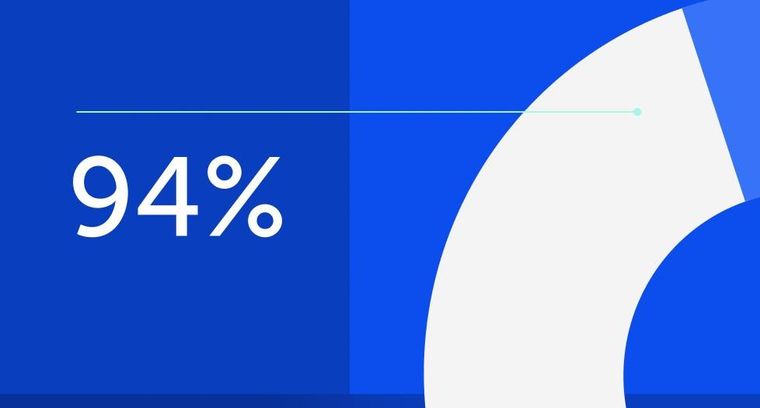
94% of researchers rate our articles as excellent or good
Learn more about the work of our research integrity team to safeguard the quality of each article we publish.
Find out more
ORIGINAL RESEARCH article
Front. Earth Sci., 07 February 2023
Sec. Structural Geology and Tectonics
Volume 11 - 2023 | https://doi.org/10.3389/feart.2023.1107833
This article is part of the Research Topic(Paleo-) Pacific Plate Subduction Tectonics and Related Magmatism and MineralizationView all 24 articles
Island arcs are postulated as the juvenile components that contribute to the growth of continental crust. Growth rates of arc crusts were previously computed using crustal thicknesses derived from seismic data. Consequently, crustal growth rates of oceanic island arcs are also constrained by the limited seismic data availability. This work presents the first comparison of gravity-derived magmatic growth rates of Western Pacific oceanic island arcs. We used the statistical correlation between Bouguer anomalies and seismic-derived crustal thicknesses to generate an empirical formula. The new empirical formula was utilized to estimate the crustal thicknesses of oceanic island arcs using Bouguer anomalies from the EGM2008 global gravity model. The resulting crustal thicknesses were employed to compute the magmatic growth rates of western Pacific island arcs and the Philippine island arc system. The latest magmatic growth rate estimates show that the magmatic productivity of Western Pacific island arcs, which are directly associated with Pacific Plate subduction, is significantly higher (28–60 km3/km/m.y). The growth rate of the Pacific island arcs is higher compared to the magmatic growth rate computed for the other oceanic island arcs (12–25 km3/km/m.y), which are derived from the subduction of other oceanic lithospheres (i.e., the Philippine Sea Plate; Caribbean Sea Plate; and Eurasia-South China Sea slabs). This is attributed to the variation in the ages of the subducting plates. The Pacific Plate, being older, is associated with higher degrees of serpentinization and sediment cover, which introduce more volatiles inducing more robust partial melting of the mantle wedge.
Materials constituting the continents are thought to have possibly originated from the juvenile products generated in active arcs (e.g., Reymer and Schubert, 1984; von Huene and Scholl, 1991; Condie, 1997; Kodaira et al., 2007; Turner and Langmuir, 2015). As such, island arcs can be considered one of the building blocks contributing to the growth of the Earth’s continental crust. Moreover, studies on collisions of island arcs with continental crust support the idea of continental growth through arc-continent collision. This has been documented in Southeast America (e.g., Byrne et al., 1985; Mann et al., 2002; DeMets et al., 2010; Granja Bruña et al., 2014), Timor (e.g., Karig et al., 1987; Nugroho et al., 2009; Harris, 2011), Taiwan (e.g., Huang et al., 1997; Lundberg et al., 1997; Malavieille et al., 2002; Byrne et al., 2011; Hsieh et al., 2020) and the Philippines (e.g., Yumul et al., 2009; Zamoras et al., 2008; Dimalanta et al., 2009; Concepcion et al., 2012; Manalo et al., 2016). Deciphering the processes that control the growth of an arc crust and its structure is, therefore, crucial in understanding crustal processes that actively influence magmatic crustal compositions (e.g., Plank and Langmuir, 1988; Farner and Lee, 2017).
The rate of productivity of arc magmatism is attributed to factors such as the nature of subduction (e.g., rate and angle of subduction) (e.g., Luo and Zhang, 2018); and the degree of hydration in the subduction system (e.g., Rupke et al., 2004; Zellmer et al., 2014). The subducted volatiles can induce flux melting of the mantle wedge and significantly influence the arc magmatic productivity in a trench-arc system. Several studies have shown that the amount of subducted volatiles are somehow related to the age of the subducting plate (e.g., Hay et al., 1998; Olson et al., 2016). These studies demonstrated that older subducting oceanic lithospheres are associated with thicker sediment covers and higher degrees of serpentinization. These conditions promote more volatiles to the subduction system causing a higher degree of flux melting (e.g., Luhr, 1992; Ulmer, 2001; Huang et al., 2006; Zellmer et al., 2014). In addition to subduction zone magmatism, arc crustal growth can also be induced via amagmatic contributions primarily from ophiolite emplacement (e.g., Dewey and Windley, 1981; Rait, 2000; Dimalanta and Yumul, 2003; Huang et al., 2015).
In the absence of seismic data, gravity data can be utilized as a reasonable substitute to estimate the thickness of island arcs. Several authors used available seismic data and Bouguer anomalies to generate an empirical formula between the two to compute crustal thickness (e.g., Worzel and Shurbet, 1955; Woollard, 1959; Milsom et al., 1996; Assumpcao et al., 2013). The studies done by Worzel and Shurbet (1955), Woollard (1959), and Assumpcao and others (2013) were all done in continental settings. Milsom and others (1996) also proposed an empirical equation derived using the average physical parameters of a continental crust, e.g., a 30-km thickness for a standard continental crust. Despite this, their equation has been used to estimate the thickness of the crust beneath oceanic island arcs. It is worth noting that crustal thickness studies in island arcs using Bouguer anomalies are not as extensive as crustal thickness estimates in continents. Reliable crustal thickness estimates for arcs are provided by ocean bottom seismometer (OBS) data such as that for the Ryukyu arc (Nishizawa et al., 2017), Izu-Bonin arc system (Suyehiro et al., 1996), and Aleutians arc (Holbrook et al., 1999).
One of the earliest crustal thickness models, the CRUST 5.1, was developed by Mooney et al. (1998). The 5° × 5° crustal thickness map of the world was produced using published seismic refraction data and information about the ice and sediment thickness. Subsequent maps and models with greater resolutions were produced by incorporating gravity data to address the need for more local-scale studies involving crustal thickness models. These studies resulted in the CRUST 2.0 model, which is a 2° × 2° crustal thickness map (Bassin et al., 2000), and the CRUST 1.0 model, which is a 1° × 1° crustal thickness map (Laske et al., 2013). While these provide reasonable estimates for the global distribution of crustal thickness, the resolution of these data is unsuitable for local studies such as characterizing the crustal thickness of specific island arcs.
In some island arcs where seismic data are unavailable, passive geophysical methods, such as the gravity method, are a reasonable substitute. The gravity method is significantly cheaper, and the spatial availability of data is also considerably better. The gravity method operates on the principle that the crust-mantle boundary is often marked by a significant change in physical properties, including density (e.g., Maystrenko and Scheck-Wenderoth, 2009; Carbonell et al., 2013). Thus, the depth to the Moho interface is treated as the crustal thickness (Bai et al., 2014). In theory, due to along-arc variations in oceanic island arcs, Bouguer anomalies, through gravity/density inversion, can also be used to produce subsurface models of the oceanic island arcs. For instance, Bouguer anomalies, albeit with additional constraints from seismic data, have been previously applied to produce subsurface models of the Bonin arc (e.g., Kodaira et al., 2011).
Various empirical formulae have been defined showing the relationship between Bouguer anomalies and the thickness of the crust derived from seismic surveys (e.g., Worzel and Shurbet, 1955; Woollard, 1959; Milsom et al., 1996; Assumpcao et al., 2013; Bai et al., 2014). Of these formulae, the one proposed by Milsom and others (1996) has been widely applied to oceanic arc settings (e.g., Dimalanta et al, 2002; Manalo et al., 2015; Parcutela et al., 2020; 2022). Based on experimental modeling, they proposed that a 1-km change in the thickness of a 30-km-thick standard continental crust will be accompanied by a 16-mGal change in the Bouguer anomaly.
This empirical formula is consistent with the observation by Huang and others (2006) regarding the relationship between Bouguer anomalies and depth to Moho in continental settings. While formulas such as these provide good estimates of crustal thickness, their applicability might be limited to the specific geologic setting which the datasets were derived from (Stolk et al., 2013). An empirical formula based on a continental setting might not work well for island arc settings, given the substantial differences between the overall composition of continental and island arc crust. Although the formula proposed by Milsom and others (1996) has been used to estimate the crustal thickness of island arcs (e.g., the Philippines), it should be noted that their formula was not constrained by ocean bottom seismometer (OBS)- or multi-channel seismic (MCS)-derived crustal thicknesses. Hence, there is currently no empirical formula for the relationship between seismic-derived crustal thickness and Bouguer anomalies in oceanic island arcs. This work will estimate the crustal thickness of several oceanic island arcs without seismic data. This, in turn, will be useful in calculating arc crustal growth rates using gravity data.
The new empirical formula is based on a linear regression analysis between the seismic-derived crustal thickness of oceanic island arcs with their corresponding Bouguer anomalies.
Available crustal thickness data of oceanic island arcs with published crustal thickness sections derived from OBS and MCS surveys were utilized in this study. Transects signifying pure oceanic island arc signatures and only crustal thickness estimates for active modern arcs were selected. These oceanic island arcs are shown in Figure 1.
FIGURE 1. Location of the oceanic island arcs used for the regression analysis determining the relationship between crustal thickness and Bouguer anomalies. The numbers shown in the figure represent the average crustal thickness of these oceanic island arcs derived from seismic data. Bathymetric data used in the base map was extracted from GEBCO (2022).
Crustal thickness sections were digitized and georeferenced to extract the corresponding Bouguer anomalies from the EGM2008 model accurately. Profiles depicting the crustal thickness of modern or active arcs were considered in this study. The Bouguer anomaly and crustal thickness data are presented in a scatter plot generated using Matlab plotting algorithms. The scatter plot consists of 88 data points (Figure 2). These data were subjected to linear regression analyses where the dependent variable is the crustal thickness, the independent variable is the Bouguer anomaly value, the y-intercept is the constant value corresponding to the thickness of a standard oceanic arc crust, and the slope is the rate of gravity signature change per 1-km change in crustal thickness.
FIGURE 2. Crustal thickness vs. Bouguer anomaly plot for all the arc datasets utilized in this study. A strong negative correlation exists between the two variables as represented by the negative regression curve and a high coefficient of correlation at 0.7161.
A geophysical constraint was further applied to the generated equation. As discussed earlier, the y-intercept value and the slope of the line correspond to the standard thickness of oceanic arc crust and the accompanying gravity signature change per 1-km change in crustal thickness, respectively. These values are automatically generated as the best-fit line is produced. However, these can be changed or substituted by other slope and y-intercept pairs conforming to the generated line. The average crustal thickness of modern arcs (26 km) (Tetreault and Buiter, 2014) was used as a starting point. Gravity modeling was carried out using the 2D GM-SYS plugin of Oasis Montaj version 9.2 (Sharma, 1997).
The geophysically-constrained empirical formula was applied to the Bouguer anomaly grid of several oceanic island arcs to compute their respective crustal thicknesses. The resulting crustal thicknesses were used to compute the magmatic addition rate of these oceanic island arcs following the methodology introduced by Reymer and Schubert (1984). This method is summarized in the equation below:
where: CT-7 = arc crustal thickness (7-km-thick oceanic crust already removed), CSA = cross-sectional area of the arc, and age = age of initiation of subduction.
For island arcs that were included in the study of Dimalanta and others (2002), we adopted the values used for the arc area and cross-sectional areas. For the other island arcs, the areas and cross-sectional areas were calculated using ArcMap version 10.6.1. The measured areas were multiplied by the crustal thicknesses obtained by applying the generated empirical formula to the EGM2008-derived Bouguer anomalies. The 7-km average thickness of standard oceanic crust was subtracted from the crustal thickness which yielded the volume of the arc magmatic material. The volume was then divided by the arc length to determine the magmatic contribution per square kilometer. The values were then divided by the age of initiation of subduction to derive the crustal growth rate from magmatic contributions (Reymer and Schubert, 1984).
A regression plot was generated with the crustal thickness as the dependent variable and the Bouguer anomaly as the independent variable (Figure 2). The observed relationship was used to determine whether Bouguer anomalies could yield reliable estimates of the crustal thickness of oceanic island arcs. A negatively sloping best-fit line represents the relationship between the two, i.e., a high Bouguer anomaly is associated with a thin crust and vice versa. This is expected since the depth or the distance of a dense body from the observation point is a significant factor affecting the Bouguer anomaly (e.g., Weilly, 1961; Chakravarthi et al., 2007). Considering that shorter wavelength gravity signals were removed in a typical oceanic arc setting, the main density contrast occurs between the arc crustal layers and the upper mantle. A thicker arc crust means that the mantle is situated farther from the observation point (i.e., ground) when compared to thinner arc crusts. This would then cause the Bouguer anomalies at a particular observation point to decrease.
The R2 value of the plot in Figure 2 is 0.7161. This means that crustal thickness only accounts for 71% of the observed variations in the Bouguer anomalies. This is acceptable, considering that several elements within the arc crust can also cause substantial density differences. For example, seismic studies of several oceanic island arcs revealed the presence of a middle crust in oceanic island arcs. These middle arc crustal layers vary in thickness and general composition as deduced from seismic velocities. For instance, the Aleutians arc is inferred to have an andesitic to basaltic middle crust (Fliedner and Klemperer, 1999; Holbrook et al., 1999; Lizarralde et al., 2002; Shillington et al., 2004). On the other hand, the Izu-Bonin arc, represented by green squares plotting way above the regression line, is inferred to have a granitic middle crustal layer (Suyehiro et al., 1996; Takahashi et al., 2007). The presence of this granitic middle crustal layer induced a major change in the expected gravity signatures which caused the points to deviate from the regression line (Figure 1). Furthermore, there are reports associating the observed discrepancies between Bouguer anomaly-derived crustal thicknesses with those derived using seismic data. These were attributed to the effects of deep structures in subduction zone systems (e.g., Zhao et al., 1994; Lo et al., 2018). Since we adopted a constant density contrast representing the crust-mantle interface for the computations of the Bouguer anomalies, the presence of these other bodies at depth might require a revised density contrast for the calculation of the Bouguer anomalies. This might have caused the slight deviation between the Bouguer anomalies and crustal thicknesses relationship in these regions. Despite this being the case, the computed R2 still indicates that crustal thickness values can be predicted from Bouguer anomalies.
Following the concept used by Milsom and others (1996) for their empirical formula, the intercept of the equation, which in this case is 40.31 km, should represent the standard thickness of an oceanic island crust wherein a 1-km change from this thickness is accompanied by a gravity signature change equivalent to the reciprocal of the slope, which in this case is 9 mGal. However, based on available geologic data, the 40.31-km standard is too thick for oceanic island arcs. Thus, this indicates that the equation herein should be constrained using the average crustal thickness (26 km) reported by Tetreault and Buiter (2014). This will serve as a starting point for plotting the other linear equations that may fit the dataset (Figure 3). Multiple lines with set coefficients from 25 to 30 were plotted in Figure 3. These lines are characterized by their unique slope-intercept value combinations.
FIGURE 3. Plot showing the other regression curves that fit the dataset. The intercepts of the selected lines were determined following the average arc crustal thickness reported by Tetreault and Buiter (2014).
Gravity modeling of an arc lithosphere with an average arc density of 2.79 g/cm3 and an average mantle density of 3.3 g/cm3 was performed to determine which of these linear equations can satisfy the geophysical constraint. Several iterations of gravity modeling revealed that in a 29-km-thick arc crust, a 1-km change in the crustal thickness is accompanied by ∼18 mGal change in the total gravity signature. The reciprocal of 18 is 0.056, which should be the slope value for the linear equation that satisfies the results from the geophysical modeling. The closest fit among the available equations is observed with the linear equation where y = 29—0.0523x. This linear equation can be rewritten to conform with the format of the equation of Milsom and others (1996). Based on the results of the statistical analysis and geophysical constraint, the empirical formula proposed to estimate the crustal thickness of oceanic island arcs using Bouguer anomalies is as follows: CT = 29—∆gB/18, where CT = crustal thickness and ∆gB = Bouguer anomaly. Based on the results of the statistical correlation and gravity modeling, we propose a thickness of 29 km for the oceanic island arc crust, which is thicker than the 26-km crustal thickness obtained by Tetreault and Buiter (2014). Their value was derived by getting the average of the crustal thickness values from 26 island arcs, which include remnant arcs and extinct arcs as well as arcs formed from the subduction of a continental crust.
Using the crustal thickness estimates computed from regional gravity data, new arc magmatic addition rates of the oceanic island arcs in the western Pacific were computed following the methodology proposed by Reymer and Schubert (1984) (Table 1). Furthermore, since these magmatic addition rates were derived from identical data types and methodologies, a comparison between these rates can be made in a more straightforward manner. As such, the observed differences in the computed arc magmatic addition rates can be used to infer the subduction conditions affecting the magmatic productivity in these island arcs. Table 1 shows the distinction between the arc magmatic growth rates between Pacific island arcs in comparison with other island arcs. The Pacific island arcs (i.e., Bonin, Izu, Kermadec, Mariana, and Vanuatu) recorded higher growth rates (28–60 km3/km/m.y.) (Table 1). On the other hand, oceanic island arcs caused by the subduction of other younger and smaller plates (i.e., the Philippine Sea Plate, the South China Sea Plate, and the Caribbean Plate) recorded lower arc magmatic addition rates. This is clearly shown in the computed growth rates for the East Philippine Arc, Luzon Arc, Ryukyu Arc, and Leeward Antilles Arc, which range from 11 to 26 km3/km/m.y. (Table 1).
TABLE 1. New magmatic growth rates computed for oceanic island arcs using Bouguer anomaly-derived crustal thicknesses. Crustal thicknesses were computed following the methodology forwarded by Reymer and Schubert (1984).
As mentioned earlier, arc magmatic productivity can be generally influenced by: (1) the nature of subduction, which includes the rate of subduction and angle of subduction, and (2) the amount of sediment in the slab as a function of age. Regarding the rate of subduction, which can be inferred indirectly from the plate velocity, both the Pacific Plate and the Philippine Sea Plate move northwestward. They do not show significant differences in their velocities at approximately 10 cm/year (e.g., Seno et al., 1993). In terms of subducting angles, it seems that older oceanic plates are characterized by steeper slopes as compared with younger subducting slabs. For instance, the subduction of the older Pacific Plate generally shows steeper subduction angles except for some parts subducting further west. Holt et al. (2018) showed through 3-D numerical modeling of seismic data that the Pacific Plate subducting under Japan featured shallow/gentle slab dips. However, the region of the Pacific Plate subducting beneath the Philippine Sea Plate through the IBM trench system is characterized by steep to overturned slab dips (Lallemand, 2016). On one hand, the subduction of younger slabs is generally characterized by gentler slab dips. For instance, the subduction of the South China Sea (SCS) beneath the Manila trench is characterized by gentler slopes, albeit with significant differences from north to south. The northern part of the SCS subducts at a relatively shallower dip of 45° due to the subduction of a buoyant plateau. Further south, the angle of subduction becomes steeper at ∼75° (Fan et al., 2016) This is inferred to have been caused by the collision between the Philippine Mobile Belt and Palawan micro-Continental Block (PCB) at the central Philippines (Chen et al., 2015). The Philippine Sea Plate was also reported to be subducting beneath the Ryukyu Arc at a dip of ∼75° as identified from the Wadatti-Benioff zone and seismic tomography (e.g., Bijwaard et al., 1998; Widiyantoro et al., 1999; Wang et al., 2008; Wei et al., 2015). The angle of subduction of these island arcs, among other factors related to plate kinematics, is greatly influenced by the age of the subducting slab due to density variations. Previously, it has been established that the angle of subduction affects arc-trench gap (e.g., Cross and Pilger, 1982). The exact mechanism of how magmatic productivity can also be affected by the dip of subduction remains to be further investigated.
Other factors related to age that can possibly directly affect the magmatic productivity of oceanic island arcs are the sediment thickness and the degree of serpentinization in the subducting slab. The variation of sediment thickness with the age of the subducting oceanic crust might explain this phenomenon. Generally, older oceanic plates are associated with thicker sediment covers and higher degrees of serpentinization as compared to younger subducting plates (e.g., Hay et al., 1988; Olson et al., 2016; Grevemeyer et al., 2018). Sediment cover and a higher degree of serpentinization in the older oceanic crust would contribute to a higher volume of slab-derived fluid during slab dehydration at depth which in turn results in a more robust melting of the mantle wedge. Furthermore, other works have shown that dehydration of the underlying serpentinites during the subduction of a highly serpentinized oceanic crust promotes the melting of the overlying sediments (e.g., Skora and Blundy, 2010). After which, the subducting sediments partial melts will rise and interact with the overlying mantle wedge causing subduction-related magmatism (e.g., Grove et al., 2012; Cruz-Uribe et al., 2018; Forster and Selway, 2021). This higher degree of volatiles introduced to the subduction system as a function of the age of the subducting slab might explain why the computed arc magmatic addition rates for the Pacific island arcs are higher (28–60 km3/km/m.y). Previous works have shown that the subducting ∼132 Ma Pacific slab along its western margin beneath the Izu-Bonin-Mariana trench arc system (Oakley et al., 2008) is marked by significantly thick sediment covers ranging from 0.5 to 2 km (e.g., Abrams et al., 1993). Furthermore, the presence of serpentinite seamounts west of the Izu-Bonin-Mariana trench arc system is postulated to have been caused by old slab devolatilization due to the subduction of the Pacific Plate (Fryer and Hussong, 1981; Fryer and Fryer, 1987; Fryer and Mottl, 1992). Moreover, the chemistry of the vent fluids in the proximity of these serpentinite seamounts is an indication of the presence of a serpentinized mantle beneath the edifice of these volcanoes related to the IBM trench system (Oakley et al., 2008). These are pieces of evidence of the previously forwarded concept that subduction of older oceanic plates is associated with thicker sediment subduction and a higher degree of serpentinization, which in turn caused the higher arc magmatic productivity observed for the Pacific island arcs.
This is compared to the lower magmatic addition rates computed for oceanic island arcs created by the subduction of younger minor plates (11–26 km3/km/m.y). A similar distinction is also observed between the two volcanic arcs in the Philippine island arc system. The Luzon arc is characterized by a relatively lower growth rate (11–18 km3/km/m.y.) compared to the East Philippine arc, which has a growth rate of 24 km3/km/m.y. (Table 1). The Luzon arc and the East Philippine arc are products of the subduction of the South China Sea and the West Philippine Basin, respectively. The South China Sea has a proposed age of 32 Ma (Barckhausen et al., 2014), whereas the West Philippine Basin has a suggested age of 55 Ma (Deschamps and Lallemand, 2002). Moreover, reported sediment covers on the South China Sea Basin subducting beneath the Manila Trench are reported to be ranging from 100 to 1500 m. These values are deduced from seismic velocity profiles converted using a water velocity of 1,480 m/s (Hayes and Lewis, 1985; Armada et al., 2020). While the observed magmatic growth rates showed concurrence with the age of the subducting slabs, this work does not discount the role of other factors which affect the composition, structure, and thickness of different arc crusts, such as crustal anatexis (Izu-Bonin-Marianas Arc—Tatsumi et al., 2008); delamination processes (Kuril Arc—Tsumura et al., 1999); degree of back-arc spreading (Tonga Arc—Zhao et al., 1997), and the level of maturity in crustal evolution (Aleutian Arc—Holbrook et al., 1999). This work presents the latest estimates of the magmatic productivity of oceanic island arcs. Since the method proposed in this study made use of gravity data, we could provide crustal growth rates to more island arcs in comparison with previous works. In this work, the differences in the computed magmatic productivity rates for specific island arcs were attributed to the age of the subducting slab (e.g., older slabs tend to contain thicker sedimentary cover and higher degrees of serpentinization than younger ones). While our results showed concurrence with what is to be expected of the resulting magmatism based on this parameter, future works considering the other factors may improve the values derived from this study.
The values obtained from this study were compared with the results of Reymer and Schubert (1984) and Dimalanta and others (2002). As shown in Table 2, both the estimates from Dimalanta and others (2002) and this study are significantly higher compared to that of Reymer and Schubert (1984). The difference in the computed magmatic addition rates for this study and that of Reymer and Schubert (1984) represents the improvements in how crustal thicknesses are computed over time. Crustal thicknesses used in this study were derived from a more robust dataset and were guided by improved assumptions regarding the nature of oceanic island crusts. The estimates obtained by Dimalanta and others (2002) and this study are nearly identical, even though the former based their estimates on both seismic and gravity data while the latter solely based the estimates on Bouguer anomaly-derived crustal thicknesses. This might be a testament to the robustness of the empirical formula proposed in this study. Furthermore, since this study made use of estimates from gravity data, we were able to provide magmatic addition rates to more island arcs as the computations are no longer limited by seismic data availability.
TABLE 2. Comparison of the volume, age, and magmatic addition rates between Reymer and Schubert (1984), Dimalanta et al. (2002), and this study.
This work presents a new empirical formula that can be employed to estimate crustal thicknesses of oceanic island arcs using Bouguer anomalies. The new empirical formula presented in this study could be applied to areas that have limited seismic data for crustal thickness estimates (e.g., Banda and Sunda Arc). A new set of arc magmatic addition rates computed from Bouguer anomaly-derived crustal thicknesses using this formula was also generated. Oceanic island arcs directly related to the subduction of the relatively older Pacific Plate are observed to record higher magmatic addition rates compared to other oceanic island arcs. This is possibly due to the difference in the amount of volatiles released to the mantle wedge during the subduction of oceanic plates with varying ages. The distinction in the magmatic addition rates between the subduction of older and younger oceanic lithosphere is also observed in the Philippine Island Arc system. This suggests that the magmatic productivity is controlled by the nature/degree of hydration of the subducting slab, which highlights the complexity of arc magmatism in relation to different subduction conditions.
Publicly available datasets were analyzed in this study. This data can be found here: Earth Gravitational Model (EGM2008)—https://bgi.obs-mip.fr/data-products/grids-and-models/egm2008-global-model/.
NP, CD, RA, GV, and LA contributed to the conception and design of the study. NP did the statistical analysis and wrote the first draft. KS contributed to the organization of the database. BP, JG-R, and GY provided significant inputs in several sections of the manuscript. All authors contributed to the manuscript revision, read, and approved the submitted version.
This work was supported by several NIGS research grants awarded to CD.
The authors would also like to acknowledge the free access to the EGM2008 model provided by the Bureau Gravimetrique Internationale. Fruitful discussions among the members of the Rushurgent Working Group are acknowledged.
NP is employed by the Energy Development Corporation. GY is employed by the Cordillera Exploration Co., Inc.
The remaining authors declare that the research was conducted in the absence of any commercial or financial relationships that could be construed as a potential conflict of interest.
All claims expressed in this article are solely those of the authors and do not necessarily represent those of their affiliated organizations, or those of the publisher, the editors and the reviewers. Any product that may be evaluated in this article, or claim that may be made by its manufacturer, is not guaranteed or endorsed by the publisher.
Abrams, L.J., Larson, R.L., Shipley, T.H., and Lancelot, Y. (1993). Cretaceous volcanic sequences and Jurassic oceanic crust in the East Mariana and Pigafetta basins of the western Pacific. Geophysical Monograph Series 77, 77–101.
Armada, L.T., Hsu, S.-K., Dimalanta, C.B., Yumul, G.P., Doo, W.-B., and Yeh, Y.-C. (2020). Forearc structures and deformation along the Manila Trench. Journal of Asian Earth Sciences: X 4, 100036. doi:10.1016/j.jaesx.2020.100036
Assumpcao, M., Bianchi, M., Julia, J., Dias, F., Franca, G., Nascimento, R., Drouet, S., Pavao, C., Albuquerque, D., and Lopes, A. (2013). Crustal thickness map of Brazil: Data compilation and main features. Journal of South American Earth Sciences 43, 74–85. doi:10.1016/j.jsames.2012.12.009
Bai, Y., Williams, S., Muller, D., Liu, Z., and Hosseinpour, M. (2014). Mapping crustal thickness using marine gravity data: Methods and uncertainties. Geophysics 79 (2), G27–G36. doi:10.1190/geo2013-0270.1
Barckhausen, U., Engels, M., Franke, D., Ladage, S., and Pubellier, M., 2014. Evolution of the South China Sea: Revised ages for breakup and seafloor spreading 58B, 599-611.
Barker, P.F. (1995). “Tectonic framework of the east Scotia Sea,” in Backarc basins: tectonics and magmatism. Editor B. Taylor (Boston: Springer US), 281–314.
Bassin, C., Laske, G., and Masters, G. (2000). The current limits of resolution for surface wave tomography in North America. EOS Transactions American Geophysical Union 81, F897.
Braszus, B., Goes, S., Allen, R., Rietbrock, A., Collier, J., Harmon, N., Henstock, T., Hicks, S., Rychert, C.A., Maunder, B., van Huenn, J., Bie, L., Blundy, J., Cooper, G., Davy, R., Kendall, J.M., Macpherson, C., Wilkinson, J., and Wilson, M. (2021). Subduction history of the Caribbean from upper-mantle seismic imaging and plate reconstruction. Nature Communications 12, 4211. doi:10.1038/s41467-021-24413-0
Bellon, H., and Yumul, G.P. (2000). Mio-Pliocene magmatism in the Baguio Mining District (Luzon, Philippines): age clues to its geodynamic setting. Comptes Rendus de l’Academie des Sciences, Sciences de la Terre et des Planetes 331, 295–302. doi:10.1016/s1251-8050(00)01415-4
Bijwaard, H., Spakman, W., and Engdahl, R. (1998). Closing the gap between regional and global travel time tomography. Journal of Geophysical Research 103, 30055–30078. doi:10.1029/98jb02467
Byrne, D.B., Suarez, G., and McCann, W.R. (1985). Muertos Trough subduction, microplate tectonics in the northern Caribbean? Nature 317, 420–421. doi:10.1038/317420a0
Byrne, T.B., Chan, Y.-C., Rau, R.-J., and Lu, C.-Y. (2011). The Arc-Continent Collision in Taiwan. Frontiers in Earth Sciences 4, 213–245.
Carbonell, R., Levander, A., and Kind, R. (2013). The Mohorovicic discontinuity beneath the continental crust: An overview of seismic constraints. Tectonophysics 609, 353–376. doi:10.1016/j.tecto.2013.08.037
Chakravarthi, V., Mallesh, K., and Ramamma, B. (2007). Basement depth estimation from gravity anomalies: two 2.5D approaches coupled with the exponential density contrast model. Journal of Geophysics and Engineering 14 (2), 303–315. doi:10.1088/1742-2140/aa5832
Chen, P.-F., Olavere, E.A., Wang, C.-W., Bautista, B.C., Solidum, R.U., and Liang, W-T. (2015). Seismotectonics of Mindoro, Philippines. Tectonphysics 640-641, 70–79. doi:10.1016/j.tecto.2014.11.023
Concepcion, R.A.B., Dimalanta, C.B., Yumul, G.P., Faustino-Eslava, D.V., Queaño, K.L., Tamayo, R.A., and Imai, A. (2012). Petrography, geochemistry, and tectonics of a rifted fragment of Mainland Asia: evidence from the Lasala Formation, Mindoro Island, Philippines. International Journal of Earth Sciences 101, 273–290. doi:10.1007/s00531-011-0643-5
Cross, T.A., and Pilger, R.H. (1982). Controls of subduction geometry, location of magmatic arcs, and tectonics of arc and back-arc regions. GSA Bulletin 93 (6), 545–562. doi:10.1130/0016-7606(1982)93<545:cosglo>2.0.co;2
Cruz-Uribe, A.M., Marschall, H.R., Gaetani, G.A., and Le Roux, V. (2018). Generation of alkaline magmas in subduction zones by partial melting of mélange diapirs–An experimental study. Geology 46, 343–346. doi:10.1130/g39956.1
DeMets, C., Gordon, R.G., and Argus, D.F. (2010). Geologically current plate motions. Geophysical Journal International 181, 1–80. doi:10.1111/j.1365-246x.2009.04491.x
Deschamps, A., and Lallemand, S. (2002). The West Philippine Basin: An Eocene to early Oligocene back arc basin opened between two opposed subduction zones. Journal of Geophysical Research 107 (B12), EPM 1-1–EPM 1-24. doi:10.1029/2001jb001706
Dewey, J.F., and Windley, B.F. (1981). Growth and differentiation of the continental crust, A301. Philosophical Transactions of the Royal Society of London, 189–206.
Dimalanta, C.B., Taira, A., Yumul, G.P., Tokuyama, H., and Mochizuki, K. (2002). New rates of western Pacific island arc magmatism from seismic and gravity data. Earth and Planetary Science Letters 202, 105–115. doi:10.1016/s0012-821x(02)00761-6
Dimalanta, C.B., and Yumul, G.P. (2003). Magmatic and amagmatic contributions to crustal growth of an island-arc system: The Philippine example. International Geology Review 45, 922–935. doi:10.2747/0020-6814.45.10.922
Dimalanta, C.B., Ramos, E.G.L., Yumul, G.P., and Bellon, H. (2009). New features from the Romblon Island Group: Key to understanding the arc–continent collision in central Philippines. Tectonophysics 479, 120–129. doi:10.1016/j.tecto.2009.02.015
Fan, J., Zhao, D., and Dong, D. (2016). Subduction of a buoyant plateau at the Manila Trench: Tomographic evidence and geodynamic implications. Geochemistry, Geophysics, Geosystems 17 (2), 571–586. doi:10.1002/2015gc006201
Farner, F., and Lee, C.-T. (2017). Effects of crustal thickness on magmatic differentiation in subduction zone volcanism: A global study. Earth and Planetary Science Letters 470, 96–107. doi:10.1016/j.epsl.2017.04.025
Fliedner, M.M., and Klemperer, S.L. (1999). Structure of an island-arc: Wide-angle seismic studies in the eastern Aleutian Islands, Alaska. Journal of Geophysical Research 104, 10667–10694. doi:10.1029/98jb01499
Forster, M.W., and Selway, K. (2021). Melting of subducted sediments reconciles geophysical images of subduction zones. Nature Communications 12, 1320. doi:10.1038/s41467-021-21657-8
Fryer, P., and Hussong, D.M. (1981). Seafloor spreading in the Mariana Trough: Results of Leg 60 drill site selection surveys. Initial Reports of the Deep Sea Drilling Project 60, 45–55.
Fryer, P., and Fryer, G.J. (1987). Origins of nonvolcanic seamounts in a forearc environment. Geophysical Monograph Series 43, 61–69.
Fryer, P., and Mottl, M. (1992). Lithology, mineralogy, and origin of serpentine muds recovered from Conical and Torishima forearc seamounts: Results of Leg 125 drilling. Proceedings of the Ocean Drilling Prgoram Scientific Results 125, 343–362.
Granja Bruña, J.L., Carbo-Gorosabel, A., Llanes Estrada, P., Muñoz-Martin, A., ten Brink, U.S., Gomez Ballesteros, M., Druet, M., and Pazos, A. (2014). Morphostructure at the junction between the Beata ridge and the Greater Antilles island arc (offshore Hispaniola southern slope). Tectonophysics 618, 138–163. doi:10.1016/j.tecto.2014.02.001
Greene, H.G., Collot, J.Y., Fisher, M.A., and Crawford, A.J. (1994). Neogene tectonic evolution of the New Hebrides island arc: A review incorporating ODP drilling results. Proceedings of the Ocean Drilling Program, Scientific Results 134, 19–46.
Grevemeyer, I., Ranero, C.R., and Ivandic, M. (2018). Structure of oceanic crust and serpentinization at subduction trenches. Geosphere 14 (2), 395–418. doi:10.1130/ges01537.1
Grove, T.L., Till, C.B., and Krawczynski, M.J. (2012). The role of H2O in subduction zone magmatism. Annual Reviews in Earth and Planetary Sciences 40, 413–39. doi:10.1146/annurev-earth-042711-105310
Hall, R. (2002). Cenozoic geological and plate tectonic evolution of SE Asia and the SW Pacific: computer-based reconstructions, model and animations. Journal of Asian earth sciences 20 (4), 353–431. doi:10.1016/s1367-9120(01)00069-4
Harris, R. (2011). The nature of the Banda arc-continent collision in the Timor Region. Geology 12, 163–211. doi:10.1007/978-3-540-88558-0_7
Hay, W.M., Sloan, J.L., and Wold, C.N. (1988). Mass/Age distribution and composition of sediments on the ocean floor and the global rate of sediment subduction. Journal of Geophysical Research 93, 14933–14940. doi:10.1029/jb093ib12p14933
Hayes, D.E., and Lewis, S.D. (1985). Structure and tectonics of the Manila trench system, Western Luzon, Philippines. Energy 10 (3-4), 263–279. doi:10.1016/0360-5442(85)90046-5
Holbrook, W.S., Lizarralde, D., McGeary, S., Bangs, N., and Diebold, J. (1999). Structure and composition of the Aleutian island arc and implications for continental crustal growth. Geology 27, 31–34. doi:10.1130/0091-7613(1999)027<0031:sacota>2.3.co;2
Holt, A.F., Royden, L.H., Becker, T.W., and Faccenna, C. (2018). Slab interactions in 3-D subduction settings: The Philippine Sea Plate region. Earth and Planetary Science Letters 489, 72–83. doi:10.1016/j.epsl.2018.02.024
Huang, C.-Y., Wu, W.-Y., Chang, C.P., Tsao, S., Yuan, P.B., Lin, C.W., and Kuan-Yuan, X. (1997). Tectonic evolution of accretionary prism in the arc-continent collision terrane of Taiwan. Tectonophysics 281, 31–51. doi:10.1016/s0040-1951(97)00157-1
Huang, J.-P., Fu, R.-S., Huang, J.-H., and Zheng, Y. (2006). Inversion of gravity and topography data for the crust thickness of China and its adjacent region. Acta Seismologica Sinica 19, 264–272. doi:10.1007/s11589-003-0264-6
Huang, H., Zhang, Z.C., Santosh, M., Zhang, D.Y., and Wang, T. (2015). Petrogenesis of the Early Permian volcanic rocks in the Chinese South Tianshan, Implications for crustal growth in the Central Asian Orogenic Belt. Lithos 228-229, 23–42. doi:10.1016/j.lithos.2015.04.017
Hsieh, Y.-J., Liu, C.-S., Suppe, J., Bryne, T.B., and Lallemand, S. (2020). The Chimei submarine canyon and fan: A record of Taiwan arc-continent collision on the rapidly deforming overriding plate. Tectonics 39 (11), 1–36. doi:10.1029/2020tc006148
Karig, D.E., Barber, A.J., Charlton, T.R., Klemperer, S., and Hussong, D.M. (1987). Nature and distribution of deformation across the Banda Arc-Australian collision zone at Timor. Bulletin of the Geological Society of America 98, 18–32. doi:10.1130/0016-7606(1987)98<18:nadoda>2.0.co;2
Kodaira, S., Sato, T., Takahashi, N., Ito, A., Tamura, Y., Tatsumi, Y., and Kaneda, Y. (2007). Seismological evidence for variable growth of crust along the Izu intraoceanic arc. Journal of Geophysical Research 112 (B5), B05104–25. doi:10.1029/2006jb004593
Kodaira, S., Fujiwara, T., Noguchi, N., and Takahashi, N. (2011). Structural variation of the Bonin ridge revealed by modeling of seismic and gravity data. Earth, Planets, and Space 63, 963–973. doi:10.5047/eps.2011.06.036
Kroenke, L.W., Resig, J.M., and Leckie, R.M. (1993). Hiatus and tephrochronology of the Ontong Java Plateau: Correlation with regional tectono-volcanic events. Proceedings of the Ocean Drilling Program Scientific Results 130, 423–445.
Lallemand, S. (2016). Philippine Sea Plate inception, evolution, and consumption with special emphasis on the early stages of Izu-Bonin-Mariana subduction. Progress in Earth and Planetary Science 3 (15), 15. doi:10.1186/s40645-016-0085-6
Laske, G., Masters, G., Ma, Z., and Pasyanos, M. (2013). Update on CRUST1.0 - A 1-degree global model of Earth's Crust. Geophysical Research Abstracts 15–2658. Abstract EGU2013.
Lizarralde, D., Holbrook, W.S., McGeary, S., Bangs, N.L., and Diebold, J.B. (2002). Crustal structure of a volcanic arc, wide-angle results from the western Alaska Peninsula. Journal of Geophysical Research 107, B8 2164.
Lo, Y.-T., Yan, H.-Y., and Chen, C.-R. (2018). Correlation between the Bouguer gravity anomaly and the TAIGER tomography of the Taiwan region. Terrestrial, Atmospheric and Ocean Sciences 29, 473–483. doi:10.3319/tao.2018.03.01.01
Luhr, F. (1992). Slab-derived fluids and partial melting in subduction zones: insights from two contrasting Mexican volcanoes (Colima and Ceboruco). Journal of Volcanology and Geothermal Research 54, 1–18. doi:10.1016/0377-0273(92)90111-p
Lundberg, N., Reed, D. L., Liu, C. -S., and Lieskes, J.H. (1997). Forearc basin closure and arc accretion in the submarine suture zone south of Taiwan. Tectonophysics 274, 5–23. doi:10.1016/s0040-1951(96)00295-8
Luo, Q., and Zhang, G. (2018). Control of subduction rate on Tonga-Kermadec arc magmatism. Journal of Oceanology and Limnology 36, 687–699. doi:10.1007/s00343-018-7026-8
Malavieille, J., Lallemand, S.E., Dominguez, S., and Deschamps, A. (2002). Arc-continent collision in Taiwan: New marine observations and tectonic evolution, 358. Geological Society of America Special Paper, 190–213.
Manalo, P.C., Dimalanta, C.B., Faustino-Eslava, D.V., Ramos, N.T., Queaño, K.L., and Yumul, G.P. (2015). Crustal thickness variation from a continental to an island arc terrane: Clues from the gravity signatures of the Central Philippines. Journal of Asian Earth Sciences 104, 205–214. doi:10.1016/j.jseaes.2014.08.031
Manalo, P.C., Dimalanta, C.B., Ramos, N.T., Faustino-Eslava, D.V., Queaño, K.L., and Yumul, G.P. (2016). Magnetic signatures and curie surface trend across an arc-continent collision zone: An example from Central Philippines. Surveys in Geophysics 37, 557–578. doi:10.1007/s10712-016-9357-3
Mann, P., Calais, E., Ruegg, J.-C., DeMets, C., Jansma, P.E., and Mattioli, G. S. (2002). Oblique collision in the north-eastern Caribbean from GPS measurements and geological observations. Tectonics 2 (6), 7–26. doi:10.1029/2001tc001304
Maystrenko, Y., and Scheck-Wenderoth, M. (2009). Density contrasts in the upper mantle and lower crust across the continent-ocean transition: constraints from 3-D gravity modelling at the Norwegian margin. Geophysical Journal International 179 (1), 536–548. doi:10.1111/j.1365-246x.2009.04273.x
Milsom, J., Hall, R., and Padmawidjaja, T. (1996). Gravity fields in eastern Halmahera and the Bonin Arc: Implications for ophiolite origin and emplacement. Tectonics 15, 84–93. doi:10.1029/95tc02353
Mooney, W.D., Laske, G., and Masters, G., 1998. CRUST 5.1: A global crustal model at 5° × 5°°. Journal of Geophysical Research 103, 727-747, doi:10.1029/97jb02122
Nishizawa, A., Kaneda, K., Oikawa, M., Horiuchi, D., Fujioka, Y., and Okada, C. (2017). Variations in seismic velocity distribution along the Ryukyu (Nansei-Shoto) Trench subduction zone at the northwestern end of the Philippine Sea plate. Earth, Planets, and Space 69, 86. doi:10.1186/s40623-017-0674-7
Nugroho, H., Harris, R., Lestariya, A.W., and Maruf, B. (2009). Plate boundary reorganization in the active Banda Arc-continent collision: Insights from new GPS measurements. Tectonophysics 479 (1-2), 52–65. doi:10.1016/j.tecto.2009.01.026
Oakley, A.J., Taylor, B., and Moore, G.F. (2008). Pacific Plate subduction beneath the central Mariana and Izu-Bonin fore arcs: New insights from an old margin. Geochemistry, Geophysics, Geosystems 9 (6). doi:10.1029/2007gc001820
Olson, P., Reynolds, E., Hinnov, L., and Goswami, A. (2016). Variation of ocean sediment thickness with crustal age. Geochemistry, Geophysics, Geosystems 17, 1349–1369. doi:10.1002/2015gc006143
Ozawa, A., Tagami, T., Listanco, E.L., Arpa, C.B., and Sudo, M. (2004). Initiation and propagation of subduction along the Philippine Trench: Evidence from the temporal and spatial distribution of volcanoes. Journal of Asian Earth Sciences 23 (1), 105–111. doi:10.1016/s1367-9120(03)00112-3
Parcutela, N.E., Dimalanta, C.B., Armada, L.T., and Yumul, G.P. (2020). PHILCRUST3.0: New constraints in the crustal growth rate computations for the Philippine arc. Journal of Asian Earth Sciences X, 100032. doi:10.1016/j.jaesx.2020.100032
Parcutela, N.E., Austria, R.S.P., Dimalanta, C.B., Armada, L.T., and Yumul, G.P. (2022). Arc-crustal compression and its effects on the underlying mantle geometry as elucidated from the potential field signatures of the buckled Cretaceous Cebu lithosphere, Philippines. Tectonophysics 831, 229341. doi:10.1016/j.tecto.2022.229341
Plank, T., and Langmuir, C.H. (1988). An evaluation of the global variations in the major element chemistry of arc basalts. Earth and Planetary Science Letters 90, 349–370. doi:10.1016/0012-821x(88)90135-5
Rait, G.J. (2000). “Continental growth by delamination and obduction of oceanic lithosphere during the birth of a subduction zone,” in Canadian Society of Exploration Geophysicists Conference Abstracts, North Island, New Zealand, 4.
Reymer, A., and Schubert, G. (1984). Phanerozoic addition rates to the continental crust and crustal growth. Tectonics 3, 63–77. doi:10.1029/tc003i001p00063
Rupke, L.H., Morgan, J.P., Hort, M., and Connolly, J.A.D. (2004). Serpentine and the subduction zone water cycle. Earth and Planetary Science Letters 223, 17–34. doi:10.1016/j.epsl.2004.04.018
Seno, T., Stein, S., and Gripp, A. (1993). A model for the motion of the Philippine Sea Plate consistent with NUVEL-1 and geological data. Journal of Geophysical Research 98, 17941–17948. doi:10.1029/93jb00782
Sharma, P.V. (1997). Environmental and Engineering Geophysics. United Kingdom: Cambridge University Press, 475p.
Shillington, D.J., Avendonk, H.J.A.V., Holbrook, W.S., Kelemen, P.B., and Hornbach, M.J. (2004). Composition and structure of the central Aleutian island arc from arc-parallel wide-angle seismic data. Geochemistry, Geophysics, and Geosystems 5. doi:10.1029/2004gc000715
Skora, S., and Blundy, J. (2010). High-pressure hydrous phase relations of radiolarian clay and implications for the involvement of subducted sediment in arc magmatism. Journal of Petrology 51, 2211–2243. doi:10.1093/petrology/egq054
Stolk, W., Kaban, M., Beekman, F., Tesauro, M., Mooney, W., and Cloetingh, S. (2013). High resolution regional crustal models from irregularly distributed data: Application to Asia and adjacent areas. Tectonophysics 612, 55–68. doi:10.1016/j.tecto.2013.01.022
Suyehiro, K., Takahashi, M., Ariie, Y., Yokie, Y., Hino, R., Shinohara, M., Kanazawa, T., Hirata, N., Tokuyama, H., and Taira, A. (1996). Continental Crust, Crustal Underplating, and Low- Q Upper Mantle Beneath an Oceanic Island Arc. Science 272, 390–392. doi:10.1126/science.272.5260.390
Takahashi, N., Kodaira, S., Klemperer, S., Tatsumi, S.L., Kaneda, Y., and Suyehiro, K. (2007). Crustal structure and evolution of the Mariana intra-oceanic island arc. Geology 35, 203–206. doi:10.1130/g23212a.1
Tatsumi, Y., Shukuno, H., Tani, K., Takahashi, N., Kodaira, S., and Kogiso, T. (2008). Structure and growth of the Izu-Bonin-Mariana arc crust: 2. Role of crust-mantle transformation and the transparent Moho in arc crust evolution. Journal of Geophysical Research: Solid Earth 113 (B2), B02203. doi:10.1029/2007jb005121
Taylor, B. (1992). “Rifting and the volcanic-tectonic evolution of the Izu-Bonin-Mariana Arc,” in Proceedings of the Ocean Drilling Program, Scienti¢c Results, 627–651.126
Tetreault, J.L., and Buiter, S.J.H. (2014). Future accreted terranes: a compilation of island arcs, oceanic plateaus, submarine ridges, seamounts, and continental fragments. Solid Earth 5, 1243–1275. doi:10.5194/se-5-1243-2014
Tsumura, N., Ikawa, H., Ikawa, T., Shinohara, M., Ito, T., Arita, K., Moria, T., et al. (1999). Delamination-wedge structure beneath the Hidaka Collision Zone, Central Hokkaido, Japan inferred from seismic reflection profiling. Geophysical Research Letters 26 (8), 1057–1060. doi:10.1029/1999gl900192
Turner, S.J., and Langmuir, C.H. (2015). The global chemical systematics of arc front stratovolcanoes: Evaluating the role of crustal processes. Earth and Planetary Science Letters 422, 182–193. doi:10.1016/j.epsl.2015.03.056
Ulmer, P. (2001). Partial melting in the mantle wedge–the role of H2O in the genesis of mantle-derived “arc related” magmas. Physics of the Earth and Planetary Interiors 127, 215–232. doi:10.1016/s0031-9201(01)00229-1
von Huene, R., and Scholl, D.W. (1991). Observations at convergent margins concerning sediment subduction, subduction erosion, and the growth of the continental crust. Reviews in Geophysics 29, 279–316.
Whattam, S.A., Malpas, J., Ali, J. R., and Smith, I.E. (2008). New SW Pacific tectonic model: Cyclical intraoceanic magmatic arc construction and near-coeval emplacement along the Australia-Pacific margin in the Cenozoic. Geochemistry, Geophysics, Geosystems 9 (3). doi:10.1029/2007gc001710
Wang, Z., Huang, R., Huang, J., and He, Z. (2008). P-wave velocity and gradient images beneath the Okinawa Trough. Tectonophysics 455, 1–13. doi:10.1016/j.tecto.2008.03.004
Wei, W., Zhao, D., Xu, J., Wei, F., and Liu, G. (2015). PandSwave tomography and anisotropy in Northwest Pacific and East Asia: Constraints on stagnant slab and intraplate volcanism. Journal of Geophysical Research 120, 1642–1666. doi:10.1002/2014jb011254
Weilly, W.I. (1961). Gravity and crustal thickness in New Zealand. New Zealand Journal of Geology and Geophysics 5 (2), 228–233.
Widiyantoro, S., Kennett, B.L.N., and van Der Hilst, R.D. (1999). Seismic tomography with P and S data reveals lateral variations in the rigidity of deep slabs. Earth and Planetary Science Letters 173, 91–100. doi:10.1016/s0012-821x(99)00216-2
Woollard, G. (1959). Crustal Structure from gravity and seismic measurements. Journal of Geophysical Research 64, 1521–1544. doi:10.1029/jz064i010p01521
Worzel, J., and Shurbet, G. (1955). Gravity interpretations from standard oceanic and continental crust sections, 62. Geological Society of America Special Paper, 87–100.
Yumul, G.P., Dimalanta, C.B., Marquez, E.J., and Queaño, K.L. (2009). Onland signatures of the Palawan microcontinental block and Philippine mobile belt collision and crustal growth process: A review. Journal of Asian Earth Sciences 34 (2), 610–623. doi:10.1016/j.jseaes.2008.10.002
Zamoras, L.R., Montes, M.G.A., Queaño, K.L., Marquez, E.J., Dimalanta, C.B., Gabo, J.A.S., and Yumul, G.P. (2008). Buruanga peninsula and Antique Range: two contrasting terranes in Northwest Panay, Philippines featuring an arc–continent collision zone. Island Arc 17, 443–457. doi:10.1111/j.1440-1738.2008.00645.x
Zellmer, G.F., Edmonds, M., and Straub, S.M. (2014). Volatiles in subduction zone magmatism, 410. Geological Society of London Special Publications, 1–17.
Zhao, D., Hasegawa, A., and Kanamori, H. (1994). Deep structure of Japan subduction zone as derived from local, regional, and teleseismic events. Journal of Geophysical Research: Solid Earth 99 (B11), 22313–22329. doi:10.1029/94jb01149
Keywords: oceanic island arc, crustal thickness, magmatic growth rate, Bouguer anomalies, western Pacific, Philippines
Citation: Parcutela NE, Austria RS, Dimalanta CB, Valera GTV, Gabo-Ratio JAS, Payot BD, Armada LT, Sangalang KJF and Yumul GP (2023) Enhanced arc magmatic productivity of the Western Pacific island arcs deduced from gravity-derived arc crustal growth rates. Front. Earth Sci. 11:1107833. doi: 10.3389/feart.2023.1107833
Received: 25 November 2022; Accepted: 25 January 2023;
Published: 07 February 2023.
Edited by:
Liang Zhang, China University of Geosciences, ChinaReviewed by:
Changhong Wang, China University of Geosciences, ChinaCopyright © 2023 Parcutela, Austria, Dimalanta, Valera, Gabo-Ratio, Payot, Armada, Sangalang and Yumul. This is an open-access article distributed under the terms of the Creative Commons Attribution License (CC BY). The use, distribution or reproduction in other forums is permitted, provided the original author(s) and the copyright owner(s) are credited and that the original publication in this journal is cited, in accordance with accepted academic practice. No use, distribution or reproduction is permitted which does not comply with these terms.
*Correspondence: C. B. Dimalanta, Y2JkaW1hbGFudGFAdXAuZWR1LnBo
Disclaimer: All claims expressed in this article are solely those of the authors and do not necessarily represent those of their affiliated organizations, or those of the publisher, the editors and the reviewers. Any product that may be evaluated in this article or claim that may be made by its manufacturer is not guaranteed or endorsed by the publisher.
Research integrity at Frontiers
Learn more about the work of our research integrity team to safeguard the quality of each article we publish.