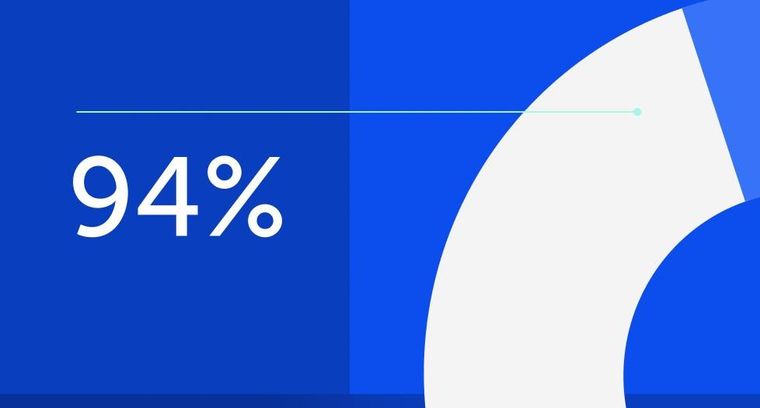
94% of researchers rate our articles as excellent or good
Learn more about the work of our research integrity team to safeguard the quality of each article we publish.
Find out more
ORIGINAL RESEARCH article
Front. Earth Sci., 05 May 2023
Sec. Cryospheric Sciences
Volume 11 - 2023 | https://doi.org/10.3389/feart.2023.1134728
In Greenland, snow avalanches have been recognized as a significant hazard and a risk to communities for decades. However, prior to this study, no formal avalanche database nor avalanche forecasting service existed in Greenland. This study was conducted over the 2021–2022 snow season to advance snow science research in Greenland and provide the town of Sisimiut with an avalanche bulletin service based on snowpack stability, weather conditions, and avalanche activity monitoring data within the Sisimiut backcountry. Snowpack stratigraphies were assessed and stability tests were performed periodically using standardized methods, and the results were linked with daily weather monitoring and avalanche event characterization. The observed avalanche activity was dominated by slab avalanches, accounting for 96% of the registered events. Instabilities were mainly driven by specific temperature patterns, strong winds, and rain episodes. During cold and calm periods, slow kinetic growth of snow crystals in low-density layers was observed. Abrupt thermal increases were recurrent, resulting in softening of superficial slabs with consequent reactivation of buried weak layers, thus reducing snowpack stability. On the other hand, prolonged warmer temperatures lowered the thermal gradient within the snowpack, leading to rounding and bonding of weak layer grains, thus recovering the snowpack stability. As observed on three occasions, rain events caused the formation of persistent weak layers and should be considered a warning for future snowpack instability. As a result of this study, we retroactively linked local weather patterns with snowpack instability and provided the first periodical avalanche bulletin service for Sisimiut.
Snow avalanches are one of the major hazards in mountains and, during the average winter, they cause around 100 fatalities in Europe alone (EAWS-European Avalanche Warning System, 2022). Avalanches are also a recurring hazard in Greenland, and fatal accidents have been reported in the past (DB-Dagbladet, 1998; Sermitsiaq, 2021). To date, all knowledge regarding avalanches has been passed on between Sisimiut residents without any formal documentation. In Sisimiut (Qeqqata Kommunia, Greenland), the local snowmobiling club organizes annual avalanche rescue training, and outdoors shops have started selling safety equipment. Although rescue courses and equipment are fundamental components of avalanche safety, they are insufficient in reducing risk on their own. Following the indications of an avalanche forecast to avoid avalanche terrain in adverse conditions can effectively reduce risk and potential accidents. However, to date, there is no permanent avalanche service anywhere in Greenland. To address this issue, the Arctic Technical University of Denmark (DTU) developed the project SOLO (Sisimiuts Oplands Lavinefare Oversigt), which provided a basic avalanche service in Sisimiut during the 2021–2022 winter season.
This study was developed around the data gathered for the SOLO project, with the aim of developing a scientific understanding of snow and avalanche characteristics in the Sisimiut backcountry. Meanwhile, a first-of-its-kind avalanche bulletin service was provided for the Sisimiut snow season (from November 2021 to June 2022) using the SOLO data interpreted and synthesized in this study. To date, snow research in Greenland has not focused on avalanche risk and related mitigation strategies, stressing the need for the study presented here and for snow science research in the country. Until now, only one study regarding avalanches in Greenland was available. The study by Jakob Abermann (2019) focuses on a single extreme case of late-season, wet snow avalanches, but is limited in data for avalanche characterization over an entire winter season in Greenland. Although other inhabited Arctic areas, such as Svalbard (Norway), have developed snow climate characterization (Markus Eckerstorfer, 2011) and avalanche forecasting services (Alexander Prokop, 2018), this type of knowledge and service is lacking in Greenland.
Although several types of avalanches exist (SLF, 2022), the most dangerous, accounting for more than 85% of fatalities worldwide according to Jurg Schweizer (2001), are slab avalanches. These avalanches assume the presence of a cohesive slab (soft or rigid, depending on its composition) that lies on a weak layer, which is usually composed of faceted grains or depth hoar. The release mechanism of a slab avalanche, which is still under study, is a sequence of fracture processing, including the failure initiation in the weak layer, the onset of crack propagation up to a critical crack length, the dynamic crack propagation through the weak layer, and, finally, the formation of a tensile fracture followed by the slab release (Jürg Schweizer B. R., 2016). Avalanche terrain is defined as any snowy slope that meets certain conditions, such that an avalanche can occur, like a slope angle between 28 and 60° ( Maggioni, 2003).
The study presented here is based on data collected through field monitoring during the 2021–2022 winter season in the backcountry of Sisimiut. Weather, snowpack stability, and avalanche activity were monitored simultaneously, on a daily to weekly basis. The collected data were documented in a project database and cross-analyzed to reconstruct the snowpack evolution and determine the main avalanche problems of the area (Benjamin Reuterab, 2022). The first snowpack observation was taken near the city at the beginning of the snow season on 11 November 2021. In situ observations were interrupted in mid-December due to the lack of daylight and weather, which yielded unsafe fieldwork conditions. The snowpack and avalanche monitoring restarted at the end of January, when the snowmobile and ski season started, and continued until 29 May 2022, when the snow conditions no longer allowed for snowmobile access into the backcountry. Weather monitoring continued throughout the entire season without interruption.
The area of interest is located within the backcountry of Sisimiut, the second-largest town in Greenland, with 5,582 registered inhabitants in 2020 (Statistics Greenland, 2022). The study area is between 66° 55′ N to 67° 00′ N, and 53° 18′ W to 53° 40′ W, approximately 50 km north of the Arctic Circle, bordered to the north by the Kangerluarsuk Tulleq Fjord and to the south by the Amerloq Fjord (Figure 1). The mountainous terrain is characterized by U-shaped valleys running on a W-E axis, used by the Sisimiut residents as access routes leading to the backcountry and, eventually, to the town of Kangerlussuaq, approximately 150 km inland. The main snowmobile track between Sisimiut and Kangerlussuaq is a regular commuting route for snowmobiles, dogsleds, skiers, and hikers and is highly trafficked during the winter season for recreational and professional purposes. The highest peak close to town is the Nasaasaaq mountain, standing at 784 m.a.s.l., with most of the remaining landscape ranging between 300 and 700 m.a.s.l. The ground surface is mostly covered by boulders, bushes, and other glacial debris, without trees. Mountain permafrost is widespread (Marcer et al., 2022), and no glaciers remain in the study area. A small ski area (Solbakken) is located 8 km east of the town on a south-facing hill.
Climatically, Sisimiut is located in the low Arctic Ocean area (Jensen H., 1999), with a mean annual temperature of −1.8°C for the period 2000–2020 and mean annual precipitation of 382 mm at sea level for the period 1961–2010 (Edward Hanna, 2020). The warmest month is July, averaging 6.3°C, while the coldest is March, averaging −14.0°C. The climate transitions from maritime to continental from the coast inland toward Kangerlussuaq, which results in a negative temperature gradient in the winter and a positive gradient in the summer (Edward Hanna, 2020).
Our observations focused on two subareas—SIS01 and SIS02—as they were most accessible and suggested by local experts as representative of the Sisimiut backcountry. SIS01 represents the north side of the Nasaasaaq range, including the cirque below the north face of the Nasaasaaq peak and the steep north-facing slopes furrowed by a series of couloirs (slopes up to 50°) that branch out from the summit ridge. These couloirs are frequently used by skiers and snowmobilers, and their debris fans reach the main valley near the main snowmobile track. SIS02 represents the main valley close to the Kangerluarsuk Tulleq Fjord. Here, the main track reaches a plateau at approximately 400 m.a.s.l., surrounded by rounded mountains with various exposed slopes that culminate on Carlsberg Mountain (730 m.a.s.l.). In addition to these areas, a sample area—SIS03—was chosen near the town, consisting of low-elevation rounded hills (maximum 52 m.a.s.l.) with slopes of all aspects. This area is not significant for avalanche activity but was easily accessible in any weather conditions (even when it was not possible to reach the backcountry), allowing for a direct correspondence between the meteorological observations from the town and the effects on the snowpack.
The weather monitoring data collection was mainly derived from daily observations at the Arctic DTU building (in the city center) and from the automatic Danish Meteorological Institute (DMI) weather station (Danish Meteorological Institute, 2022) located near the airport. When possible, manual weather measurements were taken from the backcountry and were coupled with the DMI data to have a better understanding of the actual weather conditions in the study area. The main data manually acquired consisted of new snow accumulation (HN), rainfall, and significant events such as blizzards and strong wind events. HN was measured in an undisturbed spot with a ruler, while air temperature, wind speed, wind direction (average and gust), and rainfall data were recorded by the DMI weather station. All collected weather data were recorded in the project database with a daily resolution.
Fifty-one snow pits were dug over the course of the winter season within the SIS01–03 areas to evaluate snow profiles, and weekly snow surface observations were simultaneously conducted. No documented snow profiles were available in the area prior to this study. The frequency of the surveying was increased, when possible, to analyze the evolution of particularly significant snowpack conditions. Snow profiling was conducted according to the International Classification for Seasonal Snow on the Ground (ICSSG) (C. Fierz and Armstrong, 2009). The standard snowpack parameters acquired include total snowpack depth (HS), layering, grain size, grain shape, hardness, snow temperature, snow surface temperature (Tss), and air temperature measured 40 cm above the snowpack (Tair). Field data were analyzed and plotted using NiViz1 software. The snowpack observations were always coupled with an extended column test (ECT) as a fast, reliable, and internationally recognized test for assessing snowpack stability. Column tests (CT) and Rutschblock tests (RT) were not consistently performed for practical reasons as the CT is less effective in detecting shear fracture characteristics (Kurt Winkler, 2009), and the RT requires ski mountaineering equipment, which was not always available to the field team during data collection. On occasion, the shovel shear test (SST) and the CT were carried out to confirm ECT results, while the propagation saw test (PST) was used to better understand the failure behavior of weak layers in particular conditions.
Avalanche event data were collected at the avalanche site whenever an event was directly observed or reported to the SOLO team by the Sisimiut residents. Terrestrial and UAV photographs were acquired, when possible, along with documentation of avalanche characteristics and dimensions. If an avalanche was directly observed or human-triggered, useful information was collected through interviews with the involved parties. Standard profiles and ECTs were also performed on the undisturbed snowpack near the avalanche crown if conditions were safe. The collected data were entered into the project database, and standardized reports, similar to those of the Colorado Avalanche Information Center (2022), were written for the main avalanche events or cycles. Based on all observations and data acquired, the daily avalanche activity index (AAI) was computed for the season. This is the weighted sum of all the observed avalanches, as proposed by Jurg Schweizer (2020). Local experts also provided valuable insight into common avalanche release areas and characteristics that increased the spatial resolution of the monitoring network and improved the avalanche database by capturing more remote avalanches across the Sisimiut backcountry study area.
During the monitored season, there were 96 days with precipitation (47% of the total monitored days). The main wind direction was east, which is reported as typical for the area (Thomas Ingeman-Nielsen, 2007). From mid-February to early April (approx.), the sea was continuously frozen close to the coast, while the fjords remained frozen until June. The minimum temperature registered by the weather station was −28.1°C, while in the backcountry, even lower temperatures were registered during the profiling campaigns. During the season, very cold periods were followed by rapid temperature increases, as seen in Figure 2. The precipitation was mainly in solid form (i.e., snow particles) during the season (84% of the episodes), 9% was rainfall, and the remaining 7% was mixed precipitation. Snowfall events were often followed by wind (24% of snowfall events) or occurred as a blizzard (i.e., strong wind during the precipitation, 26% of the snowfall events). Field observations confirmed this behavior, except for the SIS01 area, which is protected from the most frequent easterly wind direction. By monitoring the weather over the season, it was possible to group all observed weather conditions into six main recurrent meteorological events (ME). Each of these led to different effects on snowpack stability and avalanche activity.
ME 1: Blizzard (i.e., severe weather conditions with either falling or blowing snow, cloudy sky, and visibility less than 0.4 km), observed 18 times during the season.
ME 2: Snowfall without wind, observed 25 times during the season.
ME 3: Cold and calm period (i.e., Tmax<10°C and no wind for at least 5 consecutive days), observed twice during the season.
ME 4: Abrupt thermal increase (i.e., a sudden temperature increase over a few days to an above-average temperature), observed four times during the season.
ME 5: Rainfall episodes, observed six times during the season.
ME 6: Strong wind (i.e., moderate or strong wind episodes with good visibility), observed 48 times during the season.
FIGURE 2. Snow precipitation (HN), rain, average wind velocity, air temperature, and AAI patterns during the 2021–2022 winter season. “Dry and blown snow” is precipitation of dry snow during a wind event. The letters in the boxes represent the defined avalanche cases A-E.
During the season, 53 avalanches were observed and classified in the project database according to the EAWS standard (EAWS - European Avalanche Warning Service, 2021). As presented in Figure 3, 96% of the observed events were slab avalanches. Among them, 42 (79%) involved dry snow slabs (hard or soft), and 9 (17%) involved wet snow slabs. One gliding avalanche and one loose snow avalanche (of wet snow) were observed; however, both occurred in relatively inaccessible and low-risk areas. Most (82%) of the events were naturally triggered, while of those that were human-triggered, four were caused by hikers, three by snowmobilers, and only one by a skier. The dominant avalanche size was 1 (Small avalanche, 69%), and the largest observed avalanche was size 3 (Large avalanche). The 53 observed avalanches can be grouped into five different avalanche events or cycles (i.e., several avalanches occurred in the same period with similar characteristics), here named “Cases”. These are also distinguishable by looking at the peaks of the daily AAI pattern represented in Figure 2.
FIGURE 3. Avalanches observed during the 2021–22 season by type (A,C), by triggering cause (B), and by size (D).
Case A. The first avalanche event was observed on 5 February in a remote area, north of the Kangerluarsuk Tulleq Fjord. This large avalanche was triggered by a snowmobiler who was entirely buried for approximately 20 min and rescued by fellow riders without injury. The single event was the release of a wind slab of variable thickness (up to 1.5 m in the middle), which slid on a hard ice layer. This ice layer was detected in several profiles conducted at the beginning of February on similar slopes in both SIS01 and SIS02, with a weak layer of faceted crystals and depth hoar above. The avalanche occurred after a rain event on 19 January, followed by 2 weeks featuring several consecutive blizzards.
Case B. On 5 March, a hiker triggered a small-sized avalanche, which led to the release of a hard slab due to the collapse of a weak faceted layer. The avalanche occurred after a period with very cold temperatures (between −18°C and −28°C) and no perturbations, followed by a sharp thermal increase with strong winds (peak of above 4.5°C near the coast on the day of the avalanche).
Case C. On 16 March, a medium-sized slab avalanche near the town was triggered by a snowmobiler who induced the collapse of a faceted layer that released a recently formed soft slab. The avalanche occurred after light snowfalls with variable wind velocities and a temperature of approximately −20°C, deposited after a cold and anticyclonic period. Similar small natural avalanches and sluffs were observed in the vicinity.
Case D. From 25 to 27 March, an avalanche cycle with several small- and medium-sized, naturally triggered avalanches was observed. The avalanches occurred after a period of calm weather and very cold temperatures, followed by an abrupt thermal increase (around 0°C near the coast).
Case E. The last avalanche cycle occurred from 29 April through 1 May, with several human- and naturally triggered slab avalanches releasing after a significant wind event on 29 April. The strong wind redistributed fresh snow, forming a hard wind slab.Based on field observations, each case was assigned a standardized avalanche problem type, as defined by the EAWS (2021). Cases A, B, and D were classified as “persistent weak layer” problems, while Case C was classified as a “new snow” problem, and Case E as a “wind slabs” problem.
Widespread continuous snow cover formed with a series of light snowfalls from 11 to 19 November, losing continuity only at the end of May, limiting the monitoring and ending the snow season. Melting–refreezing cycles were occasionally observed from late March on sun-exposed slopes, particularly dictated by the multiday thermal trend rather than the night–day thermal variation (Figure 4). Interesting differences were noted between the general snowpack structure of SIS01 and SIS02. At SIS01, the snow was softer and more homogenous, while at SIS02, wind action was constant throughout the season, resulting in harder overlapping slabs and significant differences in thickness between upwind (east-facing) and downwind (west-facing) slopes. These differences were recognized through the measured snow surface harnesses at SIS01 and SIS02 (Figure 4). Because 96% of the observed events were slab avalanches, the snowpack monitoring results focus on the two main snowpack elements that characterize this avalanche type: the weak layer and the cohesive slabs above (Jürg Schweizer B. R., 2016).
FIGURE 4. The top graph represents the snowpack surface hand hardness test during the most active part of the season. The bottom graph is a timeline representing periods with visible snow surface melting.
The main weak layer grain type was faceted crystals (44%), followed by mixed forms (38%), depth hoar (6%), precipitation particles often decomposed and fragmented (6%), rounded grains (3%), and wind-broken particles (3%). In the profiles, the average weak layer depth was 78 cm from the surface, and it was observed that these weak layers were deactivated once buried deeply into the snowpack, as reported by Jurg Schweizer (2001).
All observed weak layers originated in two distinct ways (Figure 5). The first was the formation of faceted crystals near a newly formed melt–freeze crust due to the refreezing of the thin layer of melted forms (originating from a rainfall event) that released latent heat to the surroundings. The second process of forming weak layers was the slow faceting of low-density layers composed of precipitation particles (e.g., formed from ME 2), usually buried within the snowpack by wind slabs. This faceting process was due to the medium/high thermal gradient, typically found in the first meter during cold and calm periods (e.g., ME 3). After weeks, the presence of mixed forms as the main grain type suggested that this faceting was slow and incomplete. This condition allowed for the process to be reversed. Once the temperatures increased again, grain rounding was observed, thereby improving the stability of the weak layer and overall snowpack. In 12% of the profiles, the weak layer inside the snowpack was formed of newly precipitated particles, often decomposed by light wind. This case is associated with the “wind-drifted snow” EAWS avalanche problem (EAWS - European Avalanche Warning Service, 2021), which was observed on a few occasions during the season. Observations showed that without a high thermal gradient, the particles rounded within a few days after the wind transport event, and the snowpack stability improved. Alternatively, if the thermal gradient after the event was high, faceting and weak layer formation were observed.
FIGURE 5. Timeline representing the active weak layer within the snowpack during the 2021–2022 season, with the formation phenomenon and evolutionary behavior, divided into the three areas—SIS01, SIS02, and SIS03. The color blocks represent the main grain type forming the weak layers, while the aforementioned letters and numbers indicate the main ECT results obtained on the corresponding weak layer. The letters A–E above the timeline identify the main avalanche events (Cases A-E) that are linked to the collapse of the indicated weak layer. The y-axis shows the relative depth of the snowpack, with more recent weak layers on top and older layers buried below.
Only 6% of the observed slab avalanches involved soft cohesive slabs formed from the new precipitation without wind action. All other events consisted of a release of hard slabs with high density composed of small, rounded particles (average dimension around 0.3 mm). Hard slabs were the most common layer observed in the snowpack during the season. After periods of consecutive blizzards (i.e., ME 1), the formation of overlapping hard slabs was observed, initially distinguishable by the variable hardness due to the different wind velocities during precipitation. In the absence of layers with different grain types at the interface, even if the slabs were separable with the SST in the hours immediately after the deposition, the shear surfaces between the layers were no longer straight after 24 h. The ECT never induced collapse in this snowpack structure. A snowpack more predisposed to slab avalanche release was observed when hard slabs formed from single wind transport events because they often settled over soft layers. Observations showed that slabs were harder and more resistant with very cold air and snow surface temperatures (e.g., ME 3), resulting in better snowpack stability properties highlighted by the ECT, even with the presence of weak layers below. Sometimes, in these conditions, digging the snow pits proved to be difficult due to the hardness of the slabs. Conversely, slab softening was observed during thermal increase to above-average temperatures (i.e., ME 4), with consequent reactivation of the underlying weak layers, highlighted by both ECTs and avalanche activity.
Observations during the monitored season identified a snowpack of variable thickness (up to 5 m thick where wind-drifted snow accumulated) with a thick depth hoar layer (up to 50 cm) at the base, as typical of the Arctic Snowpack (Florent Domine, 2018). The widespread presence of depth hoar never showed instability, nor were avalanches observed on this layer. This was likely due to the general roughness of the terrain, mainly occupied by boulders and shrubs. Further snowpack monitoring, as well as an increase in the available avalanche observations, would be necessary to better characterize this depth hoar behavior.
The snow density was lower at the bottom of the snowpack, while the middle of the snowpack was generally of high density, formed by overlapping wind slabs. On some occasions, discontinuities were found in this part of the snowpack, formed by weak layers of faceted crystals or melt-freeze crusts with high hardness. The weaknesses formed in two distinct ways and were particularly unstable during sudden thermal increases leading to recurrent avalanche events or cycles. The significant snowpack differences between SIS01 (thicker and softer) and SIS02 (shallower and harder) were related to the geomorphology of the two areas as SIS01 is protected from the predominant wind direction (east). The “Tundra” snow class proposed by Sturm (1995) does not fully describe the snow structure observed in the study area. However, Markus Eckerstorfer (2011) introduced a new “High Arctic Maritime Snow Climate” class for Svalbard, which is similar to that of the Sisimiut backcountry. Compared to Svalbard, however, the Sisimiut snowpack was significantly thicker, with an average snow profile depth of approximately 200 cm, as opposed to a depth of 130 cm in Svalbard.
As 96% of the observed events were slab avalanches, it is implied that this type of avalanche is the main hazard in the area. This was confirmed by discussing the issue with the local population, which suggests that our observation period was, in fact, representative of the average seasonal conditions in the area. The recurrent weather events registered throughout the season (primarily strong wind and rapid thermal change with occasional rainfall) acted together, predisposing the snowpack to slab avalanche formation and temporarily increasing the AAI.
Consecutive blizzards (ME 1) led to the formation of overlapping slabs that, in the absence of different grain types at the interface, bonded together within a few days. The alternation of snowfall without wind (ME 2) followed by a wind event (ME 6) created new slabs on softer layers, increasing the instability in the 24–48 h following the events (e.g., Case E), after which time, the instability was usually reduced. However, with very cold temperatures able to increase the thermal gradient, faceting of the softer layer occurred, which prolonged instability over time. Grain faceting is produced by vapor diffusion within the snowpack in medium or high thermal gradient conditions. When the process is intense, the formation of depth hoar occurs, which is irreversible unless melting occurs. These grain types have poor cohesion and shear strength, constituting weak layers that often persist over time (McClung and Schaerer, 1993). New snowfalls without wind (ME 2) usually resulted in the accumulation of moderate amounts of fresh snow with very low density and high felting cohesion, which usually prevented the formation of dry, loose snow avalanches, if not on isolated steep slopes (i.e., sluffs). If the snowfall occurred after a cold and calm period (ME 3), the snow often settled on a layer subjected to near-surface metamorphism processes (S.C. Colbeck, 1989). This faceted surface behaved as a new weak layer predisposing the snowpack to small/medium soft slab avalanches, as observed on 16 March (Case C).
Cold air temperatures were responsible for the formation of the first weak layer by increasing the thermal gradient in the top meter of the snowpack (S.C.Colbeck, 1982). The very cold temperatures led to a slower and incomplete faceting of the softer layers buried within the snowpack, creating a weakness that was reversible once temperatures increased again, as reported by McClung and Schaerer, 1993.
Abrupt thermal increases after very cold periods occurred several times in the season. It was observed that these air temperature oscillations were a major factor leading to recurrent avalanche events or cycles. This behavior was well characterized through several snowpack profile assessments and ECTs conducted on the same slope from 18 February to 21 March, during which time, an avalanche cycle occurred (Case B on 5 March) after a thermal increase from −20°C to higher than 0°C. Each ECT failure was triggered by the collapse of the same weak layer as the March 5th avalanche. The monitoring results captured the physical evolution of the weak layer along with the development of the overlapping slabs, as summarized in Figure 6. In the studied period, the initial low temperatures led to a high thermal gradient within the first meter from the snowpack surface, which resulted in the faceting of a thin buried soft layer and the degradation of its mechanical properties from a stability perspective (McClung and Schaerer, 1993). Nevertheless, in this condition, the overlapping slabs were very rigid and stiff. Once the temperature increased (approximately +20°C from 25 February to 3 March), the superficial slabs softened, and the stability was reduced, as proven by the ECTs, resulting in the Case B avalanche triggered by a relatively low overload on the warmest day (Benjamin Reuter, 2012). During the warmer days, the thermal gradient within the first meter was close to zero, and grain rounding within the weak layer was observed in the days after the event. This improved the snowpack stability up to the complete disappearance of the weak layer below the slab, which became rigid again when temperatures dropped.
FIGURE 6. The bottom graph shows air temperature (Tair), snow surface temperature (Tss), and ECT results on a monitored weak layer during a sudden warming period. The upper graph reports the maximum thermal gradient, measured every 10 cm around the weak layer. Case B is indicated on the graph as “B”.
The monitoring confirmed what was demonstrated by Reuter (2012): cold and calm periods (ME 3) can have a double effect on the snowpack. On the one hand, the conditions facilitate an adverse evolution of the weak layers as a result of faceting from a high thermal gradient. On the other hand, the conditions increase slab stiffness and bearing capacity. A double effect was also found for abrupt thermal changes (ME 4), which led to slab softening with consequent weak layer reactivation, while at the same time reducing the thermal gradient and favoring rounding and bonding of grains within the weak layers. Recovery of the snowpack stability occurred when temperatures dropped again as the weakness within the snowpack was removed and the slabs returned to a rigid and stiff state. This snowpack evolution process led to at least two of the five AAI peaks (Case B and Case D in Figure 2). Identification of this pattern made it possible to forecast periods of increased avalanche danger linked with rising air temperatures.
The second weak layer formation process started from rain events (ME 5), which led to snow surface melting and subsequent refreezing once temperatures decreased. The refreezing process created a thin faceted layer near the newly formed melt–freeze crust because of latent heat release, which locally increased the thermal gradient, leading to kinetic growth typically within 24 h. The newly formed weak layers usually persisted for long periods due to the rapid and often complete faceting. These weak layers must also be considered particularly dangerous because they overlap a layer with a significantly different grain type, grain size, and hardness. This creates a snowpack structure particularly prone to instability, according to Jurg Schweizer (2007). On 19 January, a rapid thermal increase with associated rain melted the snow surface, on which a few centimeters of dry snow settled once the temperatures decreased. This formed a thin, weak layer of faceted crystals and depth hoar that was later buried deeper by a series of blizzards. The largest avalanche of the season was triggered along this layer (Case A). This phenomenon is typical in maritime mountain ranges (Jamieson, 2006) and was observed in Sisimiut three times during the season after sudden temperature changes with associated rain. The faceted layer usually formed above the crust if a new dry snow layer settled on the unfrozen surface immediately after the rain. However, because melt–freeze crust layers are relatively impermeable to vapor transport, faceted snow sometimes grew immediately below the crust, even when the surrounding crystals were well-rounded, as shown by Jamieson (2006).
In addition to temporary melting episodes from sudden thermal increases, continuous melt–refreeze cycles were observed from mid-April on sun-exposed slopes, and a few cases of very small wet slab avalanches were registered. From early May to August, Sisimiut has sunlight for most of the day, illuminating all slope aspects over 24 h. As such, once the temperature increased over the frost level for a significant time, the melting–refreezing cycles ended, and the continuous snow cover began to disappear, starting from the coast and moving inland. A few medium/large gliding avalanches were noticed near the Amerloq Fjord on steep south-facing channels leading into the sea. Further investigations were not carried out in these areas, given their limited accessibility and low risk to the population.
From February to mid-May, in conjunction with the Sisimiut snowmobiling and skiing season, a weekly avalanche bulletin specifically created for the type of users was published in three languages—Greenlandic, Danish, and English—via Facebook. The bulletin was created following the standards defined by the EAWS (2021), and, in particular, according to the EAWS matrix (Karsten Müller, 2016). Information including the avalanche danger level, the main avalanche problem type(s), and the terrain with increased avalanche danger was provided based on all monitoring data for the week combined with the synthesized interpretations of snowpack and avalanche characterization for the Sisimiut backcountry from this study. The bulletin content was continuously improved during the season by evaluating the feedback from the local users.
Monitoring of weather conditions, snowpack stability, and avalanche activity in the Sisimiut backcountry allowed for a good characterization of the 2021–2022 winter season, which, according to the local experts, can be considered representative of average seasonal conditions for the area. This was confirmed by comparing the average monthly precipitation, temperature, and wind speed registered during the monitored season with 1991–2020 climatological standards for the area, available in Cappelen and Drost Jensen (2021). The Sisimiut snowpack was classified according to the international classifications proposed by Sturm (1995) as a hybrid between the “Tundra snow climate” and the “Maritime snow climate”. However, the new High Arctic Maritime Snow Climate class proposed by Markus Eckerstorfer (2011) seems most representative of the complex layering found near Sisimiut, with alternating melt–freeze crusts, faceted layers, and hard slabs. Over the course of the season, 53 avalanches were observed and documented. The dominant avalanche type in this area was slab avalanches (96%). Through detailed monitoring throughout the season, some recurrent weather events were recognized. These acted together on the snowpack, changing its structure and physical properties and temporarily increasing the avalanche activity and hazard level. As a result of this study, two typical processes forming weak layers were defined: 1) the formation of melt–freeze crusts and 2) faceting in very cold and calm periods. Rain events were recorded three times during the snow season and can be considered an important warning for future snowpack instability as they were observed to form strongly persistent weak layers. These layers were caused by a local thermal gradient increase from latent heat release during refreezing of the melted layer. Monitoring of the weak layers within the snowpack throughout the season allowed for their evolutionary characterization with respect to the observed weather events. Air temperature patterns can be considered the main climatic factor, as they were able to cyclically increase the AAI by softening the superficial slabs and reactivating the buried weak layers. These new findings will allow for increased avalanche forecasting capacity in the future, with a focus on the described weather events and their expected effects on snowpack stability. Additionally, as this study methodology was successfully tested, it is possible to implement this project in other locations in Greenland.
Regarding limitations, the discontinuity of monitoring at the end of December and throughout January (imposed by adverse weather conditions and lack of daylight) is not believed to have affected the results of the study. However, because only one meteorological station is present in the area, a lack of climatic weather data was found, and the monitoring network could be improved. The set-up of automatic stations is advised to improve data acquisition and create the basis for automatic modeling to support decision-making, for example, via SNOWPACK software (Perry Bartelt, 2002). Concerning the Sisimiut study area, another location frequently visited by snowmobilers is the area north of the Kangerluarsuk Tulleq fjord, characterized by the Aqqutikitsoq mountain (1.448 m.a.s.l.) and its surrounding glaciers. This area was excluded from both the avalanche bulletin and the overall study due to the difficulties of continuous access, but its future inclusion in an avalanche forecasting system for Sisimiut would be considered valuable.
The raw data supporting the conclusions of this article will be made available by the authors, without undue reservation.
AM: article writing, data processing, on-site monitoring management, results processing, and elaboration. AA: support in field monitoring and data processing. DB: support in field monitoring and data processing. MM: support in field monitoring and data processing, and project and resource management. TI-N: support in data elaboration and resource collection for the project. FM: support in data elaboration as a snow science expert. AC: support in data elaboration as a snow science expert.
This study is part of the project SOLO, funded by the starting grant from NIS - Greenland Research Council. Arctic DTU supported the study by providing equipment and infrastructure.
Author FM was employed by Alpsolut srl.
The remaining authors declare that the research was conducted in the absence of any commercial or financial relationships that could be construed as a potential conflict of interest.
All claims expressed in this article are solely those of the authors and do not necessarily represent those of their affiliated organizations, or those of the publisher, the editors, and the reviewers. Any product that may be evaluated in this article, or claim that may be made by its manufacturer, is not guaranteed or endorsed by the publisher.
Alexander Prokop, H. H. (2018). Slope scale avalanche forecasting in the arctic (Svalbard). Innsbruck: International Snow Science Workshop, 1035–1039.
Avalanche Information Center. (2022). Accidents. Retrieved from Avalanche. Available at: https://avalanche.state.co.us/
Benjamin Reuter, J. S., and Schweizer, J. (2012). The effect of surface warming on slab stiffness and the fracture behavior of snow. Cold regions Sci. Technol. 83-84, 30–36. doi:10.1016/j.coldregions.2012.06.001
Benjamin Reuterab, L. V., Viallon-Galinier, L., Horton, S., van Herwijnen, A., Mayer, S., Hagenmuller, P., et al. (2022). Characterizing snow instability with avalanche problem types derived from snow cover simulations. Cold regions Sci. Technol. 194, 103462. doi:10.1016/j.coldregions.2021.103462
Cappelen, J., and Drost Jensen, C. (2021). Climatological standards normals 1991-2020 - Greenland. Copenhagen: Danish Meteorological Institute.
Colbeck, S. C. (1982). An overview of seasonal snow metamorphism. Rev. Geophys. 20, 45–61. doi:10.1029/rg020i001p00045
Colbeck, S. C. (1989). Snow-crystal growth with varying surface temperatures and radiation penetration. J. Glaciol. 35, 23–29. doi:10.3189/002214389793701536
Danish Meteorological Institute. (2022 ). DMI, Available at: https://www.dmi.dk/vejrarkiv/. Retrieved from DMI
DB - Dagbladet (1998). Omkom under grønlands-langrenn. Available at: https://www.dagbladet.no/sport/omkom-under-gronlands-langrenn/65449029.
EAWS - European Avalanche Warning Service (2021). EAWS, standards. Available at: www.avalanches.org.
EAWS - European Avalanche Warning System (2022). Fatalities. Available at: www.avalanches.org.
Edward Hanna, J. C., Cappelen, J., Fettweis, X., Mernild, S. H., Mote, T. L., Mottram, R., et al. (2020). Greenland surface air temperature changes from 1981 to 2019 and implications for ice-sheet melt and mass-balance change. Int. J. Climatol. 41. doi:10.1002/joc.6771
Fierz, C., and Armstrong, R. L. (2009). The international classification for seasonal SNOW ON the ground. IHP-VII Tech. Documents Hydrology No. 83, IACS Contributions 1, 80.
Florent Domine, M. B.-B., Belke-Brea, M., Sarrazin, D., Arnaud, L., Barrere, M., and Poirier, M. (2018). Soil moisture, wind speed and depth hoar formation in the Arctic snowpack. J. Glaciol. 64, 990–1002. doi:10.1017/jog.2018.89
Jakob Abermann, M. E., Eckerstorfer, M., Malnes, E., and Hansen, B. U. (2019). A large wet snow avalanche cycle in West Greenland quantified using remote sensing and in situ observations. Nat. hazards, Blind 97, nr.2, 517–534. doi:10.1007/s11069-019-03655-8
Jamieson, B. (2006). Formation of refrozen snowpack layer and their role in slab avalanche release. Rev. Geophys. 44, RG2001. doi:10.1029/2005RG000176
Jensen, H., P. L., Pedersen, L., Burmeister, A., and Winding Hansen, B. (1999). Pelagic primary production during summer along 65 to 72°N off West Greenland. Polar Biol. 21, 269–278. doi:10.1007/s003000050362
Jürg Schweizer, B. R. (2016). AVALANCHE RELEASE 101. Proceedings, international snow science workshop. Breckenridge, Colorado: International Snow Science Workshop.
Jurg Schweizer, C. C., and Camponovo, C. (2001). The skier's zone of influence in triggering slab avalanches. Ann. Glaciol. 32, 314–320. doi:10.3189/172756401781819300
Jurg Schweizer, C. M. (2020). On the relation between avalanche occurrence and avalanche danger level. Zurich: University of Zurich.
Jürg Schweizer, J. B., and Jamieson, J. B. (2007). A threshold sum approach to stability evaluation of manual snow profiles. Cold Regions Sci. Technol. 47, 50–59. doi:10.1016/j.coldregions.2006.08.011
Kurt Winkler, J. S., and Schweizer, J. (2009). Comparison of snow stability tests: Extended column test, rutschblock test and compression test. Cold Regions Sci. Technol. 59, 217–226. doi:10.1016/j.coldregions.2009.05.003
Maggioni, M., and Gruber, U. (2003). The influence of topographic parameters on avalanche release dimension and frequency. Cold Reg. Sci. Technol. 37, 407–419. doi:10.1016/S0165-232X(03)00080-6
Marcer, M., Duvillard, P. -A., Tomaškovicová, S., Nielsen, S. R., Revil, A., and Ingeman-Nielsen, T. (2022). Characteristics and evolution of bedrock permafrost in the Sisimiut mountain area, West Greenland. The Cryosphere Discuss. [preprint]. doi:10.5194/tc-2022-189
Markus Eckerstorfer, H. H., and Christiansen, H. H. (2011). The “high arctic maritime snow climate” in central svalbard. Arct. Antarct. Alp. Res. 43, 11–21. doi:10.1657/1938-4246-43.1.11
Perry Bartelt, M. L., and Lehning, M. (2002). A physical SNOWPACK model for the Swiss avalanche warning: Part I: Numerical model. Cold Regions Sci. Technol. 35, 123–145. doi:10.1016/s0165-232x(02)00074-5
Sermitsiaq (2021). Forudser fatal lavineulykke i ar ˚. Available at: https://sermitsiaq.ag/forudser-fatal-lavineulykke-i-aar?fbclid=IwAR01o6XWcTesZ0TZPoY0a5oIB8vu4n-G1lNdItegcAztbmmJk_IyZjP40MI.
Statistics Greenland (2022). StatBank Greenland. Available at: https://bank.stat.gl/pxweb/en/Greenland/.
Sturm, M., Holmgren, J., and Liston, G. E. (1995). A seasonal snow cover classification system for local to global applications. J. Clim. 8, 1261–1283. doi:10.1175/1520-0442(1995)008<1261:assccs>2.0.co;2
Thomas Ingeman-Nielsen, J. F.-R. (2007). International Conference, Arctic Roads: Operating, maintaining and building roads in a climatically challenging environment Arctic roads - international conference: Operating, maintaining and building roads in a climatically challenging environment. Sisimiut, Greenland: DTU Byg, Danmarks Tekniske Universitet, 53–61.
Keywords: snow science, avalanche, Greenland, weak layer formation, arctic snowpack, avalanche monitoring, avalanche bulletin
Citation: Mariani A, Abrahamsen AB, Bridle D, Ingeman-Nielsen T, Cicoira A, Monti F and Marcer M (2023) Snowpack and avalanche characterization over the 2021–2022 winter season in Sisimiut, West Greenland. Front. Earth Sci. 11:1134728. doi: 10.3389/feart.2023.1134728
Received: 30 December 2022; Accepted: 10 April 2023;
Published: 05 May 2023.
Edited by:
Alun Hubbard, Aberystwyth University, United KingdomReviewed by:
Lasse Laurson, Tampere University, FinlandCopyright © 2023 Mariani, Abrahamsen, Bridle, Ingeman-Nielsen, Cicoira, Monti and Marcer. This is an open-access article distributed under the terms of the Creative Commons Attribution License (CC BY). The use, distribution or reproduction in other forums is permitted, provided the original author(s) and the copyright owner(s) are credited and that the original publication in this journal is cited, in accordance with accepted academic practice. No use, distribution or reproduction is permitted which does not comply with these terms.
*Correspondence: Alberto Mariani, YWxiZXJ0b21hcmlhbmk5N0BnbWFpbC5jb20=
Disclaimer: All claims expressed in this article are solely those of the authors and do not necessarily represent those of their affiliated organizations, or those of the publisher, the editors and the reviewers. Any product that may be evaluated in this article or claim that may be made by its manufacturer is not guaranteed or endorsed by the publisher.
Research integrity at Frontiers
Learn more about the work of our research integrity team to safeguard the quality of each article we publish.