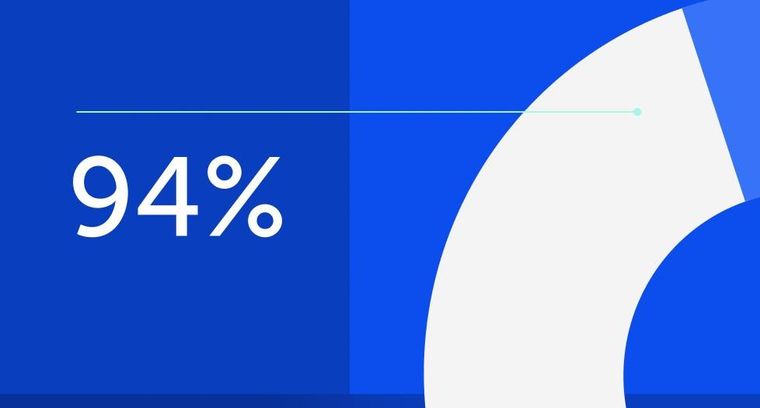
94% of researchers rate our articles as excellent or good
Learn more about the work of our research integrity team to safeguard the quality of each article we publish.
Find out more
ORIGINAL RESEARCH article
Front. Earth Sci., 09 February 2023
Sec. Cryospheric Sciences
Volume 11 - 2023 | https://doi.org/10.3389/feart.2023.1056305
This article is part of the Research TopicHydrological and Chemical Effects of a Changing Cryosphere on Mountain FreshwatersView all 9 articles
In the current context of climate change, rock glaciers represent potentially important water resources due to the melting of ice they contain and/or their role as high mountain water reservoirs. However, the hydrology of these high-altitude debris accumulations is poorly known. Understanding the origin and quality of rock glacier outflows is essential to evaluate their contribution and impact on headwater systems. In this study, we developed a conceptual model explaining the main hydro-chemical processes in active rock glaciers in the current context of permafrost warming. This conceptual model was derived from isotopic and physico-chemical analyses performed on six rock glacier outflows in the Swiss Alps during the warm season. Similar chemical and isotopic analyses were performed in sources not fed by rock glaciers at all study sites. The ion content (SO42-, Ca2+, Mg2+ and NO3−) of the water emerging from active rock glaciers was globally higher than that of sources not fed by rock glaciers. Besides, the electrical conductivity and the ion content (SO42-, Ca2+ and Mg2+) of the active rock glacier springs increased during the warm season, tracking the increasing perennial ground ice melting. We hypothesized that the ionic fingerprint of melting ice points mainly to the remobilization of chemical compounds stored during a colder period of the past in the cryosphere (e.g., the 1960s–1980s).
Mountains are often portrayed as water towers due to their important water supply, especially in semi-arid and arid regions (Viviroli et al., 2007). However, mountains play a crucial role in lowland hydrology even in the alpine areas, where the snow and ice melting in the warm season provides considerable water supply (Messerli et al., 2004). Water resources in high mountain regions—such as glaciers, snow and permafrost ice—are, however, affected by both the increase in atmospheric temperatures and changes in precipitation patterns and regimes consequent to climate change (e.g., Haeberli and Beniston, 1998; Seidel et al., 1998; Beniston and Stoffel, 2014). Permafrost undergoes accelerated degradation, as evidenced by the continuous increase in active layer thickness, the warming of ground temperatures at depth and the increase of the water-to-ice content ratio observed at different permafrost sites in recent years through thermal and geophysical methods (Marmy et al., 2016; Biskaborn et al., 2019; Mollaret et al., 2019; PERMOS 2022). However, unlike glaciers, permafrost can benefit from thermal offset conditions created by snow and/or superficial debris layers (i.e., the active layer; Giardino et al., 2011). Consequently, the ice loss from ground ice and debris-covered glaciers in the European Alps by the middle of the 21st century is expected to be smaller than for surface glaciers (Haeberli et al., 2017). In contrast to glaciers, however, the link between permafrost degradation and its impact on the hydrological cycle of Alpine catchments is less clear. On the one hand, rock glaciers represent potentially important water reservoirs due the temporary storage of liquid water in the unfrozen pore space. In addition, the storage capacity of active and inactive rock glaciers could increase in the future, as the ground ice melt will potentially increase the pore space available for the accumulation of liquid water (Wagner et al., 2021a; Wagner et al., 2021b). On the other hand, the contribution of rock glaciers to headwater systems can be given directly by the melting of the ice they contain (e.g., Duguay et al., 2015; Jones et al., 2018; Winkler et al., 2018). In fact, rock glaciers can have two types of solid water storage: a small temporary reservoir composed of seasonal ground ice in the active layer, which is aggraded and melted seasonally; a long-term reservoir formed by the perennial ground ice of the permafrost body, which is more resilient to external climatic conditions (Dunguay et al., 2015). Krainer et al. (2015) estimated that water supply due to perennial ground ice melting was about 2% of the mean discharge of the Lazaun rock glacier outflow (Austrian Alps). Harrington et al. (2018) obtained similar results for the water emerging from the Helen Creek rock glacier (Canada), where the contribution of the perennial ice melting was between 3% and 5% in July-August. However, knowledge about the contribution of ice melting in water emerging from rock glaciers is still limited. The uncertainty about the hydrological role of permafrost degradation arises from the fact that tracing the ice melt in rock glacier outflows is an indirect and technically difficult task.
The water supply related to ice melting in rock glacier springs can potentially be detected from some physical and chemical cues (for a literature review, see Colombo et al., 2018b; Jones et al., 2019). For instance, some studies linked the isotopic signature (δ18O) of rock glacier outflows during the warm season to the water supply related to the ground ice melting (Williams et al., 2006; Krainer et al., 2007; Reato et al., 2022). Similarly, the electrical conductivity and ionic content in the water emerging from rock glaciers typically increase during the warm season and more generally over recent years, which could also indicate an increase in water supply related to ground ice melting. According to some studies, the increased melting of rock glacier ice promotes the chemical weathering resulting from the contact between air/water and freshly exposed rock surfaces (e.g., Williams et al., 2006; Ilyashuk et al., 2014; Colombo et al., 2018a; Ilyashuk et al., 2018; Brighenti et al., 2019; Steingruber et al., 2020; Brighenti et al., 2021). Moreover, permafrost degradation can also increase microbial activity within the rock glacier, by promoting the release of NO3− in the internal water flows (e.g., Williams et al., 2007; Barnes et al., 2014). Finally, solutes released by rock glaciers may also have an atmospheric origin. In fact, the atmospheric deposition of pollutants and eolian dust can affect the hydrochemistry of mountain watersheds (e.g., Rogora et al., 2016; Litaor, 2022). According to Scapozza et al. (2020a), the cryosphere can store these chemical compounds and release them when the ice undergoes melting. Therefore, the loss of ice from rock glaciers has the potential to affect both the quantity and quality of mountain headwaters.
The objective of this study is to improve the knowledge on ice melt tracing in rock glacier outflows, in order to understand the hydrological role played by rock glaciers in periglacial watersheds and to quantify the impact of ground ice melting on the hydrochemistry of Alpine water ecosystems. The research question is two-fold: 1) Is the chemical and isotopic composition of rock glacier springs significantly different from that of streams not fed by rock glaciers? 2) Can the physico-chemical and isotopic composition of rock glacier springs trace the water origin during the warm season? To this aim, we investigated six rock glaciers, with the objective of identifying potential ice melt tracers through a spatial comparison between sources and sites. We also studied the temporal analysis of the hydrological functioning of one of the rock glaciers investigated. Sampling and monitoring of the springs were achieved during the warm season of 2020, from June/July to October. Finally, we developed a conceptual model of the hydro-chemical processes taking place in active rock glaciers in the current context of air, ground and permafrost temperature warming.
The rock glaciers selected for this study are located in the Lepontine Alps (Ticino Canton) and in the Pennine Alps (Valais Canton) in Switzerland (Figure 1). Each rock glacier has a flow emerging from its front. The main characteristics of these rock glaciers are summarized in Table 1. Regarding the climatic context, Mean Annual Air Temperature (MAAT) and Mean Annual Precipitation (MAP) in the Pennine Alps registered by the MeteoSwiss weather station of the Col du Grand Saint Bernard (located 27 km away from Lac des Vaux site) were −0.1°C and 2472 mm respectively for the period 1991–2020 (Table 2A). In the Lepontine Alps, meteorological stations are located at low altitude and for this reason it is necessary to estimate MAAT and MAP above 2000 m asl through vertical gradients of temperature and rainfall. The MeteoSwiss stations of Acquarossa/Comprovasco, Piotta and Robièi were the closest weather stations to the northern Ticino rock glaciers with long historical series of data. Estimated MAAT ranged between 3.1°C and 3.4°C at 2000 m asl, 0.6°C and 0.9°C at 2500 m asl, and −2.0 and −1.6°C at 3000 m asl for the period 1991–2020. Estimated MAP ranged between 1725 and 2446 mm at 2000 m asl, 1880 and 2601 mm at 2500 m asl, and 2035 and 2756 mm at 3000 m asl for the period 1991–2020 (Table 2A). Generally, the snow cover persists on the ground from October/November to June/July at the different study sites. Between October 2019 and June 2020, Campolungo/Fontane (located next to Lago di Leìt rock glacier) and Piano del Simano (next to Alpe Pièi rock glacier) stations of the Institute for Snow and Avalanche Research SLF recorded lower maximum daily snow depths than the 2011–2020 multi-year average, with values of 227 cm and 203 cm, respectively (Table 2B). In contrast, Lac des Vaux SLF station (located next to Lac des Vaux rock glacier) recorded a maximum daily snow depth of 255 cm between October 2019 and June 2020, which is above the 2011–2020 multi-year average.
TABLE 2. Climatic parameters of the study regions. A) Mean Annual Air Temperature (MAAT) and Mean Annual Precipitation (MAP) for different weather stations for the reference period 1991–2020. Estimated MAAT and MAP at 2000, 2500 and 3000 m asl in Lepontine Alps were calculated through a vertical gradients of temperature (0.5°C/100 m) and rainfall (0.31 mm/m) proposed by Scapozza et al. (2015). Data: MeteoSwiss. B) Maximum snow depth between October 2019 and June 2020 for Campolungo/Fontane, Piano del Simano and Lac des Vaux stations. 2011–2020 multi-year averages of the maximum snow depth measured between October and June are also reported. Data: Swiss Federal Institute for Snow and Avalanche Research SLF.
For each study site, two types of water sources were monitored. The first one corresponded to springs located at the front of rock glaciers (hereafter referred as to RG for “rock glacier”), whereas the second type corresponded to springs located within the same catchment but in areas where permafrost presence is unlikely (hereafter referred as to non-RG for “non-rock glacier”). The presence of permafrost was evaluated through potential permafrost distribution maps developed by Deluigi et al. (2017) for the Western Valais Alps and by Deluigi and Scapozza (2020) for the Ticino Alps (Table 3).
The Lac des Vaux site is located in the Verbier ski domain and covers an altitude range of approximately 2500–3000 m asl. The lithology of this site is mainly characterized by gneisses, igneous rocks and prasinites that belong to the Mont Fort Nappe (Swisstopo, 2020). The site features an active rock glacier of about 14.8 ha (Figure 2A). Geoelectrical surveys and ground surface temperature data suggest that permafrost conditions, and more generally ground ice, are present in most of the landform (Lambiel, 2006; Scapozza, 2013). A spring located at the rock glacier front (“Vaux RG”) forms the La Fare stream, which in turn feeds the lake. Another stream, located in the western area of the rock glacier, was used as the non-rock glacier counterpart (“Vaux non-RG”). The Vaux RG site was sampled halfway between the rock glacier front and the lake because the source was too widespread further upstream.
FIGURE 2. The rock glaciers and ice-patches investigated in this study: (A) Lac des Vaux active rock glacier, (B) Lago Nero ice patches, (C) Lago di Leìt active rock glacier, (D) Ganoni di Schenadüi active rock glacier, (E) Alpe Pièi inactive rock glacier and (F) Alpe di Sceru I relict rock glacier. Photo (A): C. Lambiel, photos (C) and (D): C. Del Siro, photos (B), (E) and (F): C. Scapozza.
The site of Lago Nero is situated in the northern part of the Val Bavona and covers an altitude range of approximately 2400–2800 m asl. This study site is located in the Maggia Nappe and is essentially characterized by leucocratic gneiss composed by biotite and plagioclase, conglomeratic gneiss, banded gneiss and polycyclic gneiss (Scapozza et al., 2020a). The site presents an active rock glacier and some ice-patches located southeast of the lake (Figure 2B). The development of the ice-patches took place mainly between 1960–1980, namely during a period favorable to ice aggradation (Scapozza et al., 2020a). In this study, the spring located downstream of the ice-patches (“Nero IP”) was investigated. According to Scapozza et al. (2020a), who analyzed the local permafrost distribution and the impacts of its degradation on the hydro-chemical characteristics of the Lago Nero watershed, Nero IP spring is fed by water from an area characterized by ice-patches surrounded by periglacial terrains, which contain very likely large amounts of ground ice. For this reason, the Nero IP spring has been associated with the springs of active rock glaciers. The water flowing over a rock wall was considered as the non-rock glacier counterpart (“Nero non-RG”).
The study site of Lago di Leìt is located in the area of Passo Campolungo, which is located in the western part of the Valle Leventina and covers an altitude range of 2200–2680 m asl (Pizzo Lei di Cima). The lithology of this site is characterized by gneiss (including plagioclase gneiss), quartz rich schists, two-mica schists and amphibolite belonging to Simano nappe (Bianconi and Strasky, 2015). An active rock glacier of about 5.4 ha is located southeast of the lake (Figure 2C). Several water springs emerge from its very steep front. Temperature measurements of these springs indicated probable permafrost occurrence in this area (Deluigi and Scapozza, 2020). Steingruber et al. (2020) also measured very high sulfate and base cation concentrations in the springs. In this study, a water source located at the rock glacier front (“Leìt RG”) and the water emerging from a rock fissure east of the front (“Leìt non-RG”) were investigated.
Val Cadlimo is a side valley located between the Lukmanier Pass and the Gotthard Pass. This study site covers an altitude range of 2400–2746 m asl (Schenadüi) and is composed entirely of Streifengneis, namely leucocratic gneiss containing alkaline feldspar and muscovite, belonging to Gotthard nappe (Bianconi and Strasky, 2015). The site is characterized by the presence of the active rock glacier of Ganoni di Schenadüi, whose area is about 7.3 ha (Figure 2D). The source located at the rock glacier front (“Schenadüi RG”) and water from a stream mainly on bedrock east of the rock glacier (“Schenadüi non-RG”) were investigated in this study.
The study site of Alpe Pièi is located in the eastern part of the Valle di Blenio and covers an altitude range of 2300–2842 m asl (Cima di Gana Bianca). The lithology of this site is fully characterized by Gana Bianca orthogneisses, which generally contain white micas, plagioclases, alkaline feldspars and, locally, biotite and carbonate nodules, belonging to Simano nappe (unpublished data supplied by M. Cavargna). This area presents a large rock glacier of about 18.4 ha characterized by an upper lobe that is superimposed on the main lobe of this periglacial landform (Scapozza and Reynard, 2007; Figure 2E). Low horizontal velocities measured from 2009 indicate that this rock glacier is inactive (Scapozza et al., 2014). The temperatures of the springs indicate the probable/possible occurrence of permafrost in the western part of the front but the unlikely occurrence of permafrost for the eastern part of the front (Scapozza and Reynard, 2007). Sites investigated for this study were the spring in the central part (“Pièi RG”) and the one in the eastern part of the front (“Pièi non-RG”), but fed by waters coming from the eastern part of the catchment, partially on bedrock.
The Valle di Sceru is located in the eastern part of the Valle di Blenio and covers an altitude range of 2000–2786 m asl (Cima di Gana Rossa). This valley is entirely constituted by feldspar rich-paragneisses belonging to Simano nappe (Scapozza et al., 2011). The extended rock glacier complex (about 37 ha) is constituted by protalus ramparts, as well as active and relict rock glaciers. The active rock glacier of Piancabella is part of the Swiss Permafrost Monitoring Network PERMOS (e.g., PERMOS, 2019). Past mapping, geophysical and hydrological studies in this region have identified a different permafrost occurrence between the left (unlikely) and right (probable) sides of the valley (Scapozza, 2008; Scapozza, 2009; Scapozza et al., 2011; Mari et al., 2013). The monitored sites (Sceru RG and Sceru non-RG sources) at the front of the large relict rock glacier of Alpe di Sceru I (Figure 2F) were chosen accordingly.
Continuous monitoring was applied only to the rock glacier springs, from June/July to October 2020, while individual sampling was manually done at all sources on three dates over the warm season: in early summer (June/July), in late summer (August) and in early autumn (October). Nevertheless, at some study sites, the sampling location varied during the season due to the presence of snow. At the Lac des Vaux site, the persistence of snowpack in early July and early snowfall in October prevented the collection of the Vaux non-RG samples at the same location during the warm season. At the Lago Nero site, because of heavy snowfalls in early October, the last sample from Nero IP was collected further downstream than previous samples. Similarly, the last sample of Nero non-RG was collected further downstream from the rock face: water in form of ice was collected because all streams were frozen, except the one emerging from the ice-patches. Finally, at Ganoni di Schenadüi site, the last sample at Schenadüi non-RG was collected further downstream than the previously collected samples due to the heavy snowfall in early October (Table 3).
Physical parameters such as the water temperature and the electrical conductivity can indicate the possible degradation of the permafrost. Water temperature is a simple method to examine the permafrost occurrence in Alpine watersheds through thresholds values (Haeberli, 1975; Carturan et al., 2016). In addition, an increase in electrical conductivity in rock glaciers outflows during the warm season could indicate progressive melting of the rock glacier ice (e.g., Krainer et al., 2007; Thies et al., 2013; Colombo et al., 2018c). In this study, temperature-conductivity sensors (Hobo Fresh Water Conductivity data logger, respective accuracies 0.1°C and 5 μS/cm) were installed at rock glacier outflows at the beginning of the 2020 warm season, using a sample rate of one hour. All electrical conductivity data are reported at 25°C (specific conductance). Furthermore, punctual and seasonal variations in discharge normally indicate a change in the water supply of rock glacier outflows (e.g., Krainer and Mostler, 2002; Berger et al., 2004; Krainer et al., 2007). Water height of La Fare was then measured hourly using a pressure sensor (Hobo Water Level datalogger, accuracy 0.5 cm), installed at the Vaux RG site. A water height-discharge calibration curve was established from salt tracings, which were performed on seven dates during the 2020 warm season: July 9 and 21, August 4 and 20, September 13, and October 1 and 13 (cf. Franchini, 2022).
Water samples from rock glacier outflows and streams not fed by rock glaciers were manually collected at all study sites. The collected water was filtered through a 0.45 µm pore size filter directly in the field, stored and frozen in the laboratory until the analyses (within the following 6 months). The concentrations of major ions (cations and anions) were measured in duplicates by ion chromatography (Metrohm ion chromatography 930 Compact IC Flex instrument, whose detection limit is about 0.1 mg/L). Total carbon was measured on a TOC-L Shimadzu instrument (detection limit: 0.5 mg/L). All analyses were performed in a laboratory at the University of Lausanne. In this work, only sulfate (SO42-), nitrate (NO3−), calcium (Ca2+), magnesium (Mg2+), total inorganic carbon (TIC) and total organic carbon (TOC) analyses were reported.
The water origin can also be defined through daily and seasonal variation of the isotopic signature. In particular, the ratio of stable isotopes oxygen-18 and oxygen-16 (the so-called δ18O) allows to trace the temperature at which water vapor is condensed in the atmosphere. The decrease in temperature of a vapor mass causes the gradual loss of water from the cloud due to condensation process. Condensation initially affects isotopically enriched water vapor in the cloud. Continued cooling of the vapor mass will also lead to condensation of the isotopically depleted water remaining in the cloud, which will fall as snow (e.g., Clark and Fritz, 1997). Because of these isotope fractionations, snow is therefore isotopically more depleted in 18O than rain. Hence, the oxygen isotopic composition of the springs was measured in this study in order to discriminate water origins, mainly differentiating between liquid and solid precipitation. Sampling was conducted manually for both RG and non-RG springs at all sites, except for the Vaux RG source.
Car accessibility to the Lac des Vaux rock glacier allowed the installation of an automated water sampler (1 sample per day). Snow samples were also collected and analyzed (here named “S samples”, cf. Table 4). All water samples for isotopic analyses were stored in a refrigerator of the laboratory until the analyses. The analyses were performed in the Stable Isotope Laboratory of the University of Lausanne between November 2020 and January 2021. The isotopic composition of the water was determined with an analytical system of Wavelength Selective Cavity Ringdown Spectroscopy of Picarro L-2140i (precision is ± 0.025‰).
TABLE 4. Measured parameters in samples collected at the rock glacier outflows (RG), at the ice-patches outflow (IP) and at the streams not fed by rock glaciers (non-RG). The isotopic values for the snow samples (S) are also reported.
Differences in water ion/isotopic values across study sites, sampling dates (early summer, late summer and early autumn) and springs (RG versus non-RG springs) were tested using a three-way ANOVA with fixed effects (study sites X sampling dates X springs and including the interaction between sites/dates and springs). The normality of residuals and the homogeneity of variances were visually checked using the QQ plots and the residuals versus fitted plots, respectively. Statistical analyses were performed with the programming language R (R Core Team, 2022) considering a significance level (α) of 0.05.
The seasonal evolution of the water electrical conductivity and temperature varied between sites, depending on the degree of activity of the rock glacier (Figure 3; Figure 4). The missing data correspond to periods when the sensor was out of the water due to low flow level. Electrical conductivity values of water emerging from active rock glaciers were characterized by a strong daily variability in early summer (June and July). The electrical conductivity of these springs also increased throughout the warm season (Figure 3). For example, at the Nero IP source, the electrical conductivity measured in early autumn was five times greater than the values recorded in early summer. In contrast, electrical conductivity was constant at the Sceru RG source (around 140 μS/cm) and decreased at the Pièi RG source over the season. The temperature of the water emerging from the Lac des Vaux, Ganoni di Schenadüi and Alpe Pièi rock glaciers (the latter only at the beginning of the measurements) showed strong daily variations, with maximum values in the afternoon and minimum values in the early morning (Figure 4). Nero IP and Leìt RG sources had steadier and colder (<1.5°C) water temperatures than the other sites, with yet some peaks and drops in temperature. The temperature recorded at Sceru RG source showed a stable value around 2°C throughout the warm season. From the graphs of the electrical conductivity and temperature, sudden spikes due to precipitation were observed but the response of physical parameters to rainfall events is described in detail through the high-resolution observation of the Lac des Vaux rock glacier (Figure 5). The hydrograph for La Fare showed that the flow was characterized by an overall decreasing trend over the warm season, with a clear diurnal cyclicity recorded in early summer and discharge peaks observed in the hours after a rainy event (Figures 5A–D). At the beginning of the monitoring, the water emerging from the rock glacier showed low δ18O values (around −14‰). From July 20 there was a rapid increase in the isotopic content of water, then from the beginning of August onwards the values stabilized around −13/−12.5‰, plateauing until the end of the observations. However, several δ18O peaks were observed throughout the warm season (Figure 5B). These peaks, which correspond to a rapid enrichment in 18O in water, often occurred after rain event (Figure 5D). Those were visible around July 11, 23 and 29, August 3, 13 and 29, and September 12. Electrical conductivity tended to increase slowly throughout the warm season (Figure 5C). While values varied between 40 and 50 μS/cm in early summer, by August 20 the electrical conductivity fluctuated around 60 μS/cm. At the beginning of the observation period, daily fluctuations in electrical conductivity were also observed and were negatively correlated to daily cyclicity in discharge. Several drops in electrical conductivity were also recorded by the instrument around July 21, August 3, 12, 29, September 11, 23 and October 3. They always corresponded to rainfall peaks measured by the Lac des Vaux SLF station (Figure 5D).
FIGURE 3. Hourly monitoring of the electrical conductivity in RG sources. Manual measurements of the electrical conductivity through a digital conductivity sensor are also indicated.
FIGURE 4. Hourly monitoring of the temperature in RG sources. Manual measurements of temperature through a digital temperature sensor are also indicated.
FIGURE 5. High-resolution monitoring at Vaux RG spring: (A) discharge (source: Franchini, 2022), (B) isotopic content (missing data correspond to samples that were lost due to instrument problems) and (C) electrical conductivity. Hourly precipitation measured at the Lac the Vaux station (D) are also reported (source: Swiss Federal Institute for Snow and Avalanche Research SLF). Grey bars indicate correlation between major rainfall events and changes in measured parameters.
The chemical and isotopic composition measured in the water samples is summarized in Table 4. The dominant ion measured in the water emerging from rock glaciers and ice-patches was sulfate (SO42-), with the exception of the Lac des Vaux and Alpe Pièi sites, where the dominant ion was calcium (Ca2+). Globally, the ion content measured at the Valle di Sceru site were very high. In contrast, the ion concentrations measured in Alpe Pièi springs were very low (<7 mg/L). A three-way ANOVA test was performed on all ion/isotopic parameters. For the NO3− variable, the residuals versus fitted plot showed outliers belonging to Alpe Pièi site. For this reason, the NO3− values measured at this study site were removed in order to meet the homogeneity of variances assumption. The ANOVA test showed significant effects of study sites, sampling dates (early summer, late summer and early autumn) and springs (RG versus non-RG springs) on ion/isotopic values (Table 5). In particular, the three sampling dates were associated with significant differences in SO42-, Ca2+, Mg2+, NO3− and δ18O values. In addition, the source factor affected significantly SO42-, Ca2+, Mg2+ and NO3− values. For sulfate values, moreover, the effect of source type depended on the study site. No parameter showed a significant interaction between sampling dates and source types. In contrast, the site factor had a significant effect on all parameters (except for TOC values), indicating the presence of local specificities (see Chapters 4.2.1 and 4.2.2).
TABLE 5. p-values of the three-way ANOVA performed on ion/isotopic values measured in water samples. Asterisk indicates the values below the significance level alpha (α = 0.05).
Site-specific differences and detailed seasonal trends are presented in Figure 6 and Figure 7. Chemical and isotopic parameters were standardized using Z-scores in order to be compared over similar scales. As shown by the ANOVA test, SO42-, Ca2+ and Mg2+ values varied strongly between sampling dates. In particular, these chemical parameters increased progressively throughout the warm season in the water emerging from active rock glaciers. In fact, for example, SO42- values detected in Schenadüi RG and Nero IP in early autumn were respectively about 9 and 3 times higher than those measured in early summer. Sceru RG also showed a seasonal increase in SO42-, Ca2+, Mg2+ concentrations but the increase factor (of 1.3 times for these ions) between early summer and early fall was smaller than that observed for active rock glacier springs (cf. Table 4). In addition, Vaux RG and Nero IP springs also showed a progressive increase in NO3− concentrations throughout the warm season, whereas an important seasonal increase in TIC values was observed only in the Vaux RG spring. In contrast, Pièi RG as well as all non-RG sources were characterized by a less clear seasonal trend in ion content. Regarding the isotopic content, water samples of RG and non-RG sources had a very low δ18O values in early summer, similar to those measured in snow samples (cf. Table 4). After the snowmelt season, the values tended to strongly increase in the water emerging from rock glaciers between early and late summer, with the exception of Sceru RG spring. In contrast, between late summer and early fall δ18O values tended to remain rather stable, with slight increases or decreases (<± 0.6‰), with the exception of Pièi RG, whose isotopic content decreased by 1.1‰. The non-RG springs followed a similar trend, although major changes in isotopic content (>± 0.7‰) were observed between late summer and early fall at Lago Nero, Ganoni di Schenadüi and Valle di Sceru sites. In contrast, Sceru RG and Vaux non-RG sources showed a different seasonal evolution, characterized by a fairly constant isotopic content throughout the warm season.
FIGURE 6. Chemical parameters measured in RG springs (left column) and non-RG springs (right column) during the warm season. Values were standardized using Z-scores.
FIGURE 7. δ18O values measured in RG springs (left column) and non-RG springs (right column) during the warm season. Values were standardized using Z-scores.
The spatial comparison between sources and study sites is represented in detail in Figure 8. As already suggested by the ANOVA test, the type of source significantly affected SO42-, Ca2+, Mg2+ and NO3− values. In detail, the median concentrations of these chemical elements measured in RG springs were greater than those detected in non-RG springs during the warm season. This trend is clearly visible in sites with active rock glaciers, with the exception of Lac des Vaux site. In fact, for example, the concentrations of sulfates and calcium measured in Leìt RG in early autumn were 2.5 times and about 2 times greater than those detected in Leìt non-RG, respectively. Similarly, the concentrations of the same ions measured in Schenadüi RG in early autumn were 15 and 4 times higher than those determined in Schenadüi non-RG, respectively (cf. Table 4). At the Valle di Sceru site, SO42-, Ca2+, Mg2+ and NO3− median values measured in the RG source were also greater than those detected in the non-RG source. In contrast, at the Alpe Pièi site, there was little difference in SO42-, Ca2+, Mg2+ and NO3− values between RG and non-RG sources. Similarly, at the Lac des Vaux site, differences between sources were less pronounced, with the exception of NO3− values, which were clearly higher in the RG source than in the non-RG source. Highest TIC concentrations were measured at the Lac des Vaux site, whereas sources located at Valle di Sceru and Lago di Leìt sites did not show significant TOC values. Significant values of total organic carbon were mainly observed in RG sources located at the Alpe Pièi, Ganoni di Schenadüi, Lago Nero and Lac des Vaux sites. At Ganoni di Schandüi site, median values of δ18O measured in RG and non-RG sources were rather similar. Leìt and Nero non-RG sources were on average more enriched in 18O than RG/IP sources during the warm season. On the contrary, at the Valle di Sceru, Lac des Vaux and Alpe Pièi sites, the median values of δ18O measured in RG sources were higher than those detected in non-RG sources.
FIGURE 8. Distribution of ion and isotopic values measured in RG and non RG springs during the warm season.
Water emerging from active rock glaciers showed a seasonal increase in electrical conductivity and, at the Vaux RG spring, a seasonal decrease in flow, which is consistent with trends observed in other studies (e.g., Krainer and Mostler, 2002; Berger et al., 2004; Krainer et al., 2007; Thies et al., 2013). Low electrical conductivity and high flow measured in early summer in rock glacier outflows were due to snowmelt. The latter also caused daily fluctuations in electrical conductivity (where minimum values were typically observed in the evening) and discharge (where maximum values were typically observed in the evening) of the water emerging from Lac des Vaux rock glacier. This negative correlation between EC and discharge was also observed in water emerging from rock glaciers investigated by Krainer et al. (2007) and Wagner et al. (2021b). The seasonal increase in electrical conductivity in the water emerging from active rock glaciers could indicate an increasing ground ice melting during the warm season. Indeed, once the snow has completely melted, the atmospheric heat can penetrate into the ground and generate thaw in the active layer. This seasonal thaw will gradually increase during the warm season, which may have two effects on the hydrochemistry of the water emerging from active and inactive rock glaciers: 1) an ion release through the perennial ground ice melting (e.g., Thies et al., 2007), because ice can temporarily store air pollutants arising from atmospheric fallout (Scapozza et al., 2020a); 2) an increase in freshly exposed mineral surfaces within rock glaciers in contact with air/water, which promotes the chemical weathering process (Williams et al., 2006; Ilyashuk et al., 2014; Ilyashuk et al., 2018; Colombo et al., 2018b). In addition, the increase in the seasonal thaw depth also changes internal flow paths, by decreasing the flow velocity of the water through rock glaciers (Buchli et al., 2013) but, at the same time, increasing the contact time between rocks and water and thus enhancing chemical weathering processes. This seasonal trend in electrical conductivity was not observed in the water emerging from the inactive rock glacier of Alpe Pièi, where the ice body might possibly be now located at greater depth. In contrast, the hydrological functioning of relict rock glaciers is expected to differ from active and inactive rock glaciers, with greater storage capacity and longer average residence times for water flowing through their internal structure (see e.g., Wagner et al., 2016; Winkler et al., 2016). Indeed, the rather constant values of electrical conductivity and temperature measured at the Sceru RG spring during the warm season, suggest that water was probably mixed for a long time in an aquifer before exiting. The possible presence of an aquifer in southern part of the rock glacier has been suggested by Scapozza (2008) and Scapozza et al. (2008) on the basis of self-potential profiles carried out in the Valle di Sceru. Despite the possible storage of water in the body of the rock glacier, major rainfall events caused decrease in water electrical conductivity at the Sceru RG source, which is consistent with the variations observed by Winkler et al. (2016) in the water emerging from the Schöneben relict rock glacier (Austrian Alps). High-resolution monitoring at the Lac des Vaux rock glacier allowed to analyze in detail the responses of physical parameters to major rainfall events. Consistent with the observations of Krainer et al. (2007) and Harrington et al. (2018), liquid precipitation caused rapid decreases in electrical conductivity in water emerging from the rock glacier, through a dilution effect of dissolved ions (Colombo et al., 2018b). Flow rates measured in La Fare also followed episodic variations: in particular, peaks were observed in the hours following a rainy event, consistent with measurements made in other studies (Krainer and Mostler, 2002; Berger et al., 2004; Krainer et al., 2007). These observations suggest that most meteoric water is rapidly released by the rock glacier, in the form of surface runoff (also called suprapermafrost flow) that flows over the surface of the ice present in the active layer or in the permafrost (Krainer and Mostler, 2002). However, some meteoric water can also seep through the ice/air and rock matrix or through taliks, forming intrapermafrost flows (Vonder Mühll, 1992; Scapozza, 2008) that feed the subpermafrost flow. In fact, Krainer et al. (2007) observed that the active rock glacier of Reichenkar (Austrian Alps), in the month of June, released only between 40% and 68% of the total precipitation within 48 h after the rainy event. In addition, tracer tests with fluorescent dye (Uranine) performed at the Lac des Vaux rock glacier in the summer of 2020 by Franchini (2022) showed that the hydrological functioning changed throughout the warm season. In early summer, high water flow velocity (115 m/h) through the rock glacier indicated that water flowed rapidly over an impermeable surface composed of seasonal ice located at the base of the active layer. Progressive ground thawing during the warm season allowed the opening of pores previously closed by ice. This, in turn, allowed greater water infiltration into the rock glacier body causing lower flow velocity measured in late summer (70.8 m/h) and in early autumn (35.4 m/h). A similar decline in water flow velocity through the rock glacier over the summer season was also observed by Buchli et al. (2013) in the Turtmann Valley (Swiss Alps), where values decreased from 43.4 m/h (early July) to 15.3 m/h (late August) according to the tracer tests with Rhodamine G. The constant water temperature of about 2°C measured at the Sceru RG spring is at boundary between possible and unlikely permafrost occurrence based on the thresholds defined by Haeberli. (1975). The cold temperatures recorded at this site could therefore be related to cold ground condition. Indeed, apparent resistivity profiles realized by Scapozza (2008) and Scapozza et al. (2011) showed the possible presence of an ice core in the southern lobe of the rock glacier, which may have been preserved through internal ventilation. Furthermore, it is also possible that cold water (with a temperature of 0.4°C; cf. Scapozza and Reynard, 2007) emerging from the active rock glacier of Piancabella feeds the Sceru RG spring through a subsurface flow along the southern slope of the valley, as directly proved by water tracing performed by Mari et al. (2013). Water temperature measured at the Leìt RG and Nero IP sources was rather constant and cold (<1.5°C) throughout the warm season, consistent with the water temperature of other active rock glacier outflows (Krainer and Mostler, 2002; Krainer et al., 2007; Geiger et al., 2014). This trend in water temperature could indicate that the flow was in contact with an ice body (Berger et al., 2004; Scapozza et al., 2020a). Vaux RG, Schenadüi RG and Pièi RG sources showed higher temperatures and daily variations throughout the monitoring period. At the Lac des Vaux site, the position of the temperature sensor (located about 225 m from the rock glacier front) probably played an important role in the observed thermal evolution. Indeed, the water emerging from the rock glacier was subjected to heat exchange with the atmosphere before reaching the sensor, which would explain the daily fluctuations observed. The very low water flow emerging from Alpe Pièi and Ganoni di Schenadüi rock glaciers (the water was almost stagnant) likely played an important role in the observed temperatures, by promoting heat exchange between water and atmosphere.
Similar isotopic values between water sources and snow samples in early summer indicate that all streams were fed by snowmelt during this period. Afterwards, an enrichment in 18O in all water sources (except in Sceru RG and Vaux non-RG sources) has been observed between early summer (June-July) and late summer (August). Between late summer and early autumn (October), δ18O values measured in RG sources tended to remain rather stable, with the exception of Pièi RG source, whose δ18O values significantly decreased. This seasonal evolution of the isotopic signature in water emerging from rock glaciers was clearly visible in Vaux RG source thanks to the high-resolution monitoring. A similar trend was observed by Krainer et al. (2007), who measured an increase in δ18O values from −17.5‰ (snowmelt) to about −14‰ (from August until early autumn). Harrington et al. (2018) also observed such a seasonal variation in isotopic signature in the water emerging from the Helen Creek rock glacier (Canada), although the transition from snowmelt (−20‰) to the plateau observed since August (−19/18.5‰) is less pronounced than that of Krainer et al. (2007) but more similar to that observed at the Vaux RG spring. Isotopic enrichment in water sources may indicate a change in the origin of water supply during the warm season. Already during the snowmelt, the isotopic content of the meltwater tends to increase due to isotopic fractionations taking place in the snowpack (cf. Moser and Stichler, 1974; Taylor et al., 2001). As suggested by Krainer et al. (2007), also water supply related to rain water can play a role in the 18O enrichment observed in the springs. Vaux RG source showed that the δ18O peaks recorded during the warm season occurred after rainfall events, indicating that there was an input of 18O-enriched water in the hydrological system.
Precipitation can have two effects on isotopic signature of water emerging from rock glaciers. In the short term, meteoric water rapidly released by rock glaciers causes δ18O peaks within hours of the rain event. In the long term, meteoric water that percolates through the ice/air and rock matrix can feed and isotopically enrich the subpermafrost flow circulating at the base of the rock glacier. But another type of water supply in active and inactive rock glaciers may contribute to seasonal enrichment in 18O, namely the water from ice melt (Krainer et al., 2007; Reato et al., 2022). As suggested by Williams et al. (2006), the increase in seasonal thaw depth during the warm season promotes the contact between liquid water and internal ice of rock glaciers, causing isotopic fractionations that concentrate heavier isotopes in the ice. In addition, several melt-freeze cycles can also enrich in δ18O the internal ice of rock glaciers (Steig et al., 1998; Williams et al., 2006). However, given the multitude of possible origins of the water emerging from rock glaciers, ice melt tracing remains a very difficult and complicated task (Krainer et al., 2007; Colombo et al., 2018a).
Water emerging from active rock glaciers was characterized by a progressive increase in ionic content (in particular SO42-, Ca2+ and Mg2+) during the warm season, consistent with the results of several studies (Williams et al., 2006; Thies et al., 2013; Colombo et al., 2018a; Scapozza et al., 2020a; Brighenti et al., 2021). Sceru RG spring was also characterized by a slight seasonal increase in SO42-, Ca2+ and Mg2+ values. In addition, SO42-, Ca2+, Mg2+ and NO3− concentrations measured in RG sources were globally greater than those detected in non-RG sources during the warm season. As explained before, the progressive increase in seasonal thaw depth probably affected the hydrochemistry of rock glacier outflows. In addition, it is possible to exclude significant solute export from watersheds caused by rainfall events, as the days preceding the sampling were generally characterized by dry conditions (except for water samples collected in early summer). Chemical elements such as sulfates, calcium and magnesium can therefore have a double origin. On the one hand, these ions can have a geological origin, through the chemical weathering of evaporite rocks (such as gypsum, anhydrite, calcite and dolomite; cf. Giovanoli et al., 1988; Spencer, 2000) and/or sulfide minerals (such as pyrrhotite and pyrite; cf. Scapozza et al., 2020a) in contact with air/water. In addition, chemical alteration by acidic water of silicate minerals (such as biotite and plagioclase) contained in gneissic rocks can release Ca2+ and Mg2+, as well as HCO3− (e.g., Giovanoli et al., 1988; Drever and Zobrist, 1992). On the other hand, these ions can also have an atmospheric origin. In fact, the cryosphere can store air pollutants and other chemical compounds derived from past atmospheric deposition, as evidenced by analyses of several ice cores collected in the Swiss Alps (e.g., Wagenbach and Geis, 1989; Sodemann et al., 2006; Bohleber et al., 2018). The last cold period favorable to significant ion storage in ice occurred probably between the 1960s and the 1980s (Scapozza et al., 2020a). Indeed, cold weather conditions (such as heavy snowfall and/or low summer temperatures) occurred during this period, as evidenced by the advancement of several glaciers in the European Alps (Wood, 1988). At the same time, a peak in anthropogenic sulphur dioxide (SO2) emissions and, consequently, in sulfur deposition occurred in Europe in the 1960s and 1970s (Mylona, 1996). Moreover, the Southern Alps is a region particularly affected by anthropogenic pollutant emissions (especially sulfur dioxide and nitrogen oxides) and deposition due to its proximity to the industrialized area of Po Plain (Steingruber, 2015; Rogora et al., 2016). Regarding base cation deposition, peaks of these ions in rain water measured in Southern Alps are often associated with alkaline precipitation that occurred in presence of dust (mostly rich in calcium and bicarbonate) in the atmosphere, which is usually of Saharan origin (Rogora et al., 2004; Rogora et al., 2016). Mineral dust concentration measured in ice cores collected from Colle Gnifetti allowed reconstruction of mineral dust events during the 20th century, showing that several depositions took place during the 1970s and 1980s (Wagenbach and Geis, 1989). Globally, the watersheds in this study are characterized by crystalline rocks such as orthogneiss and paragneiss—which are rather resistant to chemical and mechanical weathering (e.g., Kühni and Pfiffner, 2001)—and by almost total absence of evaporitic rocks and sulfide minerals (cf. Chapter 2.2). This lithological conformation suggests that ion release into the water emerging from rock glaciers is probably largely caused by perennial ground ice melting and only a small part by chemical weathering. However, at the Lac des Vaux site, the possible presence of carbonate-rich quartz schists belonging to the Col de Chassoure Formation (Sartori et al., 2006), could have affected the chemical composition of both RG and non-RG springs. In fact, both springs showed high concentrations of calcium, magnesium and inorganic carbon probably released by carbonate dissolution. Nevertheless, the seasonal increase in ion concentrations visible at the Vaux RG source would prove that the hydrochemistry of this spring was affected by ground thawing. In contrast, the less clear seasonal evolution of the ion content in the Pièi RG spring, as well as the similar concentrations measured in RG and non-RG sources, indicate the low probability that the rock glacier outflow was affected by perennial ground ice melting. High values of Ca2+ and TIC measured in both RG and non-RG sources could be caused by the dissolution of carbonate nodules contained in the Gana Bianca orthogneiss.
Nitrates contained in rock glacier outflows probably have a double origin, which would explain the absence of a clear seasonal trend of NO3− in most RG sources. On the one hand, microbial activity within rock glaciers can play an important role in nitrate release into the water, as suggested by some studies in the United States (Williams et al., 2007; Barnes et al., 2014; Fegel et al., 2016). Colombo et al. (2018a) and Colombo et al. (2019) also measured elevated NO3− values in Alpine ponds located in catchments with probable permafrost occurrence in the north-western Italian Alps (Aosta Valley). These studies suggest that NO3− enrichment was probably due to water flowing through sediments where microbial activities were active. On the other hand, as suggested by Scapozza et al. (2020a), the release of atmospheric pollutants through ice melting can also enrich the water in NO3−. The highest values of nitrates were measured at the Pièi RG (2.9 mg/L) and Sceru RG (1.5 mg/L) sources. It is possible to consider that the inactive rock glacier of Alpe Pièi and the relict rock glacier of Alpe di Sceru I may potentially represent porous environments suitable for the development of microbial communities. In contrast, all non-RG sources were characterized by low NO3− values. This is probably due to the important presence of rock outcrops upstream of these sampling points at most sites, which represent less suitable environments for microbial community development.
The spatial comparison between sources and sites of different physico-chemical parameters measured in the water, provided insight into whether and where there may have been perennial ground ice melting during the warm season and what tracers might indicate such process (Table 6). Perennial ground ice melting thus probably affects the physico-chemical composition (especially EC, SO42-, Ca2+ and Mg2+) of the water emerging from the active rock glaciers investigated. In contrast, a different situation was observed at sites with relict and inactive rock glaciers. The slight seasonal increase in ionic content (SO42-, Ca2+ and Mg2+) observed in the water emerging from the relict rock glacier could trace the perennial ground ice melting occurred at the top of the valley, although this water supply may have been partially masked by water mixing process in an internal aquifer. In contrast, no clear signal of perennial ground ice melting was observed in the outflow of the inactive rock glacier.
TABLE 6. Summary of the potential ice melt tracers resulting from the spatial comparison between sources and sites.
Figure 9 shows a conceptual model of the main hydro-chemical processes taking place within an active rock glacier in the current context of atmospheric warming, which causes both seasonal and perennial ground ice melt. This model is based on the results of this study and on the conceptual models suggested by Williams et al. (2006) and Colombo et al. (2018a). In the past, atmospheric depositions of eolian dust and anthropogenic pollutants that occurred during the winter period, allowed the temporary accumulation of various chemical compounds in the snowpack (Figure 9A). In early summer of past and present times, ice aggradation can occur at the base of the active layer through the refreezing of the meltwater coming from the snowpack (e.g., Hanson and Hoezle, 2004). The chemical compounds that were stored in the snowpack are then transferred into the ground ice. The rock glacier outflow is mainly fed by meltwater coming from the snowpack, which is poorly conductive and depleted in 18O and in ions, consistent with the model suggested by Colombo et al. (2018a). The suprapermafrost flow is dominant and flows rapidly over a largely impermeable surface formed by the seasonal ground ice. Highest discharges and reduced water circulation within the rock glacier are observed in this period (Figure 9B). In late summer of the present time, the snow cover has almost completely disappeared, allowing heat propagation into the ground. Seasonal ground ice melt occurs and voids can open. For this reason, intrapermafrost flow is greater than that observed in early summer, when the basis of the active layer was still frozen. The strong reduction of 18O-depleted and poorly mineralized water coming from snowmelt and the increase in groundwater (mainly fed by rainwater) would explain the increase in electrical conductivity and in ionic/isotopic content in the rock glacier outflow compared to early summer (Figure 9C). In early autumn of the present time, the active layer no longer contains seasonal ground ice and melting of the perennial ground ice at the permafrost table may occur. Chemical compounds that were stored into the perennial ground ice are then released and flushed out of the system by water circulating within the rock glacier. In fact, the water emerging from the rock glacier shows high electrical conductivity values and high ion content (especially SO42-, Ca2+ and Mg2+) (Figure 9D). At this stage, water percolation is quite widespread within the rock glacier, as indicated by low water flow velocities. However, the water availability is generally low during this period, as evidenced by the low discharge of the rock glacier outflow. Perennial ground ice located at the permafrost table can be subjected to δ18O enrichment due to isotopic fractionations between liquid water and ice. The isotopic values of the water emerging from the rock glacier are high and quite similar to those measured in late summer, which may indicate that the outflow is a mixture of groundwater (which is mainly fed by rainy water) and meltwater related to the perennial ground ice melting, as suggested by Krainer et al. (2007).
FIGURE 9. Conceptual model of the main hydro-chemical processes taking place in an active rock glacier, divided into four time phases: (A) winter time in the past, (B) early summer in past and present time, (C) late summer in present time and (D) early autumn in present time. This figure refers to the conceptual models proposed by Williams et al. (2006) and Colombo et al. (2018a).
In the global context of permafrost degradation as a consequence of the ongoing climate change, hydrological studies of rock glaciers are essential to understand and evaluate their potential as a water resource in high mountain regions. This research aimed at improving knowledge about ice melt tracing in rock glacier outflows through physical and chemical parameters, in order to understand the hydrological role played by these high altitude debris accumulations in periglacial watersheds. A spatial comparison between sites and springs allowed to observe that sources fed by active rock glaciers were characterized by a seasonal increase in electrical conductivity and in ionic content (especially SO42-, Ca2+ and Mg2+). In addition, RG springs were globally characterized by higher ion values (especially SO42-, Ca2+, Mg2+ and NO3−) than those measured in non-RG sources. The only exception is the site with the inactive rock glacier, where the similar chemical-isotopic composition between RG and non-RG springs could indicate that the perennial ground ice is now located at greater depth. We assume that physico-chemical parameters (especially EC, SO42-, Ca2+ and Mg2+) measured in water emerging from active rock glaciers represent potential ice melt tracers. Gneissic lithology of watersheds suggests that the ion release observed in these springs is mainly caused by perennial ground ice melting and only a small part by chemical weathering of the rocks. It is hypothesized that the cryosphere stored a large amount of chemical compounds arising from atmospheric fallout during a colder period in the past (e.g., 1960s–1980s) and that the current melting of the perennial ground ice partially releases these chemical compounds in the Alpine water ecosystems. The ion release process related to perennial ground ice melting is expected to decrease in future as the ice content of rock glaciers is also expected to decrease due to atmospheric warming. However, in the coming years it will be important to continue this kind of monitoring in order to quantify the impact of perennial ground ice melting on hydrochemistry of Alpine water ecosystems. It would also be interesting to combine hydro-chemical and geophysical/geodetic measurements in order to quantify the changes in rock glacier ice content.
The datasets presented in this study can be found in online repositories. The names of the repository/repositories and accession number(s) can be found in the article/supplementary material.
CD prepared the manuscript with contributions from all co-authors.
We would like to thank the Fondation Agassiz for funding the laboratory analyses. Thank you also to Lia Franchini, Daphné Giacomazzi, Aurélien Ballu and Jérémie Gentizon for their contribution to fieldwork, to Torsten Vennemann, Laetitia Monbaron and Micaela Faria for aiding in the laboratory analyses and to Daniel Hunkeler for his contribution to the study of the hydrology of the Lac des Vaux rock glacier. Our thanks go to MeteoSwiss and Swiss Federal Institute for Snow and Avalanche Research for the data they provided. Finally, we acknowledge the reviewers JB and CV, as well as the editor SB, for their valuable suggestions and useful comments that greatly improved the paper. This manuscript summarizes the contents of the Master’s thesis of Del Siro. (2021).
The authors declare that the research was conducted in the absence of any commercial or financial relationships that could be construed as a potential conflict of interest.
All claims expressed in this article are solely those of the authors and do not necessarily represent those of their affiliated organizations, or those of the publisher, the editors and the reviewers. Any product that may be evaluated in this article, or claim that may be made by its manufacturer, is not guaranteed or endorsed by the publisher.
Barnes, R. T., Williams, M. W., Parman, J. N., Hill, K., and Caine, N. (2014). Thawing glacial and permafrost features contribute to nitrogen export from Green Lakes Valley, Colorado Front Range, USA. Biogeochemistry 117 (2-3), 413–430. doi:10.1007/s10533-013-9886-5
Beniston, M., and Stoffel, M. (2014). Assessing the impacts of climatic change on mountain water resources. Sci. Total Environ. 493, 1129–1137. doi:10.1016/j.scitotenv.2013.11.122
Berger, J., Krainer, K., and Mostler, W. (2004). Dynamics of an active rock glacier (Ötztal Alps, Austria). Quat. Res. 62 (3), 233–242. doi:10.1016/j.yqres.2004.07.002
Bianconi, F., and Strasky, S. (2015). “138 ambrì-piotta (CN 1252),” in geological atlas of Switzerland (Bern-Wabern: Federal Office of Topography swisstopo).
Biskaborn, B. K., Smith, S. L., Noetzli, J., Matthes, H., Vieira, G., Streletskiy, D. A., et al. (2019). Permafrost is warming at a global scale. Nat. Commun. 10 (1), 264–311. doi:10.1038/s41467-018-08240-4
Bohleber, P., Erhardt, T., Spaulding, N., Hoffmann, H., Fischer, H., and Mayewski, P. (2018). Temperature and mineral dust variability recorded in two low-accumulation Alpine ice cores over the last millennium. Clim. Past 14 (1), 21–37. doi:10.5194/cp-14-21-2018
Brighenti, S., Engel, M., Tolotti, M., Bruno, M. C., Wharton, G., Comiti, F., et al. (2021). Contrasting physical and chemical conditions of two rock glacier springs. Hydrol. Process. 35 (4), e159. doi:10.1002/hyp.14159
Brighenti, S., Tolotti, M., Bruno, M. C., Engel, M., Wharton, G., Cerasino, L., et al. (2019). After the peak water: The increasing influence of rock glaciers on alpine river systems. Hydrol. Process. 33 (21), 2804–2823. doi:10.1002/hyp.13533
Buchli, T., Merz, K., Zhou, X., Kinzelbach, W., and Springman, S. M. (2013). Characterization and monitoring of the furggwanghorn rock glacier, Turtmann Valley, Switzerland: Results from 2010 to 2012. Vadose Zone J. 12 (1), vzj20120067–15. doi:10.2136/vzj2012.0067
Carturan, L., Zuecco, G., Seppi, R., Zanoner, T., Borga, M., Carton, A., et al. (2016). Catchment-Scale permafrost mapping using spring water characteristics. Permafr. Periglac. Process. 27 (3), 253–270. doi:10.1002/ppp.1875
Clark, I., and Fritz, I. (1997). Environmental isotopes in hydrogeology. Boca Raton: Lewis Publishers.
Colombo, N., Gruber, S., Martin, M., Malandrino, M., Magnani, A., Godone, D., et al. (2018a). Rainfall as primary driver of discharge and solute export from rock glaciers: The Col d'Olen Rock Glacier in the NW Italian Alps. Sci. Total Environ. 639, 316–330. doi:10.1016/j.scitotenv.2018.05.098
Colombo, N., Salerno, F., Gruber, S., Freppaz, M., Williams, M., Fratianni, S., et al. (2018b). Review: Impacts of permafrost degradation on inorganic chemistry of surface fresh water. Glob. Planet. Change 162, 69–83. doi:10.1016/j.gloplacha.2017.11.017
Colombo, N., Salerno, F., Martin, M., Malandrino, M., Giardino, M., Serra, E., et al. (2019). Influence of permafrost, rock and ice glaciers on chemistry of high-elevation ponds (NW Italian Alps). Sci. Total Environ. 685, 886–901. doi:10.1016/j.scitotenv.2019.06.233
Colombo, N., Sambuelli, L., Comina, C., Colombero, C., Giardino, M., Gruber, S., et al. (2018c). Mechanisms linking active rock glaciers and impounded surface water formation in high-mountain areas. Earth Surf. Process. Landforms 43 (2), 417–431. doi:10.1002/esp.4257
Del Siro, C. (2021). “Origine et qualité de l’eau émergeant des glaciers rocheux. Études de cas dans les Alpes suisses,” in Mémoire de Master (Suisse: Université de Lausanne, Faculté des géosciences et de l’environnement). Available at: https://igd.unil.ch/memoires/memoires/1884 (Accessed September 5, 2022).
Deluigi, N., Lambiel, C., and Kanevski, M. (2017). Data-driven mapping of the potential mountain permafrost distribution. Sci. Total Environ. 590, 370–380. doi:10.1016/j.scitotenv.2017.02.041
Deluigi, N., and Scapozza, C. (2020). Il permafrost nelle Alpi Ticinesi: Ripartizione potenziale attuale e futura. Boll. della Soc. ticinese Sci. Nat. 108, 15–27. Available at: http://repository.supsi.ch/id/eprint/12075.
Drever, J. I., and Zobrist, J. (1992). Chemical weathering of silicate rocks as a function of elevation in the southern Swiss Alps. Geochimica Cosmochimica Acta 56 (8), 3209–3216. doi:10.1016/0016-7037(92)90298-W
Duguay, M. A., Edmunds, A., Arenson, L. U., and Wainstein, P. A. (2015). “Quantifying the significance of the hydrological contribution of a rock glacier – a review,” in GEOQuébec 2015 – 68th Canadian geotechnical conference and 7th Canadian permafrost conference (Quebec, Canada: GEOQuébec). Available at: https://www.researchgate.net/publication/282402787_Quantifying_the_significance_of_the_hydrological_contribution_of_a_rock_glacier_-_A_review (Accessed March 1, 2022).
Fegel, T. S., Baron, J. S., Fountain, A. G., Johnson, G. F., and Hall, E. K. (2016). The differing biogeochemical and microbial signatures of glaciers and rock glaciers. J. Geophys. Res. Biogeosciences 121 (3), 919–932. doi:10.1002/2015JG003236
Franchini, L. (2022). Étude de l’hydrologie d’un glacier rocheux. Le cas du Lac des Vaux, Mémoire de Master (Suisse: Université de Lausanne, Faculté des géosciences et de l’environnement).
Geiger, S. T., Daniels, J. M., Miller, S. N., and Nicholas, J. W. (2014). Influence of rock glaciers on stream hydrology in the La Sal Mountains, Utah. Arct. Antarct. Alp. Res. 46 (3), 645–658. doi:10.1657/1938-4246-46.3.645
Giardino, R., Regmi, N., and Vitek, J. (2011). “Rock glaciers,” in Encyclopedia of snow, ice and glaciers. Editors V. P. Singh, P. Singh, and U. K. Haritashya (Dordrecht: Springer Netherlands), 943–948. doi:10.1007/978-90-481-2642-2_453
Giovanoli, R., Schnoor, J. L., Sigg, L., Stumm, W., and Zobrist, J. (1988). Chemical weathering of crystalline rocks in the catchment area of acidic Ticino lakes, Switzerland. Clays Clay Minerals 36 (6), 521–529. doi:10.1346/CCMN.1988.0360605
Haeberli, W., and Beniston, M. (1998). Climate change and its impacts on glaciers and permafrost in the Alps. Ambio 27 (4), 258–265. Available at: http://www.jstor.org/stable/4314732.
Haeberli, W., Schaub, Y., and Huggel, C. (2017). Increasing risks related to landslides from degrading permafrost into new lakes in de-glaciating mountain ranges. Geomorphology 293, 405–417. doi:10.1016/j.geomorph.2016.02.009
Haeberli, W. (1975). Untersuchungen zur Verbreitung von Permafrost zwischen Flüelapass und Piz Grialetsch (Graubünden). Mittl. Des. VAW – ETH Zürich 17, 1–221. Available at: https://ethz.ch/content/dam/ethz/special-interest/baug/vaw/vaw-dam/documents/das-institut/mitteilungen/1970-1979/017.pdf (Accessed March 1, 2022).
Hanson, S., and Hoelzle, M. (2004). The thermal regime of the active layer at the Murtèl rock glacier based on data from 2002. Permafr. Periglac. Process. 15 (3), 273–282. doi:10.1002/ppp.499
Harrington, J. S., Mozil, A., Hayashi, M., and Bentley, L. R. (2018). Groundwater flow and storage processes in an inactive rock glacier. Hydrol. Process. 32 (20), 3070–3088. doi:10.1002/hyp.13248
Ilyashuk, B. P., Ilyashuk, E. A., Psenner, R., Tessadri, R., and Koinig, K. A. (2014). Rock glacier outflows may adversely affect lakes: Lessons from the past and present of two neighboring water bodies in a crystalline-rock watershed. Environ. Sci. Technol. 48 (11), 6192–6200. doi:10.1021/es500180c
Ilyashuk, B. P., Ilyashuk, E. A., Psenner, R., Tessadri, R., and Koinig, K. A. (2018). Rock glaciers in crystalline catchments: Hidden permafrost-related threats to alpine headwater lakes. Glob. Change Biol. 24 (4), 1548–1562. doi:10.1111/gcb.13985
Jones, D. B., Harrison, S., Anderson, K., Selley, H. L., Wood, J. L., and Betts, R. A. (2018). The distribution and hydrological significance of rock glaciers in the Nepalese Himalaya. Glob. Planet. Change 160, 123–142. doi:10.1016/j.gloplacha.2017.11.005
Jones, D. B., Harrison, S., Anderson, K., and Whalley, W. B. (2019). Rock glaciers and mountain hydrology: A review. Earth-Science Rev. 19, 66–90. doi:10.1016/j.earscirev.2019.04.001
Krainer, K., Bressan, D., Dietre, B., Haas, J. N., Hajdas, I., Lang, K., et al. (2015). A 10,300-year-old permafrost core from the active rock glacier Lazaun, southern Ötztal Alps (South Tyrol, northern Italy). Quat. Res. 83 (2), 324–335. doi:10.1016/j.yqres.2014.12.005
Krainer, K., and Mostler, W. (2002). Hydrology of active rock glaciers: Examples from the Austrian Alps. Arct. Antarct. Alp. Res. 34 (2), 142–149. doi:10.1080/15230430.2002.12003478
Krainer, K., Mostler, W., and Spötl, C. (2007). Discharge from active rock glaciers, Austrian Alps: A stable isotope approach. Austrian J. Earth Sci. 100, 102–112.
Kühni, A., and Pfiffner, O. A. (2001). The relief of the Swiss Alps and adjacent areas and its relation to lithology and structure: Topographic analysis from a 250-m DEM. Geomorphology 41 (4), 285–307. doi:10.1016/S0169-555X(01)00060-5
Lambiel, C. (2006). Le pergélisol dans les terrains sédimentaires à forte déclivité: Distribution, régime thermique et instabilités. Thèse de doctorat. Suisse: Université de Lausanne, Faculté des géosciences et de l’environnement. Available at: https://doc.rero.ch/record/6234/files/These_LambielC.pdf (Accessed March 1, 2022).
Litaor, M. I. (2022). Alpine ecosystem response to climate warming: Long-term monitoring data of stream chemistries revisited. Sci. Total Environ. 839 (156292), 1–14. doi:10.1016/j.scitotenv.2022.156292
Mari, S., Scapozza, C., Pera Ibarguren, S., and Delaloye, R. (2013). Prove di multitracciamento di ghiacciai rocciosi e ambienti periglaciali nel Vallon de Réchy (VS) e nella Valle di Sceru (TI). Boll. della Soc. ticinese Sci. Nat. 101, 13–20. Available at: http://repository.supsi.ch/id/eprint/3625.
Marmy, A., Rajczak, J., Delaloye, R., Hilbich, C., Hoelzle, M., Kotlarski, S., et al. (2016). Semi-automated calibration method for modelling of mountain permafrost evolution in Switzerland. Cryosphere 10 (6), 2693–2719. doi:10.5194/tc-10-2693-2016
Messerli, B., Viviroli, D., and Weingartner, R. (2004). Mountains of the world: Vulnerable water towers for the 21st century. Ambio, 29–34. Available at: http://www.jstor.org/stable/25094585.
Mollaret, C., Hilbich, C., Pellet, C., Flores-Orozco, A., Delaloye, R., and Hauck, C. (2019). Mountain permafrost degradation documented through a network of permanent electrical resistivity tomography sites. Cryosphere 13 (10), 2557–2578. doi:10.5194/tc-13-2557-2019
Moser, H., and Stichler, W. (1974). Deuterium and oxygen-18 contents as an index of the properties of snow covers. Int. Assoc. Hydrological Sci. Publ. 114, 122–135.
Mylona, S. (1996). Sulphur dioxide emissions in Europe 1880–1991 and their effect on sulphur concentrations and depositions. Tellus B 48 (5), 662–689. doi:10.1034/j.1600-0889.1996.t01-2-00005.x
PERMOS (2019). “Permafrost in Switzerland 2014/2015 to 2017/2018,” in Glaciological Report (Permafrost) No. 16-19 of the Cryospheric Commission of the Swiss Academy of Sciences. Editors J. Noetzli, C. Pellet, and B. Staub (Bern: Swiss Academy of Sciences). doi:10.13093/permos-rep-2019-16-19
PERMOS (2022). Swiss permafrost bulletin 2021. Editors J. Noetzli, and C. Pellet 3, 1–22. doi:10.13093/permos-bull-2022
R Core Team (2022). R: A language and environment for statistical computing. Vienna, Austria: R Foundation for Statistical Computing. Available at: https://www.R-project.org/.
Reato, A., Borzi, G., Martínez, O. A., and Carol, E. (2022). Role of rock glaciers and other high-altitude depositional units in the hydrology of the mountain watersheds of the Northern Patagonian Andes. Sci. Total Environ. 824, 153968. doi:10.1016/j.scitotenv.2022.153968
Rogora, M., Colombo, L., Marchetto, A., Mosello, R., and Steingruber, S. (2016). Temporal and spatial patterns in the chemistry of wet deposition in Southern Alps. Atmos. Environ. 146, 44–54. doi:10.1016/j.atmosenv.2016.06.025
Rogora, M., Mosello, R., and Marchetto, A. (2004). Long-term trends in the chemistry of atmospheric deposition in northwestern Italy: The role of increasing saharan dust deposition. Tellus B Chem. Phys. Meteorology 56 (5), 426–434. doi:10.3402/tellusb.v56i5.16456
Sartori, M., Gouffon, Y., and Marthaler, M. (2006). Harmonisation et définition des unités lithostratigraphiques briançonnaises dans les nappes penniques du Valais. Eclogae Geol. Helvetiae 99 (3), 363–407. doi:10.1007/s00015-006-1200-2
Scapozza, C. (2008). Contribution à l’étude géomorphologique et géophysique des environnements périglaciaires des Alpes Tessinoises orientales. Mémoire de Master. Suisse: Université de Lausanne, Faculté des géosciences et de l’environnement. Available at: http://doc.rero.ch/record/8799 (Accessed March 1, 2022).
Scapozza, C. (2009). Contributo dei metodi termici alla prospezione del permafrost montano: Esempi dal massiccio della Cima di Gana Bianca (Val Blenio, Svizzera). Boll. della Soc. ticinese Sci. Nat. 97, 55–66. Available at: http://repository.supsi.ch/id/eprint/3145.
Scapozza, C., Deluigi, N., Bulgheroni, M., Pera Ibarguren, S., Pozzoni, M., Colombo, L., et al. (2020a). Assessing the impact of ground ice degradation on high mountain lake environments (Lago Nero catchment, Swiss Alps). Aquat. Sci. 82 (1), 5. doi:10.1007/s00027-019-0675-7
Scapozza, C., Deluigi, N., Del Siro, C., Pollo, A., and Antognini, M. (2020b). Il permafrost nelle Alpi Ticinesi (2017/2018 e 2018/2019). Rapporto No. 5 del Gruppo Permafrost Ticino. Boll. Della Soc. ticinese Sci. Nat. 108, 13–23. Available at: http://repository.supsi.ch/id/eprint/12077.
Scapozza, C., Gex, P., Lambiel, C., and Reynard, E. (2008). “Contribution of self-potential (SP) measurements in the study of alpine periglacial landforms: Examples from the southern Swiss Alps,” in Proceedings of the 9th international conference on permafrost, Fairbanks, Alaska. Editors D. L. Kane, and K. M. Hinkel (Fairbanks, Alaska, USA: Institute of Northern Engineering, University of Alaska Fairbanks), 1583–1588. Available at: http://repository.supsi.ch/id/eprint/3142.
Scapozza, C., Giaccone, E., Mari, S., Antognini, M., Fratianni, S., and Ambrosi, C. (2016). Il permafrost nelle Alpi Ticinesi (2013/2014 e 2014/2015). Rapporto No. 3 del Gruppo Permafrost Ticino. Boll. Della Soc. ticinese Sci. Nat. 104, 37–44. Available at: http://repository.supsi.ch/id/eprint/7891.
Scapozza, C., Giaccone, E., Mari, S., Antognini, M., Fratianni, S., and Ambrosi, C. (2015). Il permafrost nelle Alpi Ticinesi: Temperatura e movimenti dei ghiacciai rocciosi dal 2006 al 2014. Dati – Stat. Soc. 2, 100–109. Available at: http://repository.supsi.ch/id/eprint/6809.
Scapozza, C., Lambiel, C., Gex, P., and Reynard, E. (2011). Prospection géophysique multi-méthodes du pergélisol alpin dans le Sud des Alpes Suisses. Géormophologie relief, Process. Environ. 17 (1), 15–32. doi:10.4000/geomorphologie.8765
Scapozza, C., Mari, S., Antognini, M., Lepori, V., and Ambrosi, C. (2014). Il permafrost nelle Alpi Ticinesi (2011/2012 e 2012/2013). Rapporto No. 2 del Gruppo Permafrost Ticino. Boll. Della Soc. ticinese Sci. Nat. 102, 59–69. Available at: http://repository.supsi.ch/id/eprint/5420.
Scapozza, C., and Reynard, E. (2007). Rock glaciers e limite inferiore del permafrost discontinuo tra la cima di Gana Bianca e la Cima di Piancabella (Val Blenio, TI). Geol. Insubrica 10, 29–40.
Scapozza, C. (2013). Stratigraphie, morphodynamique, paléoenvironnements des terrains sédimentaires meubles à forte déclivité du domaine périglaciaire alpin (Géovisions n°40). Lausanne: Université, Institut de géographie et durabilité. Available at: https://igd.unil.ch/www/geovisions/40/Geovisions_40.pdf (Accessed March 1, 2022).
Seidel, K., Ehrler, C., and Martinec, J. (1998). Effects of climate change on water resources and runoff in an Alpine basin. Hydrol. Process. 12 (10-11), 1659–1669. doi:10.1002/(SICI)1099-1085(199808/09)12:10/11<1659:AID-HYP687>3.0.CO;2-4
Sodemann, H., Palmer, A. S., Schwierz, C., Schwikowski, M., and Wernli, H. (2006). The transport history of two Saharan dust events archived in an Alpine ice core. Atmos. Chem. Phys. 6 (3), 667–688. doi:10.5194/acp-6-667-2006
Spencer, R. J. (2000). Sulfate minerals in evaporite deposits. Rev. Mineralogy Geochem. 40 (1), 173–192. doi:10.2138/rmg.2000.40.3
Steig, E. J., Fitzpatrick, J. J., Potter, N., and Clark, D. H. (1998). The geochemical record in rock glaciers. Geogr. Ann. Ser. A, Phys. Geogr. 80 (3-4), 277–286. doi:10.1111/j.0435-3676.1998.00043.x
Steingruber, S. M., Bernasconi, S. M., and Valenti, G. (2020). Climate change-induced changes in the chemistry of a high-altitude mountain lake in the central Alps. Aquat. Geochem. 27, 1–22. doi:10.1007/s10498-020-09388-6
Steingruber, S. M. (2015). Deposition of acidifying and eutrophying pollutants in southern Switzerland from 1988 to 2013. Boll. della Soc. ticinese Sci. Nat. 103, 37–45. Available at: https://www.researchgate.net/publication/287201113_Deposition_of_Acidifying_and_Eutrophying_Pollutants_in_Southern_Switzerland_from_1988_to_2013 (Accessed March 1, 2022).
Swisstopo (2020). 1326 rosablanche (LK 1326), geological vector datasets GeoCover 1:25’000. Bern-Wabern: Federal Office of Topography swisstopo.
Taylor, S., Feng, X., Kirchner, J. W., Osterhuber, R., Klaue, B., and Renshaw, C. E. (2001). Isotopic evolution of a seasonal snowpack and its melt. Water Resour. Res. 37 (3), 759–769. doi:10.1029/2000WR900341
Thies, H., Nickus, U., Mair, V., Tessadri, R., Tait, D., Thaler, B., et al. (2007). Unexpected response of high alpine lake waters to climate warming. Environ. Sci. Technol. 41 (21), 7424–7429. doi:10.1021/es0708060
Thies, H., Nickus, U., Tolotti, M., Tessadri, R., and Krainer, K. (2013). Evidence of rock glacier melt impacts on water chemistry and diatoms in high mountain streams. Cold Regions Sci. Technol. 96, 77–85. doi:10.1016/j.coldregions.2013.06.006
Viviroli, D., Dürr, H. H., Messerli, B., Meybeck, M., and Weingartner, R. (2007). Mountains of the world, water towers for humanity: Typology, mapping, and global significance. Water Resour. Res. 43 (7), 5653. doi:10.1029/2006WR005653
Vonder Mühll, D. (1992). Evidence of intrapermafrost groundwater flow beneath an active rock glacier in the Swiss Alps. Permafr. Periglac. Process. 3, 169–173. doi:10.1002/ppp.3430030216
Wagenbach, D., and Geis, K. (1989). “The mineral dust record in a high altitude alpine glacier (Colle Gnifetti, Swiss Alps),” in Paleoclimatology and paleometeorology: Modern and past patterns of global atmospheric transport. Editors M. Leinen, and M. Sarnthein (Dordrecht: Springer), 543–564. doi:10.1007/978-94-009-0995-3_23
Wagner, T., Kainz, S., Helfricht, K., Fischer, A., Avian, M., Krainer, K., et al. (2021a). Assessment of liquid and solid water storage in rock glaciers versus glacier ice in the Austrian Alps. Sci. Total Environ. 800, 149593. doi:10.1016/j.scitotenv.2021.149593
Wagner, T., Kainz, S., Krainer, K., and Winkler, G. (2021b). Storage-discharge characteristics of an active rock glacier catchment in the Innere Ölgrube, Austrian Alps. Hydrol. Process. 35 (5), e14210. doi:10.1002/hyp.14210
Wagner, T., Pauritsch, M., and Winkler, G. (2016). Impact of relict rock glaciers on spring and stream flow of alpine watersheds: Examples of the Niedere Tauern Range, Eastern Alps (Austria). Austrian J. Earth Sci. 109 (1), 6. doi:10.17738/ajes.2016.0006
Williams, M. W., Knauf, M., Caine, N., Liu, F., and Verplanck, P. L. (2006). Geochemistry and source waters of rock glacier outflow, Colorado Front Range. Permafr. Periglac. Process. 17 (1), 13–33. doi:10.1002/ppp.535
Williams, M. W., Knauf, M., Cory, R., Caine, N., and Liu, F. (2007). Nitrate content and potential microbial signature of rock glacier outflow, Colorado Front Range. Earth Surf. Process. Landforms J. Br. Geomorphol. Res. Group 32 (7), 1032–1047. doi:10.1002/esp.1455
Winkler, G., Wagner, T., Krainer, K., Ribis, M., and Hergarten, S. (2018). “Hydrogeology of rock glaciers—storage capacity and drainage dynamics—an overview,” in Novel methods and results of landscape research in Europe, central asia and siberia. Editors V. G. Sychev, and L. Mueller (Moscow: FGBNU “VNII agrochimii”). Available at: https://www.researchgate.net/publication/336369223_Hydrogeology_of_rock_glaciers_-_storage_capacity_and_drainage_dynamics_-_an_overview (Accessed March 1, 2022).
Winkler, G., Wagner, T., Pauritsch, M., Birk, S., Kellerer-Pirklbauer, A., Benischke, R., et al. (2016). Identification and assessment of groundwater flow and storage components of the relict schöneben rock glacier, niedere tauern range, eastern Alps (Austria). Hydrogeology J. 24 (4), 937–953. doi:10.1007/s10040-015-1348-9
Keywords: climate change, alpine cryosphere, rock glaciers, water resources, perennial ground ice, water chemistry
Citation: Del Siro C, Scapozza C, Perga M-E and Lambiel C (2023) Investigating the origin of solutes in rock glacier springs in the Swiss Alps: A conceptual model. Front. Earth Sci. 11:1056305. doi: 10.3389/feart.2023.1056305
Received: 28 September 2022; Accepted: 18 January 2023;
Published: 09 February 2023.
Edited by:
Stefano Brighenti, Free University of Bozen-Bolzano, ItalyReviewed by:
Jan Blöthe, University of Freiburg, GermanyCopyright © 2023 Del Siro, Scapozza, Perga and Lambiel. This is an open-access article distributed under the terms of the Creative Commons Attribution License (CC BY). The use, distribution or reproduction in other forums is permitted, provided the original author(s) and the copyright owner(s) are credited and that the original publication in this journal is cited, in accordance with accepted academic practice. No use, distribution or reproduction is permitted which does not comply with these terms.
*Correspondence: Chantal Del Siro, Y2hhbnRhbC5kZWxzaXJvQHN1cHNpLmNo
Disclaimer: All claims expressed in this article are solely those of the authors and do not necessarily represent those of their affiliated organizations, or those of the publisher, the editors and the reviewers. Any product that may be evaluated in this article or claim that may be made by its manufacturer is not guaranteed or endorsed by the publisher.
Research integrity at Frontiers
Learn more about the work of our research integrity team to safeguard the quality of each article we publish.