smooth and is often found with striations. Field observation shows that shear fractures have a length range of 1–5 cm and an aperture range of 0.02–0.1 cm. Tensile fractures are scattered, with limited extensions and zigzag fracture surfaces. Their lengths are mainly 1–2 cm and the aperture is 0.02–0.3 cm (Figure 3C). In cores, some shear fractures only have one part of the expected X-shape (Figure 3E), which may be attributed to the anisotropic compressive strength associated with rock heterogeneity (Zeng et al., 2008). The core observation shows that fillings inside tectonic fractures are mostly quartz and calcite, with sporadic argillaceous fillings.
FIGURE 3. Photos of tectonic fractures, bedding seams, and abnormal overpressure-induced fractures. (A) Shear fractures in outcrops, Dalongkou reservoir profile. (B) Shear fractures in outcrops, Xiaolongkoucun profile. (C) Tensile fractures in outcrops, Dalongkoucun profile. (D) Bedding seams in outcrops, Dalongkou reservoir profile. (E) Tensile fractures in cores, 3590.60 m, Well J251. (F) Bedding seams in cores, 2311.80 m, Well J29. (G) Bedding seams in cores, 2363.42 m, Well J176. (H) Abnormal overpressure-induced fractures in cores, 4044.72 m, Well J30. (I) Abnormal overpressure-induced fractures in cores, 3126.42 m, Well J174.
Diagenetic fractures are near-horizontal fractures generated via geological processes such as pressure solution, compaction, and pressure relief (He et al., 2011; Luo et al., 2017; Zeng et al., 2017). Diagenetic fractures in this study are predominantly bedding seams, with main occurrences at interfaces of bedding. They are parallel to each other and follow the directions of rock beds and micro-bedding. At the outcrop scale, these fractures have long extensions of 5–15 m and yet limited apertures of 0.05–0.1 cm (Figure 3D). At the core scale, they can penetrate the whole core (Figures 3F, G) with apertures of 0.02–0.1 cm. Field outcrops and drilling cores both show that diagenetic fractures are mostly filled with calcite, quartz, argillaceous minerals, and hydrocarbons.
Abnormal overpressure-induced fractures are found in cores, which are mostly drainage fractures formed via hydraulic processes (Liu et al., 2017; Zhang et al., 2017; Zeng et al., 2017). They are mostly observed at the core scale (Figures 3H, I) and are less seen in the field outcrop. They present irregular distribution in the form of vein groups and curved extensions in cores. Their apertures are highly variable—ranging from 0.5 mm to 10 mm, with a maximum of 20 mm. Their lengths are generally several centimeters. Most of them are filled and have low oil content, as seen in cores.
Reservoir fractures are controlled by numerous factors (Zeng et al., 2013; Bucknall and Polymer., 2016; Ju and Sun, 2016). Moreover, their distribution and development degree are highly heterogeneous, which plays an important role in the exploration and development of tight reservoirs. However, previous studies mostly target fractures in conventional reservoirs, with insufficient attention to fractures in tight reservoirs. In this study, five factors are found to control the development of tectonic fractures and diagenetic fractures, including brittle minerals, lithology, bed thickness, tectonic stress, and TOC.
Brittle minerals such as calcite and quartz can normally promote the development of fractures (Zeng and Li, 2010). However, this kind of promotion varies from mineral to mineral. There are three methods of mineral brittleness evaluation (Diao, 2013; Wang et al., 2013; Wan et al., 2016), which are respectively based on i) Young’s modulus and Poisson ratio (elastic parameters), ii) the relative content of brittle and clay minerals, and iii) mineral composition. Particularly, carbonate rocks are found to significantly contribute to the brittleness of reservoirs rich in carbonate minerals (Fridrun et al., 2015).
The studied Lucaogou Formation has a high content of carbonate rocks, with a wide range of 3.6%–87.3% and an average of 45.8%. Therefore, carbonate rocks should be one of the most influential factors of reservoir brittleness. In this study, the brittleness is characterized by the ratio of different minerals:
Quartz brittleness = quartz/(quartz + carbonate minerals + clay minerals).
Carbonate mineral brittleness = carbonate minerals/(quartz + carbonate minerals + clay minerals).
Bulk-rock brittleness = (quartz + carbonate minerals)/(quartz + carbonate minerals + clay minerals).
As shown in Figure 4, the studied reservoir has high brittleness, with bulk-rock brittleness above 0.5. The bulk-rock brittleness presents no notable correlation with quartz brittleness (Figure 4A), while it seems to linearly scale with the carbonate mineral brittleness (Figure 4B). Therefore, carbonate minerals are more important to the bulk-rock brittleness.
FIGURE 4. Correlations between different types of brittleness with 130 sample points from six typical wells (J5, J15, J30, J32, J174, and J251). (A) Quartz brittleness vs. bulk-rock brittleness. (B) Carbonate brittleness vs. bulk-rock brittleness.
As illustrated in Figure 5, feldspar, quartz, pyrite, calcite, and dolomite have absolute controls on the development of tectonic fractures. In contrast, they have no notable control on the development of diagenetic fractures (Figure 6). Specifically, dolomite and calcite are the main controlling factors of tectonic fractures (Figure 5).
FIGURE 5. Ternary diagrams of mineral compositions for samples with tectonic fractures. (A) All samples (130 samples). (B) Samples with a tectonic fracture density greater than 20 m-1. (C) Samples with a tectonic fracture density of 15–20 m-1. (D) Samples with a tectonic fracture density of 10–15 m-1. (E) Samples with a tectonic fracture density of 5–10 m-1. (F) Samples with a tectonic fracture density below 5 m-1.
FIGURE 6. Ternary diagrams of mineral compositions for samples with diagenetic fractures. (A) All samples (130 samples). (B) Samples with a diagenetic fracture density greater than 20 m-1. (C) Samples with a diagenetic fracture density of 15–20 m-1. (D) Samples with a diagenetic fracture density of 10–15 m-1. (E) Samples with a diagenetic fracture density of 5–10 m-1. (F) Samples with a diagenetic fracture density below 5 m-1.
In this study, samples are collected from intervals that are far away from faults, belong to the same tectonic belt, and have similar bed thicknesses. The linear densities of tectonic and diagenetic fractures are summarized and plotted for several lithologies, including dolomite, dolomitic sandstone, dolomitic mudstone, limestone, siltstone, fine-grained sandstone, medium-grained sandstone, coarse-grained sandstone, conglomerate, sandy mudstone, muddy sandstone, limy mudstone and mudstone of the upper and lower sweet spots respectively.
As illustrated in Figure 7, the same lithology has the same control on fracture development in the upper and lower sweet spots. Tectonic fractures are more developed in dolomitic rocks (Figure 7A), with linear densities of 2.80 m-1 in dolomite, 2.46 m-1 in dolomitic sandstone, and 1.97 m-1 in dolomitic mudstone for the upper sweet spot; those values for the lower sweet spot are 3.18, 2.25 and 2.29 m-1, respectively. This is because dolomitic rocks are more brittle—under the same stress conditions, their bearable strains before cracking are smaller and they are more prone to generating tectonic fractures than soft/plastic rocks (Zeng et al., 2008). For both the upper and lower sweet spots, the linear density of tectonic fractures drops in the order of limestone, siltstone, fine-grained sandstone, medium-grained sandstone, and conglomerate, which indicates the control of rock particle sizes on the development of tectonic fractures. With smaller particles and lower pore volumes, rocks are associated with higher rigidity brittleness and are easier to crack under tectonic stress. Muddy rocks tend to absorb more stresses via plastic deformation, and the resultant development of tectonic fractures is relatively low.
FIGURE 7. Lithology vs. fracture density for the upper and lower sweet spots. Green and red represent the upper sweet spot and the lower sweet spot, respectively. (A) tectonic fracture. (B) diagenetic fracture.
The control of lithology on diagenetic fractures is considerably different from that on tectonic fractures. Diagenetic fractures are more developed in argillaceous rocks (i.e., mudstone, dolomitic mudstone, muddy sandstone, and limy mudstone) (Figure 7B), with the maximum linear density in mudstones, reaching 4.81 m-1. Diagenetic fractures are also well-developed in carbonate rocks (dolomite and muddy dolomite). The plentiful diagenetic fractures in muddy rocks are attributed to the intensive hydrocarbon generation and expulsion of organic matter in such rocks, which can cause dissolution and pressurization.
To conclude, lithology has strong control effects on the development of tectonic and diagenetic fractures. Specifically, tectonic fractures are most developed in dolomite, with an average density of 2.96 m-1. Compared with siltstone and fine-grained sandstone, argillaceous rocks have lower development of tectonic fractures, as they have larger plastic deformation to absorb more stresses under the same tectonic condition. Meanwhile, medium-grained sandstone, coarse-grained sandstone, and conglomerate have the least developed tectonic fractures, because their larger particles and higher pore volumes lead to lower strengths that, in turn, promote resistance to cracking after elastic deformation. As for diagenetic fractures, they are most developed in mudstone, with an average density of 4.85 m-1, which is attributed to the organic acid dissolution and pressurization during hydrocarbon generation and expulsion.
Tectonic stress represents a main control on the reservoir fracture development. It is easier to form fractures in more tectonically-active zones. Statistics of field outcrops show that tectonic stress has consistent controls on tectonic and diagenetic fractures. With the same lithology, the tectonic fracture density grows in zones nearer to faults, and the same pattern is also identified for diagenetic fractures (Figure 8), due to the opening of weak bedding planes driven by tectonic stress. It is also noted in field outcrops that the densities of tectonic and diagenetic fractures are higher around larger faults than those near smaller faults. In addition, field outcrops are often associated with conjugate shear fractures, while cores tend to present a single shear fracture. This may be attributed to the underground confining pressure, which in turn reflects the control of tectonic stress on fracture development.
Observation of field outcrops and cores reveals that mudstone and sandstone alternate with each other in forms of interbedding, which leads to the higher development of bedding seams/diagenetic fractures. To eliminate the effects of dissolution, the following analysis involves only sandstone, since dolomite contains higher contents of carbonate minerals. Statistics suggest that tectonic fractures and bedding seams are both affected by bed thickness. Their density climbs up, as the bed becomes thinner. The bed thickness over 3 m is found with the least development of fractures; on the contrary, the highest development of fractures occurs in the case of the bed thickness below 0.5 m (Figure 9).
FIGURE 9. Correlation between fracture density and bed thickness. The frequency of bedding fractures and tectonic fractures decreases with the increase of layer thickness.
Under the same stress, organic matter abundance is one of the factors affecting fracture development in mudstones (Liu et al., 2017; Zhang et al., 2017). The control of TOC on fracture development depends on organic matter distribution, organic matter and water consumption by hydrocarbon generation, and hydrocarbon generation-induced pressurization. The existence of organic matter bands becomes more prominent and influential, with higher organic matter abundance, and micro-fractures tend to occur inside and at the edge of such organic matter bands (Zhang et al., 2017).
The Lucaogou Formation in the Jimsar Sag has extensive development of argillaceous source rocks (Kuang et al., 2014), with TOC mostly of 1.29%–13.82% and averaging 5.34%. Fractures are created via the pressurization and dissolution processes during hydrocarbon generation and expulsion.
Microfractures often occur inside and at the edge of the organic matter (Figure 10), which may be attributed to the consumption of water and organic matter by hydrocarbon generation or the pressurization also induced by hydrocarbon generation (Zhang et al., 2017). The organic matter adjacent to clay minerals (e.g., illite) is also seen with numerous internal pores and fractures. The reason behind this observation may be that a large volume of fluids is generated during the conversion from smectite to illite to form transitional smectite-illite mixed minerals; such minerals are highly catalytic and promote hydrocarbon generation to create more organic pores and fractures (Zhang et al., 2017).
FIGURE 10. SEM images of fractures generated by hydrocarbon generation. (A, B) Microfractures occur inside of the organic matter. (C, D) Microfractures occur at the edge of the organic matter.
Here the STD-4 layer of the upper sweet spot and the XTD-1 layer of the lower sweet spot are taken as examples to investigate the correlation between TOC and fracture development. The STD-4 layer is mainly composed of dolomitic mudstone and dolomite, with a total content of calcite and dolomite up to 45.89%, while the XTD-1 layer is predominantly silty-fine sandstone and sandy mudstone, with the total calcite-dolomite content of 32.50%. As revealed in Figure 11, TOC has considerably decisive effects on the development of bedding seams. For both the STD-4 and XTD-1 layers, the bedding seam density grows with the rising TOC. Although the tectonic fracture density also climbs up with the increasing TOC, such a variation trend is weaker than that of bedding seams. To sum up, higher TOC results in the higher development of both tectonic fractures and bedding seams, and yet the control of TOC on bedding seams is stronger than that on tectonic fractures.
FIGURE 11. TOC vs. fracture density for the upper and lower sweet spots. Fracture density increase with the increase of TOC. (A) Tectonic fractures. (B) Bedding seams.
The incremental pressure induced by hydrocarbon generation can lead to the failure of rocks and the opening of weak bedding planes to form tectonic fractures and bedding seams respectively (Luo et al., 2017; Fall et al., 2015). In addition, hydrocarbons and acid fluids expelled during hydrocarbon generation may dissolve bedding planes to form bedding seams (Fall et al., 2015). Some fractures have prominent hydrocarbon shows, indicating that the hydrocarbon generation-expulsion is one of the important contributors to fracture formation.
As discussed in Section 4, the development of tectonic fractures varies with layer thicknesses. However, outcrops, cores, and imaging logging all reveal that the Lucaogou Formation reservoir is mostly transitional rocks, with interbedding of centimeter-scale beds of different lithologies. Statistics of bed thicknesses in cores show that beds thinner than 10 cm account for more than 80% of the total (Figure 12). Therefore, it is safe to say that the dependency of tectonic fracture development on bed thickness is rather small for the Lucaogou Formation characterized by the “relatively homogeneous” bed thickness, despite that the bed thickness to some extent restrains the development of tectonic fractures.
Linear positive correlations are found between TOC and tectonic fracture density for both the STD-4 and XTD-1 layers of the upper and lower sweet spots respectively. However, no notable correlation is found in the statistics of the samples of the four layers in the upper sweet spot (Figure 13). It is noted that samples from STD-4 and XTD-1 layers (Figure 11A) have similar brittleness indexes, whereas those from the four layers of the upper sweet spot in Figure 13 have varied brittle mineral contents. Moreover, the overall brittleness index of the upper sweet spot is lower than those of STD-4 and XTD-1 layers. In other words, with varied contents of quartz and carbonate minerals, no notable correlation is found between TOC and tectonic fracture density. This means that the development of tectonic fractures is affected jointly by mineral brittleness and TOC, and the effect of brittle minerals is stronger than that of TOC.
The above analysis indicates that brittle minerals, lithology, and tectonic stress may be the main control factors for fracture development in the Lucaogou Formation tight reservoir among all influential factors. To quantitatively characterize the main control factors for fracture development, the fracture density variation coefficient is introduced in this research (Yang, 2011).
where V is the fracture density variation coefficient, dimensionless; δf is the standard deviation of a variable; fa is the mean fracture density of all samples;
The coefficient of variation of fracture density can be used to evaluate the impact of a single factor, with a larger value indicating a stronger impact. Tectonic stress and brittle minerals are found to result in the largest coefficient of variation, followed by lithology, TOC, and bed thickness successively (Table 1).
TABLE 1. The coefficients of variation of tectonic fracture density corresponding to various control factors.
As discussed in Section 4, brittle minerals have no considerable control over the development of bedding seams—bedding seams can be well developed in rocks with high and low calcite-dolomite contents. Similar to the case of tectonic fractures, the “relatively homogenous” bed thickness results in low dependency of the bedding seam development on layer thickness. It is often observed in field outcrops that the bedding seam density grows as it approaches the fault in the case of the same lithology; nevertheless, the bedding seam density of mudstone far away from the fault is higher than that of sandstone nearer to the fault. These demonstrate the higher effects of lithology than tectonic stress on the development of bedding seams.
The above analysis shows lithology and TOC may be the main control factors of the bedding seam development. This is verified by the calculated coefficients of variation of the bedding seam density. In addition, as suggested in Table 2, the effects of tectonic stress and bed thickness on the bedding seam development are rather small.
TABLE 2. The variation coefficients of bedding seam density corresponding to various control factors.
1) Natural fractures in the Lucaogou Formation tight reservoir, Jimsar Sag are mainly three types, including tectonic fractures, diagenetic fractures, and abnormal overpressure-induced fractures. Tectonic fractures include shear and tensile fractures; diagenetic fractures are predominantly bedding seams; abnormal overpressure-induced fractures are mainly drainage fractures.
2) The development of natural fractures is affected by brittle minerals, lithology, tectonic stress, bed thickness, and TOC. Specifically, dolomite and calcite (carbonate minerals) make the greatest contributions to the formation of tectonic fractures; in contrast, brittle minerals have no notable control over the development of bedding seams. The dolomitic rock has the highest development of tectonic fractures, while the most-developed bedding seams are found in the argillaceous rocks. The development of both tectonic fractures and bedding seams grows with the increasing tectonic stress. Higher bed thickness leads to suppressed development of both tectonic fractures and bedding seams. At last, the densities of both tectonic fractures and bedding seams are inversely proportional to TOC.
3) Tectonic stress and brittle minerals are the main control factors of the development of tectonic fractures. For bedding seams, the main control factors are TOC and lithology.
The datasets presented in this study can be found in online repositories. The names of the repository/repositories and accession number(s) can be found in the article/supplementary material.
YY, ZD, and JG contributed to conception and design of the study. CZ organized the database. YY, ZD, and CZ performed the statistical analysis. YY wrote the first draft of the manuscript. ZD, JG, and CZ wrote sections of the manuscript. All authors contributed to the article and approved the submitted version.
This work is financially supported by the grants from PetroChina Research Institute of Petroleum Exploration and Development (No. 2022KT1401, 2021DJ2302). Key Laboratory of Tectonics and Petroleum Resources (China University of Geosciences, Ministry of Education, Wuhan 430074, China) (No. TPR-2021-01).
The authors ZD and JG were employed by PetroChina Research Institute of Petroleum Exploration and Development. The author PL was employed by Bureau of Geophysics Prospecting Inc.
The authors declare that this study received funding from China University of Petroleum, Beijing. The funder had the following involvement in the study: design, collection, and writing of this article. The authors declare that this study received funding from PetroChina Research Institute of Petroleum Exploration and Development. The funder had the following involvement in the study: the decision to submit it for publication and interpretation of data. The authors declare that this study received funding from Bureau of Geophysics Prospecting Inc, CNPC, Research Center of Geology. The funder had the following involvement in the study: design, data collection and analysis. The authors declare that this study received funding from Chengdu University of Technology, Chengdu. The funder had the following involvement in the study: design, preparation of the manuscript.
All claims expressed in this article are solely those of the authors and do not necessarily represent those of their affiliated organizations, or those of the publisher, the editors and the reviewers. Any product that may be evaluated in this article, or claim that may be made by its manufacturer, is not guaranteed or endorsed by the publisher.
Becker, S. P., Eichhubl, P., Laubach, S. E., Reed, R. M., Lander, R. H., and Bodnar, R. J. (2010). A 48 m.y. history of fracture opening, temperature, and fluid pressure: Cretaceous Travis Peak Formation, East Texas basin. Bone 38 (5), 1081–1093. doi:10.1130/b30067.1
Bucknall, C. B., and Polymer, G. (2016). Fracture of: Factors controlling toughness. Reference module in materials science and materials engineering. Encyclopedia of Materials: Science and Technology, 7322–7326.
Cao, Y. C., Zhu, N., Zhang, S. M., Xi, K. L., and Xue, X. J. (2019). Diagenesis and reserving space characteristics of tight oil reservoirs of permian Lucaogou Formation jimusar sag of jungar basin, China. J. Earth Sci. Environ. 41 (3), 253–266.
Cao, Z., Liu, G. D., Kong, Y. H., Wang, C. Y., Niu, Z. C., Zhang, J. Y., et al. (2016). Lacustrine tight oil accumulation characteristics: Permian Lucaogou Formation in jimusaer sag, Junggar Basin. Int. J. Coal Geol. 153, 37–51. doi:10.1016/j.coal.2015.11.004
Diao, H. Y. (2013). Rock mechanical properties and brittleness evaluation of shale reservoir. Acta Petrol. Sin. 29 (9), 3300–3306.
Du, J. H., He, H. Q., Li, J. Z., Yang, T., Haung, F. X., Guo, B. C., et al. (2014). Progress in China's tight oil exploration and challenges. China Pet. Explor. 19 (1), 1–8.
Fall, A., Eichhubl, P., Bodnar, R. J., Laubach, S. E., and Davis, J. S. (2015). Natural hydraulic fracturing of tight-gas sandstone reservoirs, Piceance Basin, Colorado. Geol. Soc. Am. Bull. 127 (1-2), 61–75. doi:10.1130/b31021.1
Fall, A., Eichhubl, P., Cumella, S. P., Bodnar, R. J., Laubach, S. E., and Becker, S. P. (2012). Testing the basin-centered gas accumulation model using fluid inclusion observations: Southern Piceance Basin, Colorado. AAPG Bull. 96 (12), 2297–2318. doi:10.1306/05171211149
Fridrun, P., Newton, J. M., and Paul, F. (2015). The bending strength of tablets with a breaking line-Comparison of the results of an elastic and a "brittle cracking" finite element model with experimental findings. Int. J. Pharm. 495 (1), 485–499. doi:10.1016/j.ijpharm.2015.09.004
Gale, J. F. W., Robert, M. R., and Holder, J. (2007). Natural fractures in the Barnett Shale and their importance for hydraulic fracture treatments. AAPG Bull. 91 (4), 603–622. doi:10.1306/11010606061
Ghanizadeh, A., Clarkson, C. R., Aquino, S., Ardakani, O. H., and Sanei, H. (2015). Petrophysical and geomechanical characteristics of Canadian tight oil and liquid-rich gas reservoirs: I. Pore network and permeability characterization. Fuel 153 (1), 664–681. doi:10.1016/j.fuel.2015.03.020
He, Z. J., Liu, B. J., and Wang, P. (2011). Genesis of bedding fractures and its influences on reservoirs in Jurassic, Yongjin area, Junggar Basin. Petroleum Geol. Recovery Effic. 18 (1), 15–17. doi:10.13673/j.cnki.cn37-1359/te.2011.01.004
Hou, G. T. (1994). Fractal analysis of fractures. J. Basic Sci. Eng. 2 (4), 299–305. doi:10.16058/j.issn.1005-0930.1994.04.005
Ju, W., and Sun, W. F. (2016). Tectonic fractures in the lower cretaceous xiagou Formation of qingxi Oilfield, jiuxi basin, NW China. Part two: Numerical simulation of tectonic stress field and prediction of tectonic fractures. J. Petroleum Sci. Eng. 146, 626–636. doi:10.1016/j.petrol.2016.05.002
Kuang, L. C., Gao, G., Xiang, B. L., Wang, X. L., Wang, C. Y., and Liu, G. D. (2014). Lowest limit of organic carbon content in effective source rocks from Lucaogou Formation in Jimusar Sag. Petroleum Geol. Exp. 36 (2), 224–229. doi:10.11781/sysydz201402224
Kuang, L. C., Tang, Y., Lei, D. W., Chang, Q. S., Ou, Y. M., Hou, L. H., et al. (2012). Formation conditions and exploration potential of tight oil in the Permian saline lacustrine dolomitic rock, Junggar Basin, NW China. Petroleum Explor. Dev. 39 (6), 700–711. doi:10.1016/s1876-3804(12)60095-0
Laubach, S. E., Fall, A., Copley, L. K., Marrett, R., and Wilkins, S. J. (2016). Fracture porosity creation and persistence in a basement-involved laramide fold, upper cretaceous frontier formation, green river basin, USA. Geol. Mag. 153 (5-6), 887–910. doi:10.1017/s0016756816000157
Li, D., He, D. F., and Tang, Y. (2016). Reconstructing multiple arc-basin systems in the altai–junggar area (NW China): Implications for the architecture and evolution of the Western central asian orogenic belt. J. Asian Earth Sci. 121, 84–107. doi:10.1016/j.jseaes.2016.02.010
Li, H., Tang, H. M., Qin, Q. R., Zhou, J. L., Qin, Z. J., Fan, C. H., et al. (2019). Characteristics, formation periods and genetic mechanisms of tectonic fractures in the tight gas sandstones reservoir: Acase study of Xujiahe Formation in YB area, Sichuan Basin, China. Jourmal Petroleum 5cience Eneineering 178, 723–735. doi:10.1016/j.petrol.2019.04.007
Liu, D. D., Zhang, C., Luo, Q., Zhang, Y. D., Gao, Y., Zhang, Y. C., et al. (2017). Development characteristics and controlling factors of natural fractures in Permian Lucaogou Formation tight reservoir in Jimsar sag, Junggar Basin. China Pet. Explor. 22 (4), 36–47. doi:10.3969/j.issn.1672-7703.2017.04.004
Liu, Y. S., Dong, X. H., Yan, L., Chen, F. L., Gao, Y. L., and Chen, Z. X. (2019). Quantitative characterization of pore structure of Lucaogou Formation in jimsar sag. Xinjiang Pet. Geol. 40 (3), 284–289. doi:10.7657/XJPG20190305
Luo, Q., Wei, H. Y., Liu, D. D., Zhang, C., Zu, D. Y., Zhang, Y. C., et al. (2017). Geofluids in deep sedimentary basins and their significance for Petroleum accumulation. Petroleum Geol. Exp. 39 (1), 1–4. doi:10.1155/2017/3571359
Ma, K., Liu, Y. M., Hou, J. G., Huang, S., Yan, L., Chen, F. L., et al. (2019). Densification mechanism of tight reservoirs from mixed sedimentation in saline lacustrine environment: A case study of permian Lucaogou Formation, jimsar sag. Xinjiang Pet. Geol. 40 (3), 253–261.
Nelson, P. H. (2009). Pore-throat sizes in sandstones, tight sandstones, and shales. AAPG Bull. 93 (3), 329–340. doi:10.1306/10240808059
Tian, W., Liu, H. Q., He, S. L., Wang, J., and Xie, l. (2019). Characterization of microscopic pore structure of tight oil reservoirs in Lucaogou Formation,Jimusaer Sag. Petroleum Geol. Recovery Effic. 26 (4), 24–32. doi:10.13673/j.cnki.cn37-1359/te.2019.04.004
Wan, X. Q., Peng, C., Pan, J. N., Wang, X. Y., and Dong, Y. Z. (2016). Coal measures argillutite mineral composition and brittleness analysis in yuzhou coalfield. Coal Geol. China 28 (10), 24–28. doi:10.3969/j.issn.1674-1803.2016.10.06
Wang, P. F., Jiang, Z. X., Ji, W. M., Zhang, C., Yuan, Y., Chen, L., et al. (2016). Heterogeneity of intergranular, intraparticle and organic pores in longmaxi shale in sichuan basin, south China: Evidence from SEM digital images and fractal and multifractal geometries. Mar. Petroleum Geol. 72, 122–138. doi:10.1016/j.marpetgeo.2016.01.020
Wang, P., Ji, Y. L., Pan, R. F., Wang, Z. Z., and Wu, Y. (2013). A comprehensive evaluation method of shale brittleness: A case study from the lower silurian longmaxi Fm in block W, sichuan basin. Nat. Gas. Ind. 33 (12), 48–53. doi:10.3787/j.issn.1000-0976.2013.12.006
Wang, X., Jiang, Z. X., Zhang, K., Wen, M., Xue, Z. X., Wu, W., et al. (2020). Analysis of gas composition and nitrogen sources of shale gas reservoir under strong tectonic events: Evidence from the complex tectonic area in the yangtze plate. Energies 13 (1), 281. doi:10.3390/en13010281
Wu, J. J., You, L. P., and Yang, H. S. (2013). Structural evolution and hydrocarbon accumulation of Fukang fault zone in Junggar Basin. Xinjiang Pet. Geol. 34 (1), 36–40.
Yang, X. J. (2011). Characteristics and origin of fractures in tight sandstone reservoirs with low permeability, Dabei Gas Field. China: China University of Petroleum. (East China).
Yu, X., Hou, G. T., Neng, Y., Li, J., and Wei, H. X. (2016). Development and distribution characteristics of tectonic fractures in kuqa depression. Geol. J. China Univ. 22 (4), 644–656. doi:10.16108/j.issn1006-7493.2015255
Zeng, L. B., Kang, Y. S., and Xiao, S. R. (2008). Development characteristics and geneses of the fractures in low-permeability sandstone reservoir in the northern depression of Tuha Basin. J. Xi’an Shiyou Univ. Nat. Sci. Ed. 23 (1), 22–25.
Zeng, L. B., and Li, Y. G. (2010). Tectonic fractures in tight gas sandstones of the upper triassic xujiahe Formation in the western sichuan basin, China. Acta Geol. Sin. Engl. Ed. 84 (5), 1229–1238. doi:10.1111/j.1755-6724.2010.00293.x
Zeng, L. B., Qi, J. F., and Wang, Y. X. (2007). Origin type of tectonic fractures and geological conditions in low-permeability reservoirs. Acta Pet. Sin. 28 (4), 52–56.
Zeng, L. B., Su, H., Tang, X. M., Peng, Y. M., and Gong, L. (2013). Fractured tight sandstone oil and gas reservoirs: A new play type in the dongpu depression, bohai bay basin, China. AAPG Bull. 97 (3), 363–377. doi:10.1306/09121212057
Zeng, L. B., Zhao, X. Y., Zhu, S. J., and Zhao, J. Y. (2017). Waterflood-induced fractures and its signifcance for development of lowpermeability sandstone oil reservoirs. Petroleum Sci. Bulletion 2 (3), 336–343. doi:10.3969/j.issn.2096-1693.2017.03.031
Zhang, C., Zhu, D. Y., Luo, Q., Liu, L. F., Liu, D. D., Yan, L., et al. (2017). Major factors controlling fracture development in the Middle Permian Lucaogou Formation tight oil reservoir, Junggar Basin, NW China. J. Asian Earth Sci. 146, 279–295. doi:10.1016/j.jseaes.2017.04.032
Zhang, F., Jiang, Z. X., Xiao, H. M., Hu, B., Chen, P., Tang, X. L., et al. (2021). Testing origin of reservoir quality difference of tight sandstones in the Yanchang Formation, Ordos Basin, China. Mar. Petroleum Geol. 2021, 105507. doi:10.1016/j.marpetgeo.2021.105507
Zhao, W., Jia, C. Z., Jiang, L., Zhang, T., He, M. X., Zhang, F., et al. (2021). Fluid charging and hydrocarbon accumulation in the sweet spot, Ordos Basin, China. J. Petroleum Sci. Eng. 200, 108391. doi:10.1016/j.petrol.2021.108391
Keywords: tectonic fracture, diagenetic fracture, main controlling factor, tight oil reservoir, jimsar sag
Citation: Yu Y, Deng Z, Gao J, Li P and Zhang C (2023) Main controlling factors of natural fractures in tight reservoirs of the lucaogou formation in the jimsar sag, Xinjiang, China. Front. Earth Sci. 11:1170791. doi: 10.3389/feart.2023.1170791
Received: 21 February 2023; Accepted: 05 June 2023;
Published: 23 June 2023.
Edited by:
Hu Li, Southwest Petroleum University, ChinaReviewed by:
Wenming Ji, China University of Petroleum (East China), ChinaCopyright © 2023 Yu, Deng, Gao, Li and Zhang. This is an open-access article distributed under the terms of the Creative Commons Attribution License (CC BY). The use, distribution or reproduction in other forums is permitted, provided the original author(s) and the copyright owner(s) are credited and that the original publication in this journal is cited, in accordance with accepted academic practice. No use, distribution or reproduction is permitted which does not comply with these terms.
*Correspondence: Ze Deng, ZGVuZ3plQHBldHJvY2hpbmEuY29tLmNu; Jinliang Gao, amlubGlhbmcwMjA1QDEyNi5jb20=
Disclaimer: All claims expressed in this article are solely those of the authors and do not necessarily represent those of their affiliated organizations, or those of the publisher, the editors and the reviewers. Any product that may be evaluated in this article or claim that may be made by its manufacturer is not guaranteed or endorsed by the publisher.
Research integrity at Frontiers
Learn more about the work of our research integrity team to safeguard the quality of each article we publish.