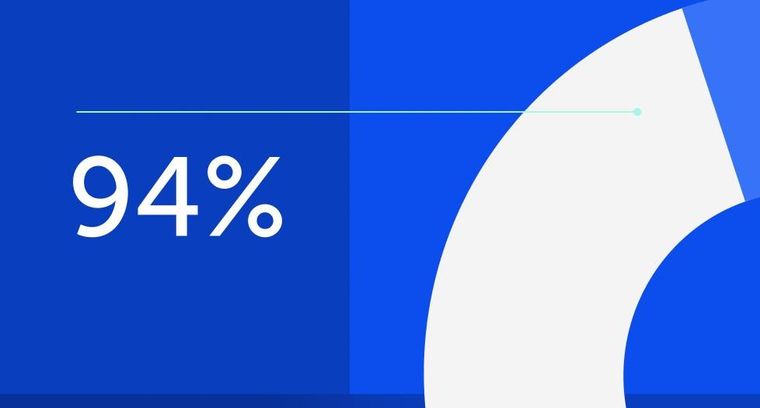
94% of researchers rate our articles as excellent or good
Learn more about the work of our research integrity team to safeguard the quality of each article we publish.
Find out more
ORIGINAL RESEARCH article
Front. Earth Sci., 17 May 2023
Sec. Economic Geology
Volume 11 - 2023 | https://doi.org/10.3389/feart.2023.1146126
This article is part of the Research TopicOrganic Matter Accumulation in Organic-Rich ShalesView all 11 articles
The Middle Magdalena Valley Basin (MMVB) in Colombia has a long history of conventional hydrocarbon exploration and production, with a cumulative production of 2.75 billion barrels of oil as of December 2021. Recent interest has been directed towards unconventional hydrocarbon plays within the basin due to the fine-grained nature of its Cretaceous source rocks and their mineralogy and mechanical properties. This study presents a three-dimensional basin model for three Upper Cretaceous source rocks, known as the “La Luna Formation,” within the MMVB. The model was developed using new data from five outcrops, 7,640 km of 2D seismic lines, and forty-one boreholes, as well as additional data sets such as X-ray diffraction analyses, pyrolysis analyses, well-log correlations, facies analysis, fracture pattern prediction, pore pressure analysis, heat flow estimations, and petrophysical data. The model estimates total retained oil and gas volumes to be 7.95 billion barrels and 4.21 trillion cubic feet in most probable scenarios, after a 15% recovery factor. Seismic interpretation reveals pre-Eocene structures beneath Paleogene and Neogene sediments, and the thickness variation of the source rocks from south to north of the basin. Petrophysical modeling shows effective porosities ranging from 2%–12% and organic porosities lower than 0.1%, with parts of the succession that might correspond to a carrier bed play. From a geomechanical perspective, we identified several brittle strata based on the higher concentrations of carbonates and quartz, and the areas with a higher probability of occurrence of natural fractures. Pore pressure analysis of multiple wells shows that wells in which the Middle Eocene unconformity has beveled the source rocks have no sealing capacity, becoming a risk for the play. The results suggest potential for unconventional hydrocarbon plays in the MMVB, with sweet spot areas being primarily controlled by porosity, thermal maturity, gas-oil ratios, and retained oil and gas volumes, as well as to a lesser degree, the probability of natural fractures and pore pressure conditions. However, further exploration is needed to constrain uncertainties regarding facies and source rock quality, particularly within the depocenters of the basin, in order to prove the economic feasibility of these unconventional plays.
The Middle Magdalena Valley Basin (MMVB) is located in Central Colombia and is the second most prolific hydrocarbons province of the country. Fine-grained Cretaceous rocks in northwestern South America and in the MMVB have been studied because of their importance as source rocks that feed conventional petroleum plays (e.g., Erlich et al., 2003; Rangel et al., 2017; Thompson-Butler et al., 2019). The chemistry, mineralogy, and mechanical properties of these rocks, however, have made them in more recent years the focus of attention as an unconventional play prospect (EIA, 2015). Yet-to find estimations of in-place and recoverable resources for the Cretaceous rocks within the MMVB as of December 2021 were 24.830 Tcf of gas and 7.787 Bbbl of oil (Mora et al., 2018; ANH-UPTC, 2021).
Despite the MMVB being an established hydrocarbon-producing basin, a thorough understanding of its full potential and the location of remaining undiscovered hydrocarbon resources requires a comprehensive evaluation of the petroleum system. Such an evaluation includes the determination of the total volume of hydrocarbons generated from each source rock, as well as the volumes of migrated and remaining hydrocarbons in or near the source beds for potential production. In the same way, previous interpretations of source-related biomarkers suggest the presence of an extensive petroleum system, although several questions to address around generation, migration, seal, and trap processes remain (e.g., Ramón et al., 1997; Rangel et al., 2017; Thompson-Butler et al., 2019).
The aim of this study is to analyze geological information from multiple sources, including outcrops, wells, wire-line logs, and source-rock geochemical data in order to: 1) determine rock properties; 2) develop a three-dimensional model depicting the regional basin architecture; and 3) use petroleum system analysis to evaluate the spatial distribution and petroleum potential of Upper Cretaceous source rocks across the MMVB, as unconventional reservoirs.
The MMVB is an intermontane basin located in the central-northern part of the Andes in Colombia. It separates the Central and Eastern cordilleras of the country (Figure 1). The basin has a long history dating back to the Mesozoic, which was shaped by the tectonic interactions between the South American, Farallon, and Caribbean plates. During this period, the Central Cordillera was a magmatic arc, and rifted sub-basins filled with marine sediments formed to the east, each with their own subsidence history (Bayona, 2018; Sarmiento-Rojas, 2019). Compressional deformation of the Central Cordillera and the MMVB began in the Late Cretaceous, resulting in several time gaps, including the Late Campanian hiatus (Cooney & Lorente, 1997; Bayona, 2018; Etayo-Serna, 2019a). In the Cenozoic, the basin began to fill with continental sedimentary rocks, which also partially covered the Central Cordillera with valley-fill deposits along an onlapping surface (Gomez et al., 2005; Caballero et al., 2013).
FIGURE 1. Location of the study area. Selected wells, composite seismic data, depocenters, and structural highs are also shown.
Figure 2 shows the sedimentary units which comprise the MMVB. The basal units of the Cretaceous include the continental-transitional strata of the Tambor and Cumbre formations (Berriasian-Valanginian), which are related to the rifting and opening stages of the basin. The so-called “Grupo Calcareo Basal” consists of the Rosa Blanca, La Paja, and Tablazo formations (Valanginian-Aptian) and shows the evolution of transitional to shallow marine calcareous environments. The Simiti Formation (Aptian-Albian) comprises shales and might correspond to a maximum base-level rise (Etayo-Serna, 2019b). The so-called “La Luna Formation” (Late Cenomanian-Campanian) consists of the Salada, Pujamana, Galembo, and La Renta formations related to thermal post-rift conditions. The Umir and Lisama formations (Maastrichtian-Early Paleocene) contain transitional and continental rocks corresponding to the Central Cordillera uplifting and basin closure. Finally, most of the Cenozoic fill consists of the La Paz, Esmeraldas, Mugrosa, Colorado formations, and the Real Group, related to the Eastern and Central Cordilleras uplifting.
FIGURE 2. Representative stratigraphic chart of the Middle Magdalena Valley Basin. Based on geologic descriptions, tectonic events, and petroleum system elements by Morales (1958), Ramón et al. (1997), Gomez et al. (2005), Thompson-Butler et al. (2019), and Etayo-Serna (2019a). Correlation with seismic reflection data of the study area and the interpreted seismic units are shown.
At least two intrabasinal highs divide the MMVB (Figure 1), corresponding to the La Cira-Infantas and Cachira highs (Gomez et al., 2003; 2005; Cortes et al., 2022; Reyes et al., 2022). The related depocenters to the La Cira-Infantas high are generally deeper (e.g., Nuevo Mundo-La Salina Depocenter) and contain a thicker and more complete Cretaceous-Cenozoic succession than those near the Cachira high (e.g., Santa Lucia Depocenter), which appears positive during the Oligocene (Horton et al., 2015).
The MMVB is host to a petroleum system that is composed by several elements, including source rocks, reservoirs and seals. The source rocks are found primarily in the Cretaceous formations, with the La Luna Formation being the most significant. The oils found in most Cenozoic reservoirs of the MMVB are genetically linked to the Salada, Pujamana, Galembo, and the La Renta formations (Turonian-Campanian) (Mora et al., 1996; Ramón et al., 1997; García et al., 2001; Rangel et al., 2017). Two intervals that correspond to the Cenomanian-Turonian sequence or the Salada Formation and the Coniacian-Campanian sequence that lithologically corresponds to the Galembo and La Renta formations, were deposited under anoxic conditions allowing large amounts of organic carbon to be deposited during this time (Villamil et al., 1999; Erlich et al., 2003). These intervals contain total organic carbon (TOC) greater than 4% and contain the best-quality source rocks, likely type B organofacies of Pepper & Corvi (1995). The Pujamana Formation of middle-late Turonian age on the other hand corresponds to organic-lean rocks deposited under oxic to disoxyc conditions and likely type C/D organofacies of Pepper & Corvi (1995), suggesting that these rocks have a lower hydrocarbons generation potential. Additionally, the La Paja-Tablazo interval (Barremian-Lower Albian) serves as source rock as it is proved by rock-oil correlations of lower Cretaceous rock extracts and oils found in Cretaceous and Cenozoic rock reservoirs of the MMVB (Rangel et al., 2017; Thompson-Butler et al., 2019). This interval was also deposited under anoxic conditions enhancing organic carbon accumulation and preservation (Gaona-Narvaez et al., 2013; Jimenez et al., 2015).
Finally, the main reservoirs in the MMVB are the La Paz and Mugrosa formation and, to a lesser extent, the Lisama and Umir formations. Also, commercial reservoirs exist in the Galembo-La Renta, Salada, and Rosa Blanca formations. In the same way, the Simiti, Umir, and Colorado formations are considered regional seals, although internal local seals are also present within the reservoirs.
This study presents an integration of a large-scale 2D seismic reflection dataset with borehole data, comprising of 7,640 km of 2D seismic data and forty-one wells, including lithological descriptions, biostratigraphic data, and velocity surveys, as well as electric logs and datasets of 1,500 X-Ray Diffraction samples (XRD), and 5,000 source rock geochemical data including vitrinite reflectance (%Ro), total organic matter (TOC), and pyrolysis. The subsurface data were primarily sourced from the Banco de Información Petrolera. The research follows a petroleum systems analysis workflow, comprising of seismic interpretation, petrophysical modeling, basin modeling, source-rock characterization, pore pressure analysis, and fracture systems characterization. The study aims to model hydrocarbon generation and predict the volumes of retained hydrocarbons, the Gas-Oil Ratio (GOR), porosity, thermal maturities in order to define sweetspots for unconventional exploration in the basin. The software used in this research include the Zetaware software suite, Techlog v2022, Petrel v2022, and Petromod v2022.
This study used three hundred 2D seismic-reflection lines in TWT to construct the regional structural framework, including the basement and key stratigraphic units. The data from thirty-five wells were tied using check-shot surveys, and data from neighboring wells were used to supplement any missing information.
The study also used lithological descriptions and stratigraphic ages from the boreholes to assign approximate ages to the marker horizons. Faults were also identified from seismic and well data. The time-to-depth conversion was done using interval velocities from check-shot surveys and synthetic seismograms. Quality control was performed by checking the seismic marker horizons against well tops from logs not initially involved in the interpretation, and isopach maps were created to provide a regional overview on the location of the depocenters and main structural features.
The depth structural maps obtained from the seismic interpretation were integrated into the basin modeling, and also for the analysis of strain and fractures on structural surfaces. This analysis employed the methodology proposed by Suo et al. (2012), which posits that the density and orientation of fractures are related to the curvature of a structural surface. Specifically, it states that as the curvature of a structure increases, the number of fractures also increases.
Forty-one wells with triple-combo logs were evaluated to obtain petrophysical properties, including lithology, porosity, and permeability. Total organic carbon (TOC) content was estimated using mathematical models based on well-log measurements. For instance, TOC content was estimated from uranium concentrations as measured from spectral gamma ray, which involves a model based on the relationship between the uranium concentration and TOC (Fertl and Rieke, 1980). Correlations to bulk density measurements were also used, using a model that relates the bulk density of the formation to TOC (Schmoker & Hester, 1983). In addition, the mathematical model which describes the correspondence of the compressional transit time with deep resistivity (Delta log R method), was also used as a related parameter to TOC (Passey et al., 1990). Finally, the validity of the values derived from petrophysical calculations was assessed by comparison to TOC contents determined via Rock-Eval pyrolysis or LECO carbon analyzer.
A probabilistic multimineral model was applied (i.e., Quanti-Elan ®) to determine rock mineralogy and clay volumes. The Quanti-Elan model uses well logs (e.g., density, photoelectric factor, sonic, neutron, gamma ray), XRD and TOC datasets to quantify the different minerals such as quartz, carbonates, phosphates, pyrite, and clays, applying multivariate statistical methods and providing a range of probabilities for each estimated mineral type rather than a deterministic answer (Ali et al., 2022). The multimineral model and the TOC estimation are necessary to calculate effective porosity, and organic porosity. We applied Galford et al. (2013) and Alfred & Vernik (2013) methods for organic porosity estimation, constrained with pyrolysis datasets, which measure the pore spaces created by thermal maturation and conversion of the organic matter into hydrocarbons within source-rock reservoirs.
The brittleness, or ability of a rock to fracture, is a valuable criterion in predicting enhanced productive intervals or areas. The Upper Cretaceous rocks were characterized based on a mineral-based brittleness index, proposed by Wang & Gale (2009), including borehole and outcrop data. Finally, evaluation of the geopressures on most of the wells were carried out establish the presence and distribution of overpressures in the basin, integrating drilling mud-weight data and well logs following Grauls' (1999) method, in order to evaluate the sealing risks for unconventionals prospectivity in the basin.
We used one-dimensional (1D) and three-dimensional (3D) models to understand how rock properties in the basin changed over time and to identify the best areas for potential production from source-rock reservoirs (sweet spots). Forty-one 1D models in the MMVB were made to evaluate changes in source-rock properties, geohistory, and thermal maturity trends during the basin evolution. These results were combined and used to calibrate a 3D geological model of the Cretaceous-Cenozoic succession to create a 3D petroleum systems model.
Subsidence analysis helps to account for how the rocks in a basin have been buried or heated over time and how deformation has affected the basin structure (e.g., Hantschel and Kauerauf, 2009). We used subsidence analysis (backstripping) to reconstruct the basin’s evolution from the Cretaceous to the present day, considering sediment compaction and variations in paleobathymetry or paleotopography (Allen & Allen, 2013). From seismic interpretation and subsidence curves, erosion maps were estimated to include the eroded thicknesses of the stratigraphic units in the basin modeling Stratigraphic names, ages, unconformities, and time gaps correspond to Morales (1958), Gomez et al. (2005), Caballero et al. (2013), Sarmiento-Rojas (2019), Bayona (2018) and Etayo et al. (2019a) proposals.
We applied inverse modeling to the regional burial history model, allowing for the reconstruction of the basin time-temperature history. Inverse modeling involves using observed data to infer the underlying parameters of a geological model. For example, the use of present-day surface temperatures, paleolatitude and paleoglobal mean surface datasets, and crustal models. Based on average yearly temperature observations from the IDEAM (2022), the calculations of present-day surface temperatures are around 26 °C. To estimate paleo-temperatures, as described by Beardsmore & Cull (2001), we included paleolatitude and paleoglobal mean surface temperature datasets (e.g., Scotese et al., 1988). Also, we used crustal models to estimate the lower thermal boundary conditions of the sedimentary basin at the present day and through time. The Pre-Cretaceous basement structure is based on the Barrera-Lopez et al. (2022) model, which proposed a crustal thickness map based on geophysical and geomorphological data for northern South America, allowing us to use an average total lithospheric thickness of 120 km (to the 1,330°C isotherm). In the same way, a transient heat flow was considered to estimate the heat flow in the study area, because heat flow affects thermal maturation of the source rocks and thus the generation of hydrocarbons (e.g., Beardsmore & Cull, 2001). We also considered the role of lithospheric extension in the basin’s thermal history, which only had a minor influence, since modeled crustal extension factors from wells were consistent with Early Cretaceous β values close 1.2 (Sarmiento-Rojas et al., 2001).
Forward modeling is a valuable method to address the physical properties of a basin involving the simulation of various scenarios to generate geological parameters (e.g., density, porosity, velocity) and iteratively adjusting the input parameters of the simulation until the simulated results match the observed data as closely as possible, also evaluating the reliability of the model. Lithologies for each formation were estimated based on individual wire-line log data analysis, cuttings, core descriptions, and well completion reports applying inverse modeling. Also, the lithologies and burial history were calibrated with density and/or sonic logs, and the effective porosity calculation from petrophysics. (Figure 3). The thermal histories of all 1-D models were calibrated using present-day corrected temperature data (e.g., Holgate and Gerner, 2010) and maturity indicators, including vitrinite reflectance (Ro) and Tmax. The %Ro suppression within MMVB sediments was not apparent in the available data as other authors previously mentioned (e.g., García-Gonzalez & Carvajal, 2006), and it is possible to have measurements in solid bitumen which require proper conversions to equivalent Ro (Juliao-Lemus et al., 2015), to calculate accurate paleotemperatures.
FIGURE 3. Example of forward modeling with well log data calibration for compaction, thermal and geochemical parameters for 1D models in the study area.
The 3D petroleum systems model for the Salada, Pujamana, Galembo and La Renta Formations also includes the estimation of the source-rock properties such as quantity and quality of the organic matter. These formations are formed up of a combination of lithologies, nomenclature broadly explained in Terraza-Melo (2019), including: phosphorites (TOC 2-3 wt%), mudstones (TOC 0.5–3 wt%), shales (TOC 0.5-1 wt%), and wackestones (TOC 0.5–3 wt%), which together create a continuum of possible source rocks with TOC values ranging from less than 1 to greater than 7 wt% (Figure 4). The hydrogen index (IH) of these rocks’ ranges from 150 mg HC/g TOC to 700 mg HC/g TOC and its organofacies are described below (Section 3.4) (Figure 4).
FIGURE 4. Plot of pyrolysis temperature (Tmax) versus hydrogen index (HI) and total organic content (TOC) for the Salada, Pujamana, Galembo and La Renta formations in the study area, that shows present-day kerogen quality and thermal maturity stages.
As additional inputs to the petroleum systems model, we used an updated dataset for the MMVB, including open file TOC, rock-eval pyrolysis, and Ro data (e.g., Aguilera et al., 2010), the TOC and hydrogen index (HI) to determine properties for each source rock (Figure 4). Using measured maturity data and the suitable kinetics based on the kerogen type, as mentioned in the following section, the HIo values—which indicate the original HI of the source rock before the start of kerogen transformation—were approximated from current values.
Modeling the kinetics of kerogen pyrolysis approximates the thermal decomposition of organic matter in source rocks, which is a key process in the generation of hydrocarbons (Pepper & Corvi, 1995). Using pyrolysis measurements, Aguilera (2022) created source-rock kinetics specific to the Cretaceous source rocks of the MMVB, using the non-linear regressions for different kerogens in Canadian basins proposed by Cheng & Jiang (2015). Most of the organic matter in the Galembo-La Renta, Pujamana, and Salada is marine, following Pepper and Corvi’s (1995) organofacies type B, and only a minor fraction is terrigenous non-marine, under Pepper & Corvi’s (1995) organofacies types D and E.
In order to model the volume of hydrocarbons expelled per unit area, additional data on rock density and sorption capacity were needed. The density of the inorganic rock matrix, the amount of organic matter, and the fluid contained in the pore spaces all influence bulk density, which in turn, depends on both organic and inorganic porosity. In this study, average values calculated from petrophysical estimations were applied for all three source rocks modeled. Also, adsorption models were used to describe the mass of hydrocarbons released into the free pore space of the source rock.
The seismic succession was subdivided into eleven (11) seismic units, from the pre-Cretaceous basement to the Colorado Formation (Figure 2). The succession above the pre-Cretaceous basement is thickening southward and eastward, reaching its maximum thickness of 5.0 s TWT (ca. 7,200 m) within the Nuevo Mundo–La Salina Depocenter (NM-LSD) and 4.5 s TWT (ca. 4,800 m) within the Santa Lucia Depocenter (SLD). Northward and westward, the succession thins to a minimum thickness of 0.5 s TWT (ca. 300 m), where the basement forms the so-called Chimichagua Platform (Reyes et al., 2004), which separates the Lower Magdalena Valley Basin. The seismic units are interpreted to be deposited between the Cretaceous and Neogene based on biostratigraphic data from outcrops and boreholes (Figure 2).
The 2D seismic profiles (e.g., Figures 5–7) show pre-Eocene structures beneath the Paleogene and Neogene sediments. Most of these structures appear to be beveled and eroded before the deposition of Eocene sediments (e.g., La Cira-Infantas High, Figure 5). Also, it preserves high-angle reverse faults and east-verging fault-propagation folds cored by the basement (e.g., Figure 6). The Salina Fault System forms the western boundary of the NM-LSD (e.g., Figure 5) and suggests pre-Andean deformation related to early phases of uplifting of the Eastern Cordillera (Sarmiento-Rojas et al., 2001). Also, the seismic profiles show a progressive onlap of Cenozoic units onto the Central Cordillera basement. The timing of the Central Cordillera’s uplift is evidenced in the Morales Monocline (MM) and the Cachira High (CH), which appears to occur before the Paleocene, our observations are consistent with several proposals for the study area (e.g., Suarez et al., 2000; Gomez et al., 2003; Parra et al., 2012; Cortes et al., 2022).
FIGURE 5. Composite seismic line traversing the Middle Magdalena Valley Basin (location in Figure 1). (A) Uninterpreted seismic line tied with wells. (B) Interpreted composite seismic line highlighting the Infantas High and the La Salina-Nuevo Mundo Depocenter.
FIGURE 6. Composite seismic line traversing the Middle Magdalena Valley Basin (location in Figure 1). (A) Uninterpreted seismic line tied with wells. (B) Interpreted composite seismic line highlighting the Cimitarra Fault and and the northern part of the La Salina-Nuevo Mundo Depocenter.
FIGURE 7. Composite seismic line traversing the Middle Magdalena Valley Basin (location in Figure 1). (A) Uninterpreted seismic line tied with wells. (B) Interpreted composite seismic line highlighting the Morales Monocline and the Totumal Field.
Figure 8 shows selected structural and isopach maps of the Galembo, Pujamana, and Salada formations. This allows us to propose three structural domains in the MMVB. To the south, the structural domain is evidenced by the presence of reactivated north-south oriented inversion faults associated with the uplift of the Eastern Cordillera, which includes oil fields such as La Cira-Infantas, Lisama, and Provincia. In the central domain, there is evidence of northeast-southwest transpressive faults associated with structural inversion, including oil fields such as Casabe, Llanito, Cantagallo, Garzas, and Cristalina. Finally, in the northern domain, there is evidence of strike-slip faults associated with the uplift of the Central Cordillera, including oil fields such as Doña Maria, Totumal, Ayombero, and Crisol. The isopach maps reveal that the Pujamana and Salada Formations are thicker in the southern and central domains than in the northern domain. However, the thickness of the Galembo Formation increases in the northern domain and could be related to increasing accommodation space due to the uplifting of the Central Cordillera.
FIGURE 8. Depth contour and isopach maps (in feet) of the Salada, Pujamana and Galembo formations in the Middle Magdalena Valley Basin. The maps show the thickness trends of the source rocks from the seismic interpretation as well as the main depocenters in the study area.
García-Delgado & Velandia (2020) studied the tectonic geomorphology of the northern part of the Central Cordillera, the San Lucas Range, proposing two regional domains related to the Palestina Fault. Our results agree with their model since interpreted seismic reveals that most of the faults in the Central Cordillera could be mapped on the subsurface of the MMVB and have right-lateral kinematics (e.g., Cimitarra and Las Brisas faults). Also, our model is an alternative to the proposal of Guerrero et al. (2021), who considers the Cimitarra and Casabe faults as structures related to the Eastern Cordillera.
The best production values in several worldwide basins (e.g., Western Gulf Basin) are in areas with high natural fractures (Ramirez & Aguilera, 2014). Figure 9 presents the results of the curvature analysis for the Galembo, Pujamana, and Salada Formations. The maps reveal areas with higher and lower probability of the existence of natural fracture systems. The NM-LSD and the Cachira High have areas with a higher probability of natural fractures. For example, the Chuira field near the Cachira High has 16% porosity related to natural fracture systems (Acevedo et al., 2012a). On the other hand, the Morales Monocline, the San Fernando Depocenter, and the La Cira-Infantas High are areas with a lower probability of naturally induced fractures.
FIGURE 9. Probability of occurrence of natural fractures maps of the Salada, Pujamana, and Galembo formations in the Middle Magdalena Valley Basin.
The petrophysical modeling allows us to suggest the Galembo, Pujamana, and Salada Formations’ lithology based on quartz-feldspar-mica, carbonate, and clay content (Figure 10). We mapped these distributions to assess the lateral variations of the source rocks. Notably, the Salada Formation has carbonate contents in areas neighboring the SLD and the La Cira-Infantas High between 0.5 v/v and 1.0 v/v. This contrasts with the carbonate content of the Pujamana Formation, which in all study area is below 0.3 v/v, with small areas of the Chimichagua Platform containing values of 0.5 v/v. The Galembo Formation has carbonate contents ranging between 0.3 v/v and 0.5 v/v. For all three units, neighboring areas around the San Fernando Depocenter contain quartz-feldspar-mica contents between 0.4 v/v and 0.7 v/v. Also, the Galembo and Pujamana Formations have quartz-feldspar-mica contents between 0.4 v/v and 0.6 v/v in the SLD. Finally, the Pujamana Formation has the highest contents of clays, between 0.4 v/v and 0.7 v/v in most of the study area. As an alternate petrophysical mineral model, Rojas et al. (2013) applied conventional models, which include the apparent grain density (DGA), and the apparent volumetric photoelectric factor (UMA), obtaining results consistent with our proposal, despite having a limited well log and XRD dataset.
FIGURE 10. Quartz-Feldspar-Micas (QFM), clays and carbonates content maps from petrophysical modeling of the Salada, Pujamana, and Galembo formations in the Middle Magdalena Valley Basin.
In the same way, Figure 11 presents XRD datasets plotted in a ternary diagram (Gamero-Diaz et al., 2013) of quartz-feldspar-mica, clay, and carbonates content, comparing the MMVB source rocks with worldwide shale plays from Bromhead et al. (2017) and Mews et al. (2019). Petrographic data suggest that most of the quartz in this source rocks is related to diagenetic processes rather than its depositional environment (e.g., Acevedo et al., 2012a; Galvis-Portilla et al., 2014). In all cases, clays content is lower than 40%, suggesting, that most of the units have an excellent brittleness quality which might help in future completion designs, also agreeing previously published petrophysical models which proposes at least two landing zones within the Salada and Galembo formations (e.g., Piedrahita and Aguilera, 2017a; Veiga et al., 2023). Perez et al. (2014) presented an XRD dataset of the La Luna-1 well, showing the composition of clay minerals. Their results show that illite and interstratified species compose the bulk of the clay minerals and have lesser proportions of kaolinite. However, future research in other boreholes of the basin is required to understand the composition of clay minerals and unveil their depositional and diagenetic history.
FIGURE 11. Ternary diagram of QFM, carbonates and clays content from XRD datasets of the Salada, Pujamana, Galembo and La Renta formations in the Middle Magdalena Valley Basin, and its comparison with worldwide shale plays.
The effective porosity in the studied source rocks ranges between 2% to 12%. The La Renta Formation has the higher porosities which range between 5% to 12%. Considering this, the La Renta Formation is a probable carrier bed, despite not being modeled as an independent source rock from the Galembo Formation. We estimated TOC and organic porosity from well logs, revealing that organic porosity values range from 0.0006 v/v to 0.002 v/v for all three formations, implying low levels of organic matter conversion (Jarvie, 2014). When compared to worldwide shale plays (e.g., Barnett Shale), the estimations for the MMVB are quite low, because organic porosity values range from 0.01 v/v to 0.04 v/v (Romero-Sarmiento et al., 2013). However, our results are comparable with those obtained by Piedrahita & Aguilera (2017a, 2017b) in the study area, who used different well log estimations to obtain kerogen density and organic porosity. Also, our results match those obtained by Aguilera (2022), who applied Chen & Jiang’s (2016) methodology for organic porosity estimations from pyrolysis datasets, and to Ceron et al. (2013) estimations using digital rock physics. Finally, should be expected higher organic porosity values in deeper parts of the study area.
The overpressure increases the production drive of liquid hydrocarbons and favors higher production rates (Gong & Rodriguez, 2017) but also is a sign of sealing integrity and capacity. As a first approach, the pore pressure analysis helps screen overpressure intervals and allows us to map the distribution of areas with low pore pressure, which might become a risk for the source-rock play (see Section 5.1). We observed two areas with low pore pressure conditions: First, areas with structures beveled by unconformities (e.g., La Cira-Infantas High), and second, areas with low overburden (e.g., Morales Monocline). Our results are consistent with those obtained by Vargas-Silva et al. (2019), revealing that factors such as water expansion have a negligible effect on the pore pressure in the basin. Although thermal stress and oil and gas generation have limited influence on the pore pressure estimation at the wells, with most of the effect related to compaction pressure, its effects could increase overpressure in deeper areas and be observed in the predicted GOR (see Section 4.5).
Progressive burial of the strata during the basin infill process promotes physical changes in rock properties, reducing their porosity and permeabilities in contrast to increasing density, velocity, and heat capacity as the rock is buried and compacted by sediments. Athy’s Law (Allen & Allen, 2013) explains these processes and allows empirical calculation based on porosity reduction as burial increases. In this way, the burial models of the wells were calibrated by comparing the porosity, velocity, and density profiles measured from well-logs and laboratory data to predicted data by modeling (Figure 3). As a result, burial modeling suggests at least two significant episodes of generation of accommodation space, which extend throughout the Cretaceous and from the Oligocene to the present day. In contrast, a major episode of erosion is recorded from the final part of the Late Cretaceous until the Eocene, widely observed using seismic lines and outcrop data (e.g., Section 4.1). From the Berriasian through the Hauterivian, the basin records an increased rate of tectonic subsidence (Tambor and Rosa Blanca formations). The La Paja and Tablazo formations, which span the Barremian to Aptian, undergo a low tectonic subsidence rate. The Simiti, Salada, Pujamana, Galembo-La Renta, and Umir formations, which range from the Albian to the Maastrichtian, show an increase in the basin’s tectonic subsidence rate. Although the highest subsidence rates are recorded in the southern province (e.g., La Salina-Nuevo Mundo Depocenter), the central and northern provinces have areas with high subsidence rates (e.g., Santa Lucia Depocenter).
The heat flow modeling allows us to identify three stages of the basal heat flow history consistent with the major tectonic episodes described above, reflecting burial history (Figure 12). As an alternative to the heat flow model published by Gonzalez et al. (2020), during the Early Cretaceous, the basin setting could be explained with basal heat flow values below 60 mW/m2. Likewise, the history of basal heat flow is consistent with the subsidence history of the basin proposed by authors such as Sarmiento-Rojas et al. (2001), who suggest that the stretching factors (β) for the basin are very low (≈1.2).
FIGURE 12. Basal heat flow variation along time from forty-one 1D models in the Middle Magdalena Valley Basin. Three main tectonic events in the basin are shown.
Figure 13 compares the transformation ratio and the modeled thermal maturity. The source-rock transformation ratios are quite different in the SLD and NM-LSD. The highest transformation ratios were reached in the NM-LSD, ranging between 70% to 100% (wet-gas window to overmature) for the Galembo, Pujamana, and Salada Formations. In the SLD, the highest transformation ratios range between 50% to 80% (peak-oil to the wet-gas window). The variation in the transformation ratio between depocenters is controlled by the maximum burial depth. However, the number of boreholes drilled in the depocenters is low to compare the transformation ratios’ variations properly. In the same way, there is a need for more data to evaluate the effects of crustal radiogenic heat production and the conductivity of coals within the Cenozoic succession, and we hope future research assesses it.
FIGURE 13. Thermal maturity (%Ro) and transformation ratio maps of the Salada, Pujamana and Galembo formations in the Middle Magdalena Valley Basin.
The estimation of generated and retained hydrocarbons from the different source rocks is based on a volumetric material balance controlled by the kinetics of the source rock model used, in this case Pepper and Corvi’s (1995) organofacies models, according to its kerogen type and thermal maturity levels reached during the maturation process. From these models an estimation of the hydrocarbons, generated and expelled, is made and a retained volume of hydrocarbons is obtained (liquid and gas phases), volume that is controlled by the organic porosity wettability, adsorption and absorption of the rock matrix, and the progressive transformation of kerogen into hydrocarbons with increasing temperature.
The total generation potential of all modeled Upper Cretaceous rocks in the MMVB is approximately 163.63 billion BOE (Table 1). Critical factors affecting total generation potential include source-rock quality and thermal maturity. The extent of the Upper Cretaceous generation pod is consistent with the location of major conventional fields (Figure 14).
FIGURE 14. Gas-oil ratio (GOR), oil total retained, gas total retained maps of the Salada, Pujamana and Galembo formations in the Middle Magdalena Valley Basin.
Determining the relative hydrocarbon quantities expelled by each source rock depends on several other critical variables, including organofacies. For example, Aguilera (2022) observed similar HIo values between Lower and Upper Cretaceous rocks (HIo 600–750 mg HC/g TOC). However, Lower Cretaceous seem to have affinity with lower quality Pepper and Corvi’ (1995) B/D/E organofacies. The Upper Cretaceous rocks have a lower maturity trend and organofacies with a lesser proportion of terrigenous organic matter, which increases the volume of hydrocarbons expelled compared with the Lower Cretaceous source rocks.
The thermal maturity influences the total hydrocarbons generated because the Galembo, Pujamana, and Salada formations reached higher transformation ratios in the depocenters than in less mature margins of the basin. Although hydrocarbon generation began in the Late Cretaceous, most of the generation occurred in the Neogene, coincident with the maximum burial depth as earlier studies suggest (e.g., Gonzalez et al., 2020).
The basin modeling total liquid and gas retained from the Upper Cretaceous source rocks of the MMVB are estimated at 7.95 Bbbl and 4.21 Tcf, after a 15% recovery factor respectively. Most liquids and gas retained are in the Galembo and Salada Formations rather than the Pujamana Formation (Figure 14). Although the results from this study are comparable with those from Mora et al. (2018), with modeled volumes of a similar order of magnitude, their proposal considers the Pujamana Formation with the higher total gas retained.
The predicted GOR values range between 10–3,780 scf/bbl, suggesting that the expelled products will be primarily light oil in areas below 75% transformation ratios and gas condensates in areas with higher transformation ratios (Figure 14). Despite no unconventional well test data, conventional production data help to check the consistency of the predicted GOR. For example, oil fields neighboring the Cachira High (e.g., Totumal field) produced 130 barrels from the Galembo Formation in initial tests, but its production quickly declined. Consistent with the GOR values of approximately 600 sfc/bbl predicted by the basin modeling for such interval in that part of the basin.
The volumes of hydrocarbons retained within the source rocks suggests good potential for shale oil and gas plays. Therefore, the predicted hydrocarbon type is light oil, with some gas condensate in areas of high thermal maturity. This result is consistent with the oil and gas shows reported during drilling in most wells.
Several authors mentioned solid bitumen remnants and extensive asphaltites within the Upper Cretaceous rocks’ outcrops of the study area and boreholes drilled near the Cachira High (e.g., Acevedo et al., 2012b). A plausible explanation invokes the generation of solid bitumen in the early stages of hydrocarbon generation (Juliao-Lemus et al., 2015). However, future source-rock correlations should answer this question, although the possibility of asphaltite accumulations from hydrocarbons generated in the Lower Cretaceous source rocks cannot be ruled out.
Each basin’s geology is different, and its conditions should be evaluated to propose a sweet spot. In this context, we define a sweet spot as a geographic area with expected high production values surrounded by areas with lower production values, following Shurr & Ridgley (2002) definition. This proposal differs from the sweet spot considered from the net-pay at a reservoir scale (e.g., Slatt et al., 2015) or economically viable areas.
After data integration and interpretation, we suggest that the sweet spot areas in the MMVB for the Galembo, Pujamana and Salada Formations are driven by: 1. The effective porosity. 2. The transformation ratio. 3. The total retained gas and liquid hydrocarbons. 4. The gas-oil ratio. We propose the following cut-off values to rank and reclassify each map into good, fair, or low (Table 2).
As presented before (see Section 4.1, 4.2), the mineralogical brittleness is constrained by the facies distribution, considering that all source rocks were deposited under mixed-ramp conditions, with low deposition of clay minerals. On the other hand, the thickness variation of the source rocks along the basin remains quite constant, despite areas with higher thicknesses (see Section 4.1). The probability of natural fractures (see Section 4.1), considered a criterion that would account in combination with the sweet spot maps, helping to screen areas that could be produced under conventional conditions. These criteria are not deemed as factors for a definition of an unconventional sweet spot in the MMVB at a regional scale, because they are related to more local effects related to sedimentological heterogeneity and structural deformation within the basin. Organic porosity is another geologic criterion that does not drive the sweet spot definition and is included in the effective porosity. The basin modeling and petrophysical data show that thermal maturity in the areas outside the depocenters, where most of the wells have been drilled so far in the basin, is too low to develop significant organic porosity systems (see Section 4.2).
However, published results of mercury intrusion porosimetry reveal discrete intervals within the Salada and Galembo Formations, with pore volume constituted by 0.2–5.5 nm pore sizes (Juliao-Lemus et al., 2015; Casadiego-Quintero & Rios-Reyes, 2020), that could be produced under more conventional completions (Baek & Akkutlu, 2019).
Considering geologic constraints, we present three sweet-spot maps for the Galembo, Pujamana, and Salada formations (Figure 15). Contours in maps represent depth contours from each stratigraphic surface, and hatched polygons are areas with an additional risk due to low pore pressure conditions (see Section 4.2). Also, when combined with curvature analysis maps, the sweet-spot maps highlight areas that could be produced under unconventional completions and improve conventional completions. The green areas represent a good sweet-spot area, the yellow and red areas represent fair and no sweet-spot areas, respectively.
FIGURE 15. Sweet spot areas maps of the Salada, Pujamana, and Galembo formations in the Middle Magdalena Valley Basin. Low pore pressure areas and structural depth contours (in feet) are also shown.
These maps help delineate areas representing shale oil and shale gas plays. Until now, only the Picoplata-1 well was drilled in the SLD reached the Salada Formation with promising results, but its tests were conventional. Although the La Renta Formation was not included in the sweet-spot maps, future research is required to confirm if it could be considered as a carrier bed play. Future drilling in the depocenters is required to confirm the modeling results and to address its uncertainties.
This study used petroleum systems analysis to provide a regional framework for understanding unconventional hydrocarbon prospectivity of the Upper Cretaceous source rocks in the MMVB. Results have allowed to assess the spatial distribution and petroleum generation potential of Upper Cretaceous source rocks across the basin, and the likely volumes of both expelled and retained hydrocarbons. The deterministic petroleum systems model estimated the volumes of retained hydrocarbons at 7.95 Bbls of oil and 4.21 Tcf of gas after a 15% recovery factor. The combined volumes of hydrocarbons generated from the Upper Cretaceous are mapped, highlighting the broad extent of the generation pods and their consistency with the location of major conventional fields across the basin. Source rocks within the Salada and Galembo formations are the biggest contributors to both expelled and retained hydrocarbons because these are the richest, thickest, and most extensive source facies, with good to excellent potential across the entire basin. In contrast, the hydrocarbon volumes generated by the Pujamana Formation are low because its shale facies have source rocks of lower quality.
The three sweet-spot maps for the Upper Cretaceous source rocks show areas with good potential for shale oil and shale gas to be tested. In addition, the development of these resources in such areas could contribute to the energy transition of the region. However, the distribution of the sweet-spot areas reveals that the depocenters are still to be drilled to better constrain uncertainties regarding the facies and source rock quality, and to prove the economic feasibility of the unconventional play in the MMVB.
The raw data supporting the conclusion of this article will be made available by the authors, without undue reservation.
Project design, definition of the study area and objectives: RA, OR-B, MP-R, and AP-C. Data collection: AP-C and JT. Data curation: AP-C, RA, and JT. Data interpretation and analysis of the results: All authors. Draft manuscript preparation: AP-C. All authors reviewed the results and approved the final version of the manuscript. Funding: OR-B, HF, HB and MR.
This research was funded by the National Hydrocarbon Agency (ANH) and the Colombian Geological Survey (SGC) through an inter-administrative agreement “Convenio 048 del 2021, ANH-SGC”.
We are grateful to the Hydrocarbons Division and the Editorial Board of the Colombian Geological Survey for their continuous support during the preparation of this manuscript. As part of an integral project that has involved several disciplines, we thank Cristian Benavides, Hellman Pinilla, Camilo Davila, Edgar Arciniegas, Hernando Mahecha, Laura Roman, Dayra Saenz, Sebastian Hernandez, Ruth Robayo, Johan Sierra and John Ortiz for their valuable contributions. Special thanks to Luis Quiroz, Juan Carlos Ruiz, Lucila Morales, German Pardo, Juan Sebastian Eslava, Karen Rodriguez, Xiomara Prince, Daniel Rojas, Andrea Pablos, Sergio Amaya, Alberto García, Juan Manuel Herrera and Daniela Carrera, who contributed to this research during its early stages. We would like to express our sincere gratitude to the anonymous reviewers for their valuable comments and feedback.
The authors declare that the research was conducted in the absence of any commercial or financial relationships that could be construed as a potential conflict of interest.
All claims expressed in this article are solely those of the authors and do not necessarily represent those of their affiliated organizations, or those of the publisher, the editors and the reviewers. Any product that may be evaluated in this article, or claim that may be made by its manufacturer, is not guaranteed or endorsed by the publisher.
Acevedo, R., Daza, D., Prince, M., Rojas, D., and Sanchez, C. (2012a). An integrated approach to the exploration of fractured reservoirs: A challenge in the cretaceous rocks of the middle Magdalena Valley Basin, Colombia. XI Simp. Boliv. Exploración Pet. las Cuencas Subandinas. doi:10.3997/2214-4609-pdb.330.43
Acevedo, R., Daza, D., Prince, M., Rojas, D., and Sanchez, C. (2012b). Presencia de Gilsonita y bitumen en la cuenca Valle Medio del Magdalena: Un reto para la perforación exploratoria. XI Simp. Boliv. Exploración Pet. las Cuencas Subandinas. doi:10.3997/2214-4609-pdb.330.164
Agencia Nacional de Hidrocarburos (Anh) & Universidad Pedagógica y Tecnológica de Colombia (Uptc), (2021). Geologic integration, petroleum system evaluation and prospectivity of Colombian frontier basins: Northern middle Magdalena and cesar-ranchería. Technical presentation https://www.anh.gov.co/documents/3688/UPTC_ANH_VMMN_CR_GEOLOGICAL_INTEGRATION_FEB_11_2022.pdf.Retrieved from:
Aguilera, R. C., Sotelo, V. A., Burgos, C. A., Arce, C., Gómez, C., Mojica, J., et al. (2010). Organic geochemistry atlas of Colombia. Earth Sci. Res. J. 14, 61–77.
Aguilera, R. (2022). “Data-driven kerogen kinetics from rock-eval data of the middle Magdalena Valley Basin, Colombia,” in Implications on the prospectivity of unconventionals AAPG International Conference and Exhibition, Cartagena, Colombia, March, 2022.
Alfred, D., and Vernik, L. (2013). A new petrophysical model for organic shales. Petrophysics 54, 240–247. SPWLA-2013-v54n3-A4.
Ali, J., Ashraf, U., Anees, A., Peng, S., Umar, M., Thanh, H., et al. (2022). Hydrocarbon potential assessment of carbonate-bearing sediments in a meyal oil field, Pakistan: Insights from logging data using machine learning and Quanti elan modeling. ACS Omega 7 (43), 39375–39395. doi:10.1021/acsomega.2c05759
Allen, A. A., and Allen, R. A. (2013). Basin analysis: Principles and applications. 3. New Jersey, United States: Wiley-Blackwell Publishing, 632. 978-0-470-67377-5.
Baek, S., and Akkutlu, Y. (2019). Produced-fluid composition redistribution in source rocks for hydrocarbon-in-place and thermodynamic recovery calculations. SPE J. 24, 1395–1414. doi:10.2118/195578-PA
Barrera-Lopez, C., Mooney, W., and Kaban, M. (2022). Regional geophysics of the caribbean and northern South America: Implications for tectonics. Geochem. Geophys. Geosystems 23, e2021GC010112. doi:10.1029/2021GC010112
Bayona, G. (2018). El inicio de la emergencia en los Andes del norte: Una perspectiva a partir del registro tectónico-sedimentológico del coniaciano al Paleoceno. Rev. Acad. Colomb. Ciencias Exactas Físicas Nat. 42 (165), 364–378. doi:10.18257/raccefyn.632
Beardsmore, G. R., and Cull, J. P-. (2001). Crustal heat flow: A guide to measurement and modelling. Cambridge, United Kingdom: Cambridge University Press, 324.
Bromhead, A., Sutcliffe, O., Hay, D., and Evans, K. (2017). “Lessons learned from the vaca muerta: An exploration model to aid sweet-spot prediction in the frontier hanifa unconventional resource play in the Middle East,” in Paper presented at the SPE/AAPG/SEG Unconventional Resources Technology Conference, Austin, United States, July, 2018.
Caballero, V., Mora, A., Quintero, I., Blanco, V., Parra, M., Rojas, L. E., et al. (2013). “Tectonic controls on sedimentation in an intermontane hinterland basin adjacent to inversion structures: The Nuevo Mundo syncline, Middle Magdalena Valley, Colombia,” in Thick-skin-dominated orogens: From initial inversion to full accretion. Editors M. Nemcok, A. Mora, and J. Cosgrove (London, United Kingdom: Geological Society, London, Special Publications), 377, 315–342.1.
Casadiego-Quintero, E., and Rios-Reyes, C. A. (2020). Pore structure characteristics and gas storage potential of the cretaceous La Luna Formation, middle Magdalena Valley Basin, Colombia. Ing. Cienc. 16 (31), 169–205. doi:10.17230/ingciencia.16.31.8
Ceron, M., Walls, J., and Diaz, E. (2013). “Comparison of reservoir quality from La Luna, gacheta and eagle ford shale formations using digital rock physics,” in Presented at AAPG International Conference and Exhibition, Cartagena, Colombia, June, 2013.
Chen, Z., and Jiang, C. (2015). A data driven model for studying kerogen kinetics with application examples from Canadian sedimentary basins. Mar. Petroleum Geol. 67, 795–803. doi:10.1016/j.marpetgeo.2015.07.004
Chen, Z., and Jiang, C. (2016). A revised method for organic porosity estimation in shale reservoirs using Rock-Eval data: Example from Duvernay Formation in the Western Canada Sedimentary Basin. AAPG Bull. 100 (3), 405–422. doi:10.1306/08261514173
Cooney, P., and Lorente, M. (1997). Implicaciones tectónicas de un evento estructural en el Cretácico Superior (Santoniense-Campaniense) de Venezuela Occiental. Memorias del VIII Congreso Geológico Venezonalo. Soc. Venez. Geólogos 1, 195–204.
Cortes, M., Cortes, Y., Higuera, C., García, D., and Maya, L. (2022). “Chapter 16 – regional structure, trap mechanisms, and basin evolution of the Middle Magdalena Basin of Colombia,” in Andean structural styles: A seismic atlas. Editors G. Zamora, and A. Mora (Amsterdam, Netherlands: Elsevier), 221–226.
Erlich, R. N., Villamil, T., and Keens-Dumas, J. (2003). “Controls on the deposition of Upper Cretaceous organic carbon–rich rocks from Costa Rica to Suriname,” in The circum-gulf of Mexico and the caribbean: Hydrocarbon habitats, basin formation, and plate tectonics: AAPG memoir 79. Editors C. Bartolini, R. T. Buffler, and J. Blickwede, 1–45.
Etayo-Serna, F. (2019a). “Basin development and tectonic history of the middle Magdalena Valley,” in Estudios geológicos y paleontológicos sobre el Cretácico en la región del embalse del río Sogamoso, Valle Medio del Magdalena, Compilación de los Estudios Geológicos Oficiales en Colombia. Editor F. Etayo-Serna (Bogota, Columbia; Bogotá: Servicio Geológico Colombiano), 413–423.
Etayo-Serna, F. (2019b). “Formación” simití: Unidad ambigua en la estratigrafía del valle medio del Magdalena,” in Estudios geológicos y paleontológicos sobre el Cretácico en la región del embalse del río Sogamoso, Valle Medio del Magdalena, Compilación de los Estudios Geológicos Oficiales en Colombia. Editor F. Etayo-Serna (Bogota, Columbia; Bogotá: Servicio Geológico Colombiano), 293–302.
Fertl, W. H., and Rieke, H. H. (1980). Gamma ray spectral evaluation techniques identify fractured shale reservoirs and source-rock characteristics. J. Petroleum Technol. 32 (11), 2053–2062. doi:10.2118/8454-PA
Galford, J., Quirein, J., Westacott, D., and Witkowsky, J. (2013). “Quantifying organic porosity from logs,” in Presented at SPWLA 54th Annual Logging Symposium, New Orleans, Luisiana, June 2013, 16.
Galvis-Portilla, H., Higuera, I., Cespedes, S., Ballesteros, C., Forero, S., Marfisi, N., et al. (2014). “Regional sequence stratigraphy of the upper cretaceous La Luna Formation in the Magdalena Valley Basin, Colombia,” in Paper presented at the SPE/AAPG/SEG Unconventional Resources Technology Conference, Colorado, United States, August 2014, 10.
Gamero-Diaz, H., Miller, C., and Lewis, R. (2013). “sCore: A mineralogy based classification scheme for organic mudstones,” in SPE Annual Technical Conference and Exhibition, New Orleans, Louisiana, USA, September 2013.
Gaona-Narvaez, T., Maurrasse, F. J. M., and Etayo-Serna, F. (2013). “Geochemistry, palaeoenvironments and timing of aptian organic-rich beds of the Paja formation (curití, eastern cordillera, Colombia),” in Isotopic studies in cretaceous research. Editors A. Bojar, M. Melinte-Dobrinescu, and J. Smit (London, United Kingdom; Geological Society, London, Special Publications), 31–48.1.
García, D., Parra, P., and Sánchez, C. (2001). Modelos de Generación de petróleo en el Valle Medio del Río Magdalena. Internal Report Ecopetrol-ICP. Piedecuesta 166, 5. annexes.
García-Delgado, H., and Velandia, F. (2020). Tectonic geomorphology of the serranía de San Lucas (central cordillera): Regional implications for active tectonics and drainage rearrangement in the northern Andes. Geomorphology 349, 25. 106914. doi:10.1016/j.geomorph.2019.106914
García-Gonzalez, M., and Carvajal, H. (2006). La supresión de la reflectancia de la vitrinita en las formaciones La Luna y Hondita y sus implicaciones en la exploración de hidrocarburos de las cuencas del VSM y VMM, Colombia. IX Simposio Bolivariano de Exploración Petrolera en las Cuencas Subandinas. https://www.acggp.org/ix-simposio-bolivariano-2006/.7Retrieved from:
Gomez, E., Jordan, T. E., Allmendinger, R. W., Hegarty, K., Kelley, S., and Heizler, M. (2003). Controls on architecture of the late cretaceous to cenozoic southern middle Magdalena Valley Basin. Colombia Geol. Soc. Am. Bull. 115 (2), 131–147. doi:10.1130/0016-7606(2003)115<0131:COAOTL>2.0.CO;2
Gomez, E., Jordan, T. E., Allmendinger, R. W., Hegarty, K., and Kelley, S. (2005). Syntectonic cenozoic sedimentation in the northern middle Magdalena Valley Basin of Colombia and implications for exhumation of the northern Andes. Geol. Soc. Am. Bull. 117 (5/6), 547–569. doi:10.1130/B25454.1
Gong, C., and Rodriguez, L. (2017). “Challenges in pore pressure prediction for unconventional petroleum systems,” in Presented at AAPG Hedberg Conference, The Future of Basin and Petroleum Systems Modeling, California, United States, April 2016.
Gonzalez, R., Suarez, C., Higuera, I., and Rojas, L. (2020). Alternative workflow for three-dimensional basin modeling in areas of structural complexity: Case study from the Middle Magdalena Valley, Colombia. AAPG Bull. 104, 1–19. doi:10.1306/0415191612917185
Grauls, D. (1999). Overpressures: Causal mechanisms, conventional and hydromechanical approaches. Oil Gas Sci. Technol. – Rev. IFP 54 (6), 667–678. doi:10.2516/ogst:1999056
Guerrero, J., Montes, L., Jaillard, E., and Kammer, A. (2021). Seismic interpretation of the cretaceous unconformities and sequences in the middle Magdalena Valley and the Western margin of the eastern cordillera, Colombia. Comptes Rendus Géoscience 353 (1), 155–172. doi:10.5802/crgeos.47
Hantschel, T., and Kauerauf, A. (2009). Fundamentals of basin and petroleum systems modelling. Berlin, Germany: Springer-Verlag, 476.
Holgate, F., and Gerner, E. (2010). OZTemp well temperature data. Retrieved from: https://researchdata.edu.au/oztemp-well-temperature-data/1215040
Horton, B. K., Anderson, V. J., Caballero, V., Saylor, J. E., Nie, J., Parra, M., et al. (2015). Application of detrital zircon U–Pb geochronology to surface and subsurface correlations of provenance, paleodrainage, and tectonics of the Middle Magdalena Valley Basin of Colombia. Geosphere 11 (6), 1790–1811. doi:10.1130/GES01251.1
Ideam, (2022). Open weather data. http://dhime.ideam.gov.co/atencionciudadano/.Retrieved from:
Jarvie, D. (2014). Components and processes affecting producibility and commerciality of shale resource systems. Geol. Acta 12 (4), 307–325. ALAGO Special Publication. doi:10.1344/GeologicaActa2014.12.4.3
Jimenez, M., Rojas, C., and y Valderrama, Y. (2015). “Initial analysis of hydrocarbon potential in the Tablazo formation; center and northern areas of middle Magdalena Valley, -mmv- Colombia,” in Paper presented at the SPE/AAPG/SEG Unconventional Resources Technology Conference, Texas, Unite States, July 2015.
Juliao, T., Suarez-Ruiz, I., Marquez, R., and Ruiz, B. (2015). The role of solid bitumen in the development of porosity in shale oil reservoir rocks of the Upper Cretaceous in Colombia. Int. J. Coal Geol. 147-148 (1), 126–144. doi:10.1016/j.coal.2015.07.001
Mews, K., Alhubail, M., and Barati, R. (2019). A review of brittleness index correlations for unconventional tight and ultra-tight reservoirs. Geosciences 9 (319), 319. doi:10.3390/geosciences9070319
Mora, C., Cerón, M., Quintero, M., Sanz, C., Rey, C., and Mejia, O. (2018). Evaluación de recursos en Yacimientos de Roca Generadora (YRG) Formación La Luna, Cuenca Valle Medio del Magdalena, Colombia. Bogotá, Colombia: Memorias I Cumbre del Petróleo y Gas.
Mora, C., Cordoba, F., Luna, O., Sarmiento, L. F., Rangel, A., Giraldo, N., et al. (1996). “Petroleum systems of the middle Magdalena Valley, Colombia,” in AAPG International Conference and Exhibition, Caracas, Venezuela, Bogotá, Colombia, August 1996, 1–7. Memorias de la Cumbre del Petróleo y Gas.
Morales, L. (1958). “The Colombian petroleum industry,” in General geology and oil occurrences of middle Magdalena Valley, Colombia: South America. Editor L. En: Weeks (Tulsa, Oklahoma: American Association of Petroleum Geologists Special Publication). Habitat of oil.
Parra, M., Mora, A., López, C., Rojas, L. E., and Horton, B. K. (2012). Detecting earliest shortening and deformation advance in thrust belt hinterlands: Example from the Colombian Andes. Geology 40 (2), 175–178. doi:10.1130/G32519.1
Passey, Q., Creaney, S., Kulla, J. B., Moretti, F. J., and y Stroud, J. D. (1990). A practical model for organic richness from porosity and resistivity logs. AAPG Bull. 74 (12), 1777–1794. doi:10.1306/0C9B25C9-1710-11D7-8645000102C1865D
Pepper, A. S., and Corvi, P. J. (1995). Simple kinetic models of petroleum formation. Part I: Oil and gas generation from kerogen. Mar. Petroleum Geol. 12 (3), 291–319. doi:10.1016/0264-8172(95)98381-E
Perez, E., Pachon, Z., Gomez, J., and Marin, P. (2014). “Integrated methodology for laboratory evaluation of Shale Plays cores,” in Paper presented at the SPE Latin America and Caribbean Petroleum Engineering Conference, Maracaibo, Venezuela, May 2014.
Piedrahita, J., and Aguilera, R. (2017b). “Models for calculating organic and inorganic porosities in shale oil reservoirs,” in Paper presented at the SPE Latin America and Caribbean Petroleum Engineering Conference, Buenos Aires, Argentina, May 2017.
Piedrahita, J., and Aguilera, R. (2017a). Moveable oil detection in a shale reservoir by integrating simultaneously geochemical, petrophysical and geomechanical models developed from well logs and laboratory test. Bogotá, Colombia. Paper presented at the Congreso Colombiano del Petróleo.
Ramirez, J., and Aguilera, R. (2014). “Factors controlling fluid migration and distribution in the eagle ford shale,” in Paper presented at the SPE/CSUR Unconventional Resources Conference – Canada, Alberta, Canada, September 2014.
Ramón, J. C., Dzou, L., and Giraldo, B. (1997). Geochemical evaluation of the middle Magdalena basin, Colombia. CT&F-Ciencia, Tecnol. Futuro 1 (3), 47–66.
Rangel, A., Osorno, J., Ramirez, J., De Bedout, J., Gonzalez, J., and Pabón, J. (2017). Geochemical assessment of the Colombian oils based on bulk petroleum properties and biomarker parameters. Mar. Petroleum Geol. 86, 1291–1309. doi:10.1016/j.marpetgeo.2017.07.010
Reyes, H., Montenegro, B., and Gómez, P. (2004). Tectonoestratigrafía y evolución geológica del Valle Inferior del Magdalena. Bol. De. Geol. 26 (42), 19–38.
Reyes, M., Kley, J., Mora, A., Bello-Palacios, D., Vargas, A., Carvajal-Torres, J., et al. (2022). “Chapter 15 – La Cira basement-high; middle Magdalena Valley Basin, Colombia,” in Andean structural styles: A seismic atlas. Editors G. Zamora, and A. Mora (Amsterdam, Netherlands: Elsevier), 207–219.
Rojas, D., Galeano, A., and Sanchez, C. (2013). First approach for quantifying undiscovered petroleum initially in place on unconventional resources for a new play in the Cretaceous La Luna Formation, at the north of the Middle Magdalena Valley Basin, Colombia. Bogotá, Colombia: XV Congreso Colombiano del Petróleo, 10. https://www.slideshare.net/marioprince1/first-approach-for-quantifying-undiscovered-petroleum-initially-in-place-on-unconventional-resources-for-a-new-play-in-the-cretaceous-la-luna-formation-at-the-north-of-the-middle-magdalena-valley-basin-colombia.Retrieved from:
Romero-Sarmiento, M. F., Ducros, M., Carpentier, B., Lorant, F., Cacas, M., Pegaz-Fiornet, S., et al. (2013). Quantitative evaluation of TOC, organic porosity and gas retention distribution in a gas shale play using petroleum system modeling: Application to the Mississippian Barnett Shale. Mar. Petroleum Geol. 45, 315–330. doi:10.1016/j.marpetgeo.2013.04.003
Sarmiento-Rojas, L. F. (2019). “Cretaceous stratigraphy and paleo-facies maps of northwestern South America,” in Geology and tectonics of northwestern South America. Frontiers in Earth sciences. Editors F. En Cediel, and R. P. Shaw (Berlin, Germany: Springer, Cham), 673–747.
Sarmiento-Rojas, L. F., Van Wess, J. D., and Cloetingh, S. (2001). Mesozoic transtensional basin history of the Eastern Cordillera, Colombian Andes: Inferences from tectonic models. J. S. Am. Earth Sci. 21, 383–411. doi:10.1016/j.jsames.2006.07.003
Schmoker, J., and Hester, T. (1983). Organic carbon in bakken formation, United States portion of williston basin. AAPG Bull. 67 (12), 2165–2174. doi:10.1306/AD460931-16F7-11D7-8645000102C1865D
Scotese, C. R., Gahagan, L. M., and Larson, R. L. (1988). Plate tectonic reconstructions of the cretaceous and cenozoic ocean basins. Tectonophysics 155 (1-4), 27–48. doi:10.1016/0040-1951(88)90259-4
Shurr, G., and Ridgley, J. (2002). “Unconventional shallow biogenic gas systems,” in Unconventional petroleum systems. Editors B. Law, and J. Curtis, 1939–1969. AAPG Bulletin.11.
Slatt, R., McCullough, B., Molinares, C., Baruch, E., and Turner, B. (2015). “Paleotopographic and depositional environment controls on “sweet spot” locations in unconventional resource shales: Woodford and Barnett shale examples: Part 2,” in Presented at AAPG Annual Convention & Exhibition, Colorado, United States, June 2015.
Suarez, M., Serrano, M., and Morales, M. (2000). Estilos Estructurales y Potencial de Entrampamiento de la Sección Cretácea, Cuenca del Valle Medio del Magdalena, Colombia. Presented A. T. VII Simp. Boliv. Exploración Pet. las Cuencas Subandinas. doi:10.3997/2214-4609-pdb.118.024esp
Suo, C., Peng, S., Chang, S., Duan, R., and Wang, G. (2012). A new calculating method of the curvature to predicting the reservoir fractures. Procedia Environ. Sci. 12, 576–582. doi:10.1016/j.proenv.2012.01.320
Terraza-Melo, R. (2019). “Formación La Luna”: Expresión espuria en la geología colombiana,” in Estudios geológicos y paleontológicos sobre el Cretácico en la región del embalse del río Sogamoso, Valle Medio del Magdalena, Compilación de los Estudios Geológicos Oficiales en Colombia. Editor F. Etayo-Serna (Bogota, Colombia: Bogotá: Servicio Geológico Colombiano), 303–362.
Thompson-Butler, W., Peters, K. E., Magoon, L. B., Scheirer, A. H., Moldowan, J. M., Blanco, V. O., et al. (2019). Identification of genetically distinct petroleum tribes in the Middle Magdalena Valley, Colombia. AAPG Bull. 103 (12), 3003–3034. doi:10.1306/04101918107
U.S. Energy Information Administration (Eia), (2015). Technically recoverable shale oil and shale gas resources: Northern south America. https://www.eia.gov/analysis/studies/worldshalegas/pdf/Northern_South_America_Columbia_Venezuela_2013.pdf.30Retrieved from
Vargas-Silva, D. A., Gambús-Ordaz, M., and Calderón-Carrillo, Z. (2019). Assessment of causes of overpressure different from sub-compaction: Application in unconventional reservoir. CT&F - Cienc. Tecnol. Y Futuro 9 (2), 5–14. doi:10.29047/01225383.177
Veiga, R., Micucci, E., Cuberes, M., and Bande, A. (2023). “Reservorios no convencionales en Latinoamérica: La Formación La Luna en el Valle Medio del Magdalena-Colombia,” in 11th Congreso de Exploración y Desarrollo de Hidrocarburos: Exploración y Sistemas Petroleros, Instituto Argentino del Petróleo y Gas, Argentina, 231–258. Available at: https://www.researchgate.net/publication/370057425_RESERVORIOS_NO_CONVENCIONALES_EN_LATINOAMERICA_LA_FORMACION_LA_LUNA_EN_EL_VALLE_MEDIO_DEL_MAGDALENA-COLOMBIA.
Villamil, T., Arango, C., and Hay, W. W. (1999). “Plate tectonic paleoceanographic hypothesis for Cretaceous source rocks and cherts of northern South America,” in Evolution of the cretaceous ocean-climate system: Boulder, Colorado. Editors E. Barrera, and C. C. Johnson, 191–202. Geological Society of America Special Paper.
Keywords: Middle Magdalena Valley Basin, unconventional reservoir, basin modeling, sweet spot area, Cretaceous
Citation: Pastor-Chacón A, Aguilera R, Triana JL, Paez-Reyes M, Cantisano M, Bravo L, Gamba N, Niño M, Delgado A, Mendoza G, Rodriguez JD, Romero-Ballén O, Ruiz MC, Buitrago H and Fuenzalida H (2023) Sweet spot areas for shale oil and shale gas plays in the Upper Cretaceous rocks of the Middle Magdalena Valley, Colombia: insights from basin modeling. Front. Earth Sci. 11:1146126. doi: 10.3389/feart.2023.1146126
Received: 17 January 2023; Accepted: 21 April 2023;
Published: 17 May 2023.
Edited by:
Salam Al-Rbeawi, Middle East Technical University, TürkiyeReviewed by:
Hamid Al-Sari, University of Misan, IraqCopyright © 2023 Pastor-Chacón, Aguilera, Triana, Paez-Reyes, Cantisano, Bravo, Gamba, Niño, Delgado, Mendoza, Rodriguez, Romero-Ballén, Ruiz, Buitrago and Fuenzalida. This is an open-access article distributed under the terms of the Creative Commons Attribution License (CC BY). The use, distribution or reproduction in other forums is permitted, provided the original author(s) and the copyright owner(s) are credited and that the original publication in this journal is cited, in accordance with accepted academic practice. No use, distribution or reproduction is permitted which does not comply with these terms.
*Correspondence: Andrés Pastor-Chacón, YWZwYXN0b3JAc2djLmdvdi5jbw==
Disclaimer: All claims expressed in this article are solely those of the authors and do not necessarily represent those of their affiliated organizations, or those of the publisher, the editors and the reviewers. Any product that may be evaluated in this article or claim that may be made by its manufacturer is not guaranteed or endorsed by the publisher.
Research integrity at Frontiers
Learn more about the work of our research integrity team to safeguard the quality of each article we publish.