- 1State Key Laboratory of Oil and Gas Reservoir Geology and Exploitation, Southwest Petroleum University, Chengdu, China
- 2State Key Laboratory of Natural Gas Hydrate, Beijing, China
- 3State Key Laboratory of Coal Mine Disaster Dynamics and Control, Chongqing University, Chongqing, China
Securing energy means grasping the key link in the national development and security strategy. Under the goals of carbon peak and carbon neutrality, the overall tendency of energy development is to increase the proportion of natural gas while stabilizing oil consumption, and the global primary energy is entering the era of natural gas. Gas hydrate in deep seabed shallow strata and extremely cold permafrost regions has piqued the interest of researchers due to its abundant resources, widespread distribution, and high energy density. Although the drilling of hydrate wells is still fraught with unknowns and challenges due to the technological barriers between countries, complex on-site working conditions, and unique physical chemical properties, accumulation forms, and occurrence characteristics of gas hydrate, more than ten successful trial productions around the world have opened the door of hope for the development of this potentially new energy. The gas hydrate reservoir drilling technique is the frontier and hotspot of scientific and technological innovation and competitiveness around the globe today, reflecting the level of oil and gas technical advancement. At the national level, it possesses strategic and revolutionary features. Innovative drilling techniques, scientific well location layout, appropriate wellbore structure and well trajectory design, efficient drilling fluid, qualified drilling and completion equipment, and successful pressure-temperature preserved coring may all provide a strong guarantee for the successful completion of gas hydrate wells. This review comprehensively reviews the drilling techniques and engineering measures that can be used to develop gas hydrate. It focuses on the research advancement of important hydrate drilling technologies and the enlightening significance of these developments in the application of hydrate drilling. This work will deliver valuable experience as well as comprehensive scientific information for gas hydrate exploration and drilling.
Introduction
The primary issue of energy development has always been how energy-consuming countries efficiently secure national energy security as well as national economic and social development. Exploring alternative energy is critical for the demand for clean energy at present due to the depletion of traditional energy and the increase in energy demand (Li et al., 2016a; Thakur, 2010). However, alternative energy sources such as electricity, nuclear energy, and geothermal energy are insufficient to supply the world’s fast-increasing energy demand. Accordingly, fossil fuels such as oil and natural gas are expected to remain the fundamental energy sources in the future. Deep oil and gas, offshore oil and gas, unconventional oil and gas, low-grade oil and gas, and enhanced oil and gas recovery of developed fields are the key areas of oil and gas resource exploration and development in the future (Heidari et al., 2022; Li et al., 2020a; Isaac et al., 2022). Natural gas, as the fastest-growing fossil fuel in the twenty-first century, will not only help us achieve a low-carbon future but will also serve as a link between today’s primary fossil fuels and future renewable energy (Sahu et al., 2020). According to the BP Statistical Review of World Energy 2022 (71st edition), the global oil consumption in 2022 is still lower than the level in 2019, while natural gas consumption is greater than in 2019, breaking the 4 trillion cubic meter threshold for the first time (BP, 2022). The trend of weak oil and strong gas is more obvious. With the consumption of conventional gas reservoirs, unconventional natural gas sources such as gas hydrate, shale gas, and tight gas have become increasingly significant due to their high potential (Song et al., 2014; Vedachalam et al., 2015; Zou et al., 2018). Gas hydrate, also known as combustible ice (see Figure 1), is one of the most elusive natural hydrocarbons (John et al., 2020). They can pose a significant danger to deep-water oil and gas operations while also representing a potentially huge untapped clean energy supply (Pelley, 2008; Dong and Zeng, 2017). As an important alternative energy, its commercialization will play a major role in driving the energy production and consumption revolution, as well as providing a way to handle the challenges of security, the economy, and low-carbon energy.
It is estimated that 97–99% of the discovered gas hydrate resources in the world are located in the ocean, and only 1–3% are distributed on land (Merey and Chen, 2022). Gas hydrate also exists in deep-water areas of some inland seas and lakes, such as the Black Sea and Lake Baikal (Gerivani et al., 2020; Yevgeny et al., 2021). Nevertheless, people are more concerned about the gas hydrate stability zone (GHSZ) with considerable thickness in the ocean because the thick layered hydrate-bearing sediments generally do not appear in the inland seas and lakes. Deepwater drilling is one of the key elements of offshore oil and gas engineering (Li et al., 2020b). Although the globe has extensive expertise in conventional oil and gas drilling operations, deep sea drilling experience in unconventional oil and gas deposits, particularly gas hydrate, remains limited (Wang and Gao, 2022). In the past decades, many drilling expeditions for gas hydrate in marine sediments have been successfully carried out around the world. The well-known hydrate drilling expeditions include the Deep Sea Drilling Project (DSDP), the Ocean Drilling Program (ODP), the Integrated Ocean Drilling Program (IODP), the International Ocean Discovery Program (IODP), the Gulf of Mexico Gas Hydrate Joint Industry Project (GOM JIP), the National Gas Hydrate Program (NGHP), the program of Guangzhou Marine Geological Survey (GMGS), the program of Ulleung Basin Gas Hydrate (UBGH), and the hydrate investigation activities carried out by the Research Consortium for Methane Hydrate Resources in Japan (MH21), and the Ministry of Economy, Trade and Industry (MITI) (Winters et al., 2008; Hsiung et al., 2019; Zhong et al., 2021; Park et al., 2022; Xu et al., 2022). Although these drilling operations are located on the shallow surface of the seabed, drilling hydrate wells is considered extremely challenging due to the complex environment in deep water and the thermodynamic properties of the hydrate. From October 2019 to April 2020, the China Geological Survey organized and carried out the second offshore gas hydrate pilot production with the depressurization method (Ye et al., 2020). Based on the previous depressurization pilot production, this round of pilot production broke through horizontal well drilling and production techniques, making China the first country in the world to deploy this technique for offshore gas hydrate pilot production. Nonetheless, there is a significant gap between this trial production and the goal of long-term, safe, stable and efficient industrialized development and utilization of gas hydrate reservoirs. Therefore, international research teams are working hard to develop efficient drilling techniques to exploit gas hydrate sources in a long-term and stable manner. It is known with certainty that laboratory, virtual simulation, and fieldwork have provided an impetus for the development of gas hydrate drilling technology. However, when it comes to the current situation of drilling technology, it is found that only a few reviews comprehensively summarized the development of hydrate drilling techniques.
In this review, the latest gas hydrate drilling technologies and further research and development opportunities are summarized and introduced, including the gas hydrate drilling techniques, wellbore structure, drilling fluid system, equipment and tools, coring techniques, and field application, etc. Meanwhile, we point out the gaps and put forward some suggestions for future studies, which can provide insightful guidance for comprehensively understanding the drilling of the gas hydrate reservoirs.
Overview of gas hydrate
Under high pressure and low temperature, methane and/or other small non-polar or slightly polar hydrocarbons can form hydrates with water. Some inorganic small molecules, such as CO2 and H2S, are also very efficient hydrate formers. Gas hydrate reservoir development typically requires four basic conditions, namely the presence of a water phase, a channel and/or space for fluid migration and accumulation (Zhang et al., 2021a), as well as a low-temperature and high-pressure environment and a suitable gas source. Therefore, one of the prerequisites for the production of large-scale gas hydrate deposits is the geological structure that faults, fractures, and porous strata may deliver hydrocarbon gases from deep (Liang et al., 2019). The formation of faults and fractures is closely related to tectonic activities, sedimentary responses, and over-pressurized fluids. Non-local gas sources along fault paths have been confirmed to play a role in gas hydrate formation in the South China Sea, the Gulf of Mexico, etc. (Zeng et al., 2022). The analysis of gas hydrate systems and environmental assessment in these areas rely heavily on fluid flow migration, accumulation process, and sealing integrity. For example, the widely distributed faults in Baiyun Sag in the Pearl River Mouth Basin have strong geological activity, which has experienced three evolutionary stages of rifting, transition, and subsidence (Liang et al., 2022a). Natural gas diffusion and advection in deep sediment supply a significant amount of gas to the Shenhu area. In Woolsey Mound in the northern Gulf of Mexico, hydrocarbon fluids migrate from deep reservoirs thousands of meters deep through faults and fractures to shallow sediments with high porosity and/or fractured formations (Macelloni et al., 2015). Deep fluid seepage channels typically penetrate the overlying strata in these locations, producing hydrocarbon gas and causing geochemical anomalies such as isotope anomalies, pore water ion concentration anomalies, organic carbon and water content abnormalities (Hu et al., 2017; Monteleone et al., 2022). To identify gas hydrate, geophysical methods such as multi-channel seismic surveys, seabed profiles, and controlled source electromagnetic (CSEM) are frequently used (Minshull et al., 2020; Liang et al., 2020; Crutchley et al., 2010). Bottom-simulating reflectors (BSR), a special physical interface generated by seismic profiles, are the earliest and most widely used, reliable, and intuitive geophysical markers to confirm the occurrence of gas hydrate (Foschi et al., 2019; Colin et al., 2020). Seabed geological sampling, microbial exploration techniques, and seabed visual exploration techniques such as remotely operated vehicles (ROV), ocean floor observation systems (OFOS), TV grabs, and deep-towed systems provide a more robust foundation for determining the presence of gas hydrate (Su et al., 2020). Logging while drilling (LWD) has been widely used in drilling to acquire parameters such as resistivity, acoustic waves, porosity, radioactivity, electromagnetic characteristics, and saturation (Saumya et al., 2019; Boswell et al., 2020; Kim et al., 2022). Whereas pressure coring is the most direct, accurate, and persuasive method in gas hydrate exploration, which is primarily used to recover unspoilt rock columns to study the formation mechanism, shape, accumulation type, and physical chemical properties of gas hydrate, etc. (Gaafar et al., 2015; Wang et al., 2020a; Singh et al., 2022a).
According to the research, about 90% of the ocean and about 27% of the land have favorable conditions for the generation of gas hydrate (Ruan et al., 2012), thus the ocean is the primary target of gas hydrate exploration and development. Fracture systems through the sealing structures, which connect to the ocean and bring in seawater will lead to hydrate dissociation due to the low concentration of CH4 in seawater (Kvamme and Saeidi, 2021). If the seafloor openings of the fractures are at hydrate-forming depths and temperatures are within the range of hydrate generation, hydrate mounds will form on the seafloor. This type of seafloor hydrate mound can be found all over the world and gives rise to bio-geo ecosystems. Large-scale active cold spring outcrops, for instance, were found on the seafloor of the W01 and Haima sites in the Qiongdongnan Basin, as well as a large number of live mussels, shrimp, crabs, and buried authigenic carbonates, carbonate crusts (He et al., 2022). Gas hydrate occurs in submarine sediments on the active and passive continental margins in nature, as well as permafrost. It is found most commonly in accretionary wedges on active continental margins and geological structures such as mud volcanoes, mud diapirs, gas chimneys, structural faults, polygonal faults, and pipes on passive continental margins (Hu et al., 2021). The hydrate reservoirs found in marine environment are distributed around the world (Chong et al., 2016; Ruppel, 2020; Hu et al., 2021), as shown in Figure 2. Data from seismic observation and gas hydrate drilling projects show that gas hydrate resources are abundant throughout the world, with an estimated 0.2–3 × 1018 m3 (Singh et al., 2022b). Table 1 shows the estimation of gas hydrate resources in some parts of the world (Sain and Gupta, 2012; Liu et al., 2019a; Sahu et al., 2020; Chibura et al., 2022). According to the conservative estimation of Pang et al. (Pang et al., 2022), the amount of technically recoverable gas hydrate resources on the planet could range from 190 × 1012 m3 to 700 × 1012 m3.
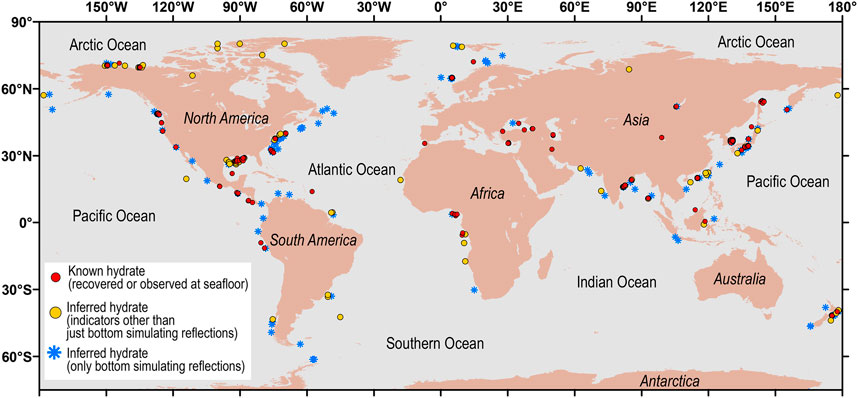
FIGURE 2. Schematic diagram of gas hydrate distribution (reproduced from Ruppel (Ruppel, 2020)).
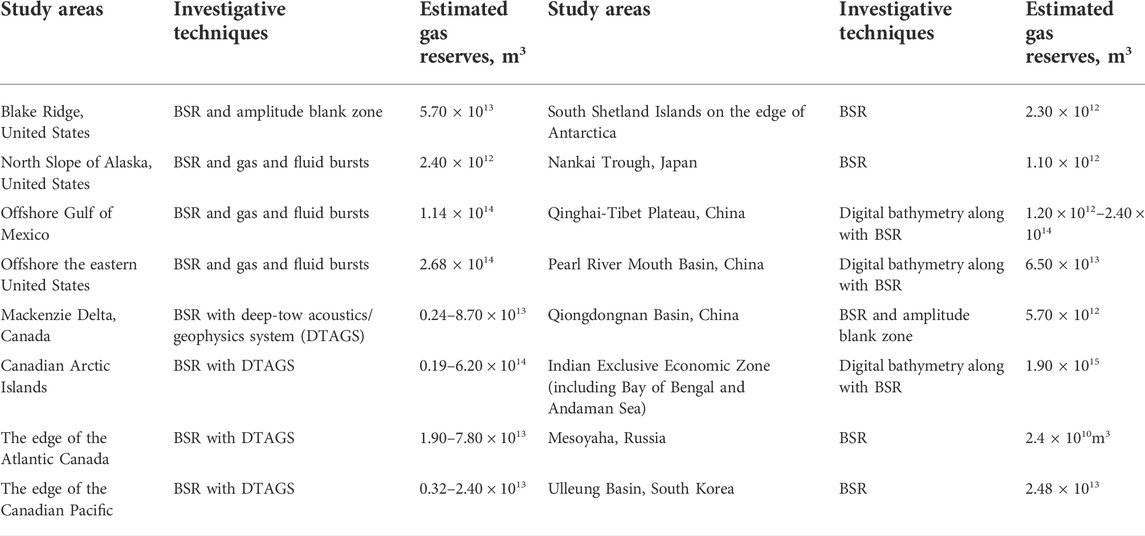
TABLE 1. Distribution of gas hydrate resources (Sain and Gupta, 2012; Liu et al., 2019a; Sahu et al., 2020; Chibura et al., 2022).
Production methods and drilling challenges
Depressurization, thermal stimulation, inhibitor injection, CO2 replacement, and solid-state fluidization methods are currently being used in field experiments for gas hydrate development (Olga et al., 2022; Wei et al., 2022; Zhou et al., 2022). The depressurization method refers to the dissociation of gas hydrate into gas by reducing the pressure of the hydrate reservoir in equilibrium (extracting formation fluid or exploiting underlying free gas). This method has a simple process and low cost, but there are problems such as slow production, sand production, and geological stability (Liu et al., 2019b; Yang et al., 2019; Liu et al., 2022; Olga et al., 2022). The thermal stimulation method refers to the dissociation of gas hydrate into natural gas by increasing the reservoir temperature to break the phase equilibrium (heating by hot fluid, microwave, electromagnetic energy, or solar energy) (Roostaie and Leonenko, 2020a; Roostaie and Leonenko, 2020b; Liu et al., 2022). This approach is easy to control, efficient, and pollution-free, however, it is expensive due to high energy consumption, heat loss, low injection rate, and weather sensitivity. The inhibitor injection method makes the gas hydrate easier to decompose into natural gas (Sung et al., 2002; Demirbas, 2010). This method is simple and convenient, and the energy consumption of injection at the initial stage of production is low, but it has the problems of high cost, slow reaction, low efficiency, environmental pollution, etc. In an environmentally friendly way, CO2 or other gas that is more likely to form hydrate under reservoir conditions is used to replace the methane molecules in the hydrate crystal structure. This CO2 replacement method can reduce geomechanical hazards, reduce water yield, and be environmentally friendly, but it has problems such as slow speed, low injection rate, low replacement rate, etc. (Uchida et al., 2005; Fakher et al., 2019; Heydari and Peyvandi, 2020). The solid-state fluidization method breaks the solid gas hydrate in the formation first and then fluidizes it into hydrate slurry by mechanical means, and then lifts it to the surface treatment facilities through the transmission pipeline (Zhou et al., 2018; Wei et al., 2019a; Zhou et al., 2022). This method constructs an artificially closed area with controllable hydrate dissociation, which can realize the exploitation of gas hydrate in deep water and shallow sediment. However, the key theory, process, and technology are still in the experimental stage. Technical feasibility, market acceptance, and environmental permission are the three decisive factors for the realization of commercial exploitation of resources. To ensure production safety and the improvement of development efficiency, new mining techniques or combined mining techniques like the depressurization-solid fluidization combined method, CO2 replacement assisted depressurization method, electric heating assisted depressurization method, and inhibitor injection-replacement combined method should be further studied.
In permafrost and marine environments, many gas hydrate drilling operations have been completed successfully during the past decades (Dallimore et al., 2002; Chuvilin et al., 2020; Zhang et al., 2020; Liang et al., 2022b). Presently, over 150 gas hydrate wells have been drilled around the world (Makogon, 2010; Zhang et al., 2020). Most gas hydrate drilling activities, including those in the United States, China, India, and South Korea, aim to demonstrate the presence of hydrates in the target area and identify their properties. Hence, most of the hydrate wells deployed around the world are exploration wells, with only a few pilot production wells scattered throughout the Mackenzie Delta in Canada, the North Slope of Alaska in the United States, the Messoyakha in Russia, the Nankai Trough in Japan, the Qilian Mountains in China, and the South China Sea (Sun et al., 2021; Sun et al., 2022). The drilling of these pilot production wells has provided valuable experience for the production operations of the whole industry. However, the technological barriers between countries, complex on-site working conditions, and unique physical chemical properties, accumulation forms, and occurrence characteristics of gas hydrate make the drilling of hydrate wells still full of unknowns and challenges. So far, the natural gas produced in the trial production has not yet crossed the industrialization threshold (Wu et al., 2021; Chen et al., 2022), as shown in Figure 3.
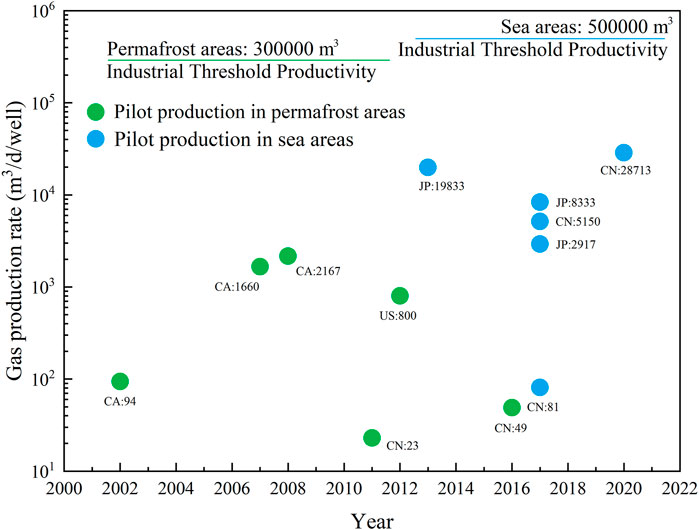
FIGURE 3. The daily production capacity of gas hydrate pilot production has not yet crossed the industrialization threshold (modified from (Wu et al., 2021; Chen et al., 2022)).
Most hydrate drilling operations are offshore, so the risks and challenges faced by drilling hydrate wells are similar to those of most deep-water drilling operations. In addition to the formation stability problems of weak formation cementation, poor diagenesis, and low strength, there are numerous other problems caused by deep-water drilling tripping fluctuation pressure, deep-water string vibration, shallow gas, shallow water flow, and deep-sea harsh environment (Khabibullin et al., 2011a; Khabibullin et al., 2011b; John et al., 2020; Huang et al., 2020; Li et al., 2021a). The gas hydrate in the Shenhu area of China occurs 203–277 m below the mudline. The predicted equivalent density of fracture pressure of the target layer is only 1.14–1.15 g/cm3, and the equivalent density of pore pressure is 1.03–1.05 g/cm3 (Hou et al., 2022). The narrow mud density window, or perhaps a zero density window, induced by high collapse pressure and low fracture pressure in shallow formation makes controlling downhole pressure difficult, and it is very easy to create downhole mishaps such as leakage and collapse. We must consider the occurrence as well as the specific physical and chemical features of gas hydrate when drilling the hydrate reservoirs. The change of phase equilibrium increases the danger of gas hydrate dissociation. So when drilling hydrate wells, great attention should be paid to maintaining the low temperature and high pressure state of the reservoir, or making it more stable by changing the phase equilibrium of gas hydrate. Some common problems and challenges related to gas hydrate dissociation recorded in previous drilling operations include but are not limited to the following (Prassl et al., 2004; Khabibullin et al., 2011b; Li et al., 2018a; Wang et al., 2020b; Li et al., 2021b; Hou et al., 2022). The extremely narrow safe density window, for example, makes downhole temperature and pressure control more difficult than in other deep-water operations; Temperature and pressure fluctuations (swabbing, surge, etc.) during tripping or downhole tool movement lead to hydrate dissociation; Hydrate dissociation leads to a loose reservoir framework and aggravates wellhead/wellbore/formation stability problems; Environmental and engineering disasters caused by gas escape and leakage after hydrate dissociation; The secondary formation of gas hydrate near or in the wellbore; Complex multiphase flow of hydrate containing fluid in a wellbore; Rheological property of the drilling fluid; Gas hydrate form around the drill string (riser and casing) or on surface equipment (wellhead, BOP, kill line, and choke manifold). In a nutshell, hydrate drilling seeks to be successful, good, fast, and economical. All engineering measures should be taken to prevent gas hydrate dissociation or secondary formation during operation and to ensure that secondary disasters caused by gas hydrate dissociation are under control.
Gas hydrate drilling techniques
Drilling a gas hydrate reservoir can generally use the slightly overbalanced drilling method, which means keeping the pressure in the borehole slightly higher than the formation pore pressure (but less than the formation fracture pressure) to prevent the wellbore instability caused by the dissociation of gas hydrate and subsequent methane overflow. Driven by the pressure difference between the bottom hole and the formation, the drilling fluid continuously seeps into the formation during the invasion process. In this process, there is not only fluid migration and material transfer but also changes in temperature, pressure, and pore water salinity (Ning, 2005; Fereidounpour and Vatani, 2014; Dong et al., 2022). The change in salinity will cause the deviation of the hydrate phase equilibrium curve, and the change in equilibrium conditions will cause the dissociation of gas hydrate, which is likely to cause uncontrollable drilling risks (Ning et al., 2013a; Ning et al., 2013b). Therefore, in order to effectively protect the reservoir and avoid gas hydrate dissociation during drilling, the drilling technique must be strictly evaluated for necessity, feasibility and technical economy according to the actual situation of the target area.
The development of drilling technology is changing with each passing day. At present, the developed drilling techniques applicable to gas hydrate reservoirs mainly include managed pressure drilling (MPD), casing while drilling (CWD), coiled tubing drilling (CTD), LWD, etc. (Wu et al., 2021; Merey and Chen, 2022). By actively managing the annulus pressure profile, MPD, an adaptive drilling method, can considerably improve drilling controllability, solve the issue of a narrow density window, and lower the likelihood of accidents (Pui et al., 2017). Compared with overbalanced drilling, MPD can effectively reduce reservoir damage, wellbore instability and operating costs. Halliburton, Schlumberger and Baker Hughes have more than 40 years of theoretical and practical experience in this field. In the field experiment of gas hydrate exploration well Hot Ice #1 in the Arctic region of Alaska, the well control and blowout preventer equipment (BOPE) stack was managed by MPD technique (Kadaster et al., 2005). Dual gradient drilling (DGD), a method of MPD, limits the total hydrostatic pressure through the density of two different annular fluids in the wellbore to avoid exceeding the fracture pressure gradient. Thereinto, riserless DGD is an economical, efficient, and safe gas hydrate drilling method, which can improve the integrity and safety of drilling (Rosenberg et al., 2022). The drilling vessel or platform used in the riserless drilling has lighter loads, allowing drilling in deeper oceans. It has low drilling cost and less likely to jam the drilling tools. However, some unavoidable problems need to be solved. For example, the exposed drill pipe has a long suspension section in the sea water, so high-strength drill pipe shall be selected; The drilling rig shall be provided with the function of drilling string motion compensator. China and other developing countries are still in the early stages of research and development in this field. With the acceleration of the development of deep-sea oil and gas resources in various maritime sovereign countries, the research and development of DGD and key equipment are imminent. International commercialization of DGD techniques has occurred, and the DGD system represented by Riserless Mud Recovery (RMR), Subsea Mudlift Drilling (SMD), DeepVision, Subsea Pumping System (SSPS), and Hollow Glass Spheres (HGS) has been established (Chen et al., 2007; Naser et al., 2022). Concentric drill pipe reverse circulation DGD technique, riser level control DGD technique, riserless subsea pump lifting DGD technique, dual density drilling technique, and other DGD schemes have been developed at the same time. To employ DGD to produce deep-water oil and gas hydrate safely and effectively, we need to create a dual gradient drilling technique with more versatility. Southwest Petroleum University has proposed a new technology for safe drilling of deep-water gas hydrate with double-layer pipe DGD system and structural scheme, but it has not yet reached the level of commercial application (Wang et al., 2019a).
As early as around 2004, Hannegan et al. (Hannegan, 2005; Hannegan et al., 2005; Todd et al., 2006) put forward the view that MPD is suitable for gas hydrate drilling, and believed that CWD may have a unique application in gas hydrate drilling. CWD applies torque and weight on bit (WOB) by replacing drill pipe with casing, and runs casing while drilling, thus saving time and operation cost (Sánchez and Al-Harthy, 2011). The CWD and casing-bit system allows drilling through the problem area at a relatively low flow rate and setting casing to avoid hole enlargement (Motghare and Musale, 2017). The lower flow rate also makes it possible to use smaller and lighter drilling equipment. In addition, the plastering effect in CWD can form a filter cake with low porosity and low permeability on the wellbore. This effect can reduce or prevent leakage, expand the safe density window of drilling fluid, improve borehole stability and reduce formation damage effectively, which is critical for the design of gas hydrate drilling engineering (Briner et al., 2015). CWD has been verified by a large number of practices and has been successfully applied to gas hydrate drilling operations in the Canadian Arctic permafrost (Vrielink et al., 2008). Managed pressure casing drilling (MPCD) is built on MPD and leverages CWD techniques to handle the problem of downhole high pressure management in the process of open hole logging after tripping out, ensuring that the pressure control mechanism is in place (Balanza et al., 2015). At present, MPCD has been successfully applied in well Gao 124 - Geng 30, well Gao 132 -Geng 33, well Bei 3 - Ding 5 - Geng P34 and well Bei 3-342 - Geng P51 in Daqing Oilfield (Liu et al., 2020; Liu et al., 2021), and it is expected to be applied to gas hydrate drilling in China in the future.
CTD technique also shows its potential in this field. In 2016, the small hole (small diameter) directional drilling technique, one of the CTD techniques, was first applied to the gas hydrate production wells in the Muli permafrost area, Qinghai (Li et al., 2017a), making China the first country to successfully connect two wells in high-altitude areas using this technology. CTD technique is also used in the drilling of gas hydrate well during the solid fluidization production in the South China Sea. Moreover, LWD, which can drill and log at the same time, is extremely useful in identifying and evaluating producible gas hydrate deposits, as well as acquiring and quantifying critical parameters of gas hydrate production. Due to its widely application in the mineral exploration, it is also recommended to use reverse circulation drilling (RC) technology in gas hydrate drilling (Zhang et al., 2015). As summarized in Table 2, these drilling techniques have their pros and cons. Specific techniques and processes need to be selected according to the actual situation.
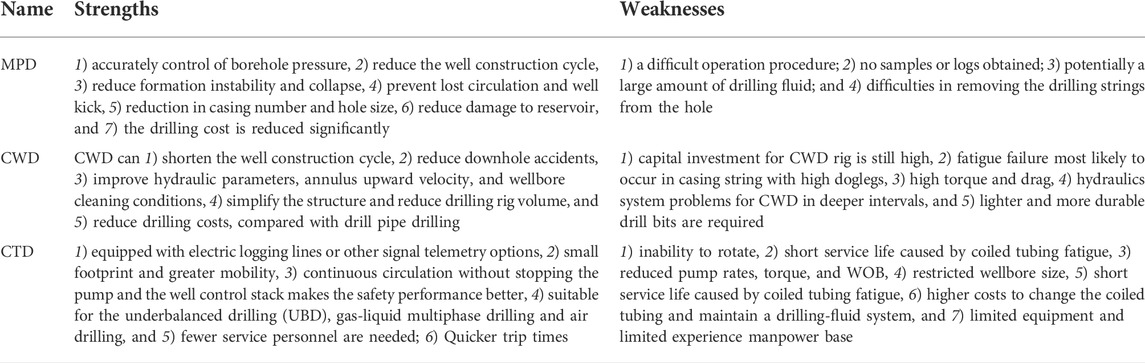
TABLE 2. Characteristics of drilling technologies suitable for gas hydrate exploration and development.
Wellbore structure and well trajectory
Because gas hydrate mostly occurs in the semi-consolidated or even unconsolidated mud and sand layer on the seabed, the reservoir after hydrate dissociation is likely to cause wellbore instability. Proper wellbore structure, wellbore trajectory, and borehole reaming are not only conducive to the stability of the wellbore but also help to improve the productivity of gas hydrate. Before designing the well structure and well trajectory, it is necessary to ascertain the potential drilling location of exploration wells or production wells according to the exploration and research results of BSR, GHSZ, formation temperature and pressure, reservoir properties, plume, hydrate mound, etc. Thereafter, design the casing program based on the downhole pressure profile and pressure balance.
Gas hydrate development is currently at the exploratory and pilot production stages worldwide. The chosen drilling location’s geological characteristics are not very complicated. Most exploration wells adopt open hole drilling without a riser, and the designed well type and well structure are relatively simple. Only China has so far broken through in the drilling and production techniques of horizontal wells in the gas hydrate field experiment. For comparison, other explorations or pilot production wells are vertical wells. During the second gas hydrate production test in the South China Sea with the depressurization method, the maximum bending degree of the horizontal wellbore reached 15.2°/30 m (Ye et al., 2020). By increasing the contact area between the wellbore and the reservoir and expanding the gas hydrate dissociation area, the hydrate dissociation rate and productivity were greatly improved during this field experiment.
Both domestic and international academics have theoretically analyzed and demonstrated different well types and well layouts strategies, such as vertical wells, horizontal wells, and multilateral branch wells. Moridis et al. (Moridis et al., 2008) studied the productivity improvement of horizontal wells in different types of hydrate reservoirs compared with vertical wells at the earliest. Since then, a large number of experiments and theoretical studies have emphasized the advantages of horizontal wells in gas hydrate production. Li et al. used the parallel version of the TOUGH+HYDRATE code to simulate the gas production of the two-point horizontal well system of permafrost hydrate in the Qilian Mountains and evaluate its commercial feasibility (Li et al., 2015). The stimulation measures under different well structures have been compared by many scholars since the production efficiency of gas hydrate is one of the most concerning issues. Jin et al. recently used TOUGH+HYDRATE and GMS software to research the new radial multi-branch horizontal wells in the Shenhu area to improve the gas production rate (Jin et al., 2022). It was found that as the well’s overall length increases, radial branch horizontal wells produce more water and gas. The production of gas and water from multi-branch wells is greatly influenced by depth, while the rate at which hydrates dissociate is hardly affected.
Figures 4, 5 show the information of wellbore structure and well trajectory adopted in several gas hydrate pilot production experiments. Figures 4A–E respectively show the structure of production wells of the first offshore pilot production in Japan, the second offshore pilot production in Japan, the first offshore solid fluidization pilot production in China, the first offshore depressurization pilot production in China, and the second offshore depressurization pilot production in China. It can be seen from these trial productions that the current drilling depth of offshore gas hydrate is about 1000–1400m, the test depth is 200–500 m. Except for the solid fluidization production well, 914.4 mm conductors are used in the first spud, and 339.7 and 244.5 mm casings are used in the second spud and the third spud, respectively. Figures 5A–C depict the well Ignik Sikumi # 1 in Alaska, the well Mallik 5L-38 in Mackenzie Delta, and the well SK in Qilian Mountains. Similarly to marine hydrate drilling, hydrate drilling in permafrost regions mainly adopts vertical well structures at present. For example, the vertical well Mount Elbert # 1 on the North Slope of Alaska was drilled to the hydrate formation using a 12 1/4-inch bit, water-based mud, and LWD tools (Sun et al., 2011). The 9 5/8-inch diameter casing was fixed slightly below the bottom of the permafrost (594 m). The drilling of gas hydrate layer was further drilled with a 7 7/8-inch bit and oil-based mud. Coring from the bottom of the casing to a depth of 760 m, then the drilling was deepened to 914 m. The hole was reamed to a diameter of 8 3/4 inches at last.
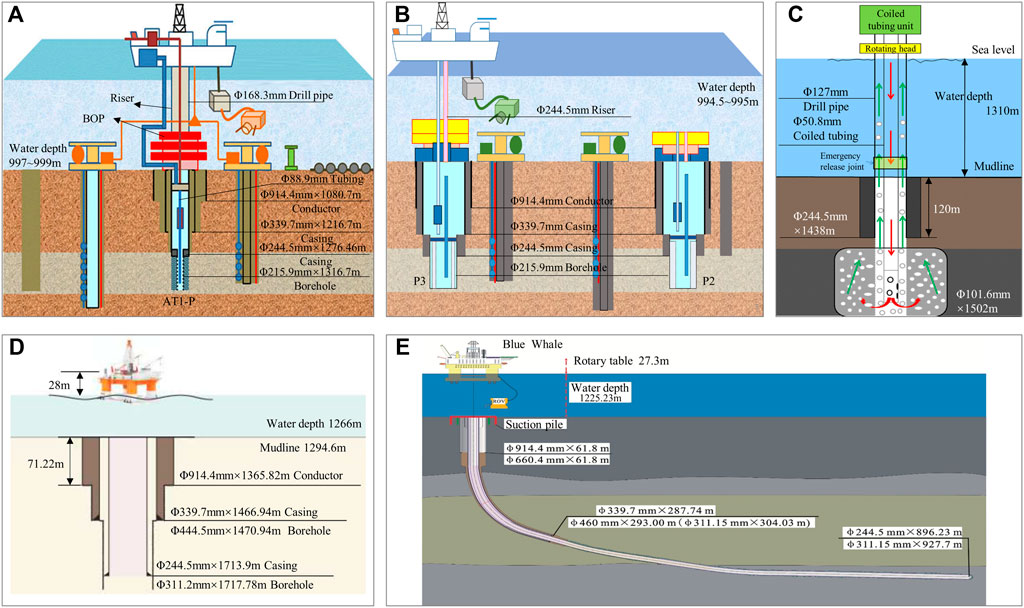
FIGURE 4. Wellbore structures of offshore gas hydrate pilot production wells. (A) the first offshore pilot production well in Japan, (B) the second offshore pilot production well in Japan, (C) the first offshore solid fluidization pilot production well in China, (D) the first offshore depressurization pilot production well in China, and (E) the second offshore depressurization pilot production well in China. (Matsuzawa et al., 2014; Yamamoto et al., 2014; Li et al., 2019; Yamamoto et al., 2019; Ye et al., 2020).
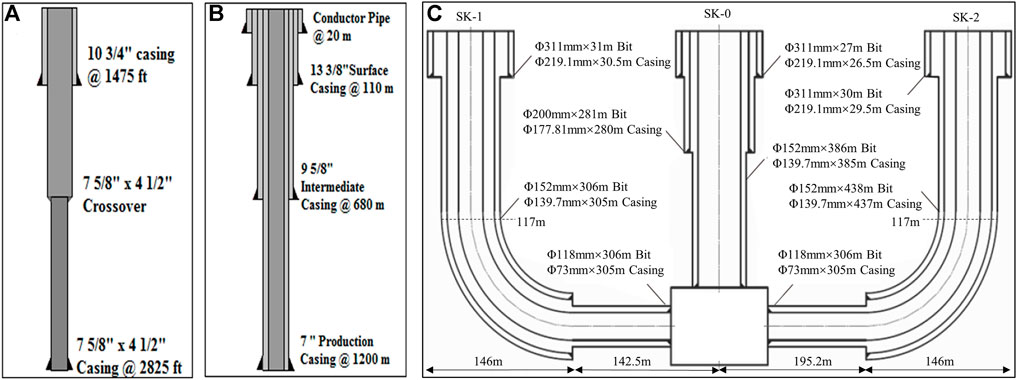
FIGURE 5. Wellbore structures of permafrost gas hydrate pilot production wells. (A) the well Ignik Sikumi # 1 in Alaska, (B) the well Mallik 5L-38 in Mackenzie Delta, and (C) the well SK in Qilian Mountains. (Merey, 2016; Li et al., 2017b; Merey, 2019; Zhu et al., 2020).
So far, the most complex gas hydrate production wells are SK-0, SK-1, and SK-2 in the Muli permafrost area of the Qilian Mountains. The well trajectory is divided into three sections, as shown in Figure 5C, namely the vertical section, the build-up section, and the horizontal section. The measuring while drilling (MWD) technique was used to control the wellbore trajectory in butt joint horizontal well drilling, and the drilling in the build-up section and horizontal section was completed by a single-bend positive displacement motor (PDM) and a polycrystalline diamond compact (PDC) bit (Li et al., 2017b). The build-up section of well SK-1 started from the depth of 95 m. In the first curve section, the buildup rate was controlled at about 10.5°/30 m. When drilling to a depth of about 300 m, the inclination angle increases to 64°. The buildup rate in the later curve section was controlled at about 9°/30 m until the hole depth reached 340 m and drilling passed through the first layer of gas hydrate. The maximum inclination angle of well SK-1 was 83, and the length of the horizontal section was designed to be short. Lastly, SK-1 extended to the bottom hole butt-joint area of SK-0. The build-up section of the SK-2 well was designed to continuously increase the inclination angle. The kick-off point was set at a well depth of 80 m, and the buildup rate in the curve section was controlled at about 10°/30 m. The SK-2 connected with SK-1 and SK-0 in the butt-joint area at last. The completion of SK-1 and SK-2 has greatly promoted the development of hydrate experimental technology and numerical simulation technology under different well types in China and even other countries in the world.
Generally speaking, marine hydrate drilling faces softer formation, poorer drillability, and greater difficulty in casing running when compared to hydrate drilling in permafrost. In the weakly cemented formation on the seabed, the orientation of horizontal wells and the extension of horizontal sections are more difficult. We therefore need to accelerate the progress of marine hydrate drilling and production. It will be important to build a theory of increasing production and a technology system to address the issue of gas hydrate development efficiency. Complex wells significantly enhance the exposed area of gas hydrate as well as the controlled reserves of wells, thus increasing output and also the production efficiency of single wells. Directional wells, multi-branch wells, cluster wells, complex well patterns, etc., as shown in Figure 6, may be the main ways to increase the production of gas hydrate in the future (Ning et al., 2022), (Mahmood and Guo, 2021), (Zhang et al., 2022), (Yu et al., 2021), (Li et al., 2013), (Ye et al., 2021), (Li et al., 2011). Accordingly, the complex wells also put forward higher requirements for gas hydrate well structure and well trajectory.
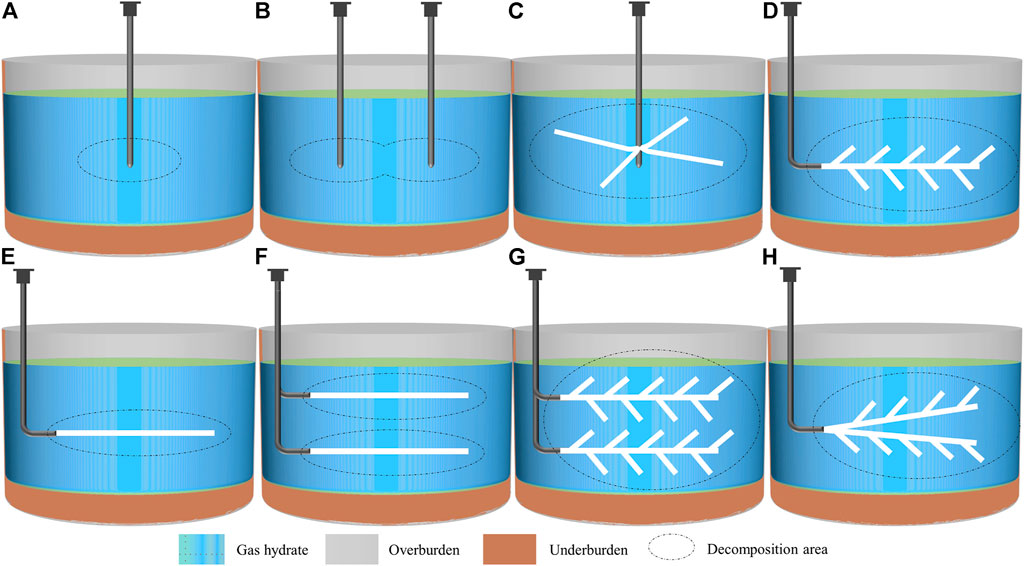
FIGURE 6. Wellbore structure model of gas hydrate production well to improve gas production efficiency. (A) Single vertical well, (B) dual vertical wells, (C) radial lateral well, (D) fishbone well, (E) single horizontal well, (F) dual horizontal wells, (G) dual fishbone well, and (H) dual branch multilateral horizontal well.
Drilling fluid system
During the drilling of hydrate reservoirs, the drilling fluid in the wellbore will exchange material and energy with seawater. The original pressure and temperature field will be disturbed by the circulating drilling fluid interacting with the hydrate particles when it enters the hydrate deposit through holes and throats (Chen et al., 2019). As drilling fluid penetrates into the formation, the physical characteristics of the hydrate-bearing sediments will change as a result of the superposition of numerous causes. One of the fundamental characteristics of drilling fluid invasion into hydrate formation is the phase change of gas hydrate, which is also the primary distinction between drilling fluid invasion into gas hydrate formation and conventional oil and gas reservoir. The interaction process between drilling fluid and formation is a process involving the coupling of drilling, rock mechanics, rock mineralogy, chemistry, and dynamics of fluids in porous media (Teymouri et al., 2020; Ruan et al., 2021). This process has an impact on the mechanical, electrical, thermal, and permeability properties of the formation around the wellbore. At the same time, it also changes the geomechanical stability of the formation, the reliability of resistivity logging during drilling, heat transfer, the following gas production rate, etc. (Ning et al., 2013b). This is closely related to many complex downhole issues and is a world-class problem that has not been well solved on a worldwide scale. It is vital to prevent drilling fluid from entering the formation during the deep water drilling and gas hydrate development processes because it poses a severe danger to safe and successful drilling. In the process of Qilian Mountain drilling exploration, for instance, the boreholes of QTZK2 and SK-2 collapsed and were buried due to drilling fluid problems (Gao et al., 2017). Even if the formula and performance of the drilling fluid are adjusted, the wellbore is still unstable during subsequent operations. It can be seen that research and development of drilling fluid systems to solve the key technical challenges of hydrate drilling, whether on land or at sea, is the development direction, frontier subject, and good opportunity for drilling fluid technology.
At present, the commonly recognized method is to maintain the thermodynamic stability of gas hydrate by increasing the well pressure and reducing the mud temperature. This method primarily employs water-based drilling fluid, oil-based drilling fluid, and synthetic-based drilling fluid systems for gas hydrate exploration and drilling (He et al., 2021; Das et al., 2022a). Oil-based drilling fluid is generally used in hydrate drilling activities in permafrost, such as the production well of the Ignik Sikumi oilfield in the United States (Grigg and Lynes, 1992; Schoderbek et al., 2013a). It provides excellent hydrate inhibition performance and antifreeze properties, and will not induce hydrate dissociation. However, there are several drawbacks, such as environmental pollution, excessive costs, and safety issues. It is highlighted that the drilling fluid should have low toxicity, no bioaccumulation, and be harmless to the environment after discharge. Consequently, from the standpoints of inhibitory effects, environmental protection, and cost management, the water-based drilling fluid system is still the best choice for gas hydrate exploration (Liu et al., 2016a). Table 3 shows the gas hydrate drilling fluid system used in some drilling operations. It can be seen that water-based drilling fluids with hydrate inhibitors such as sepiolite, KCl polymer, lecithin, and attapulgite are widely used.
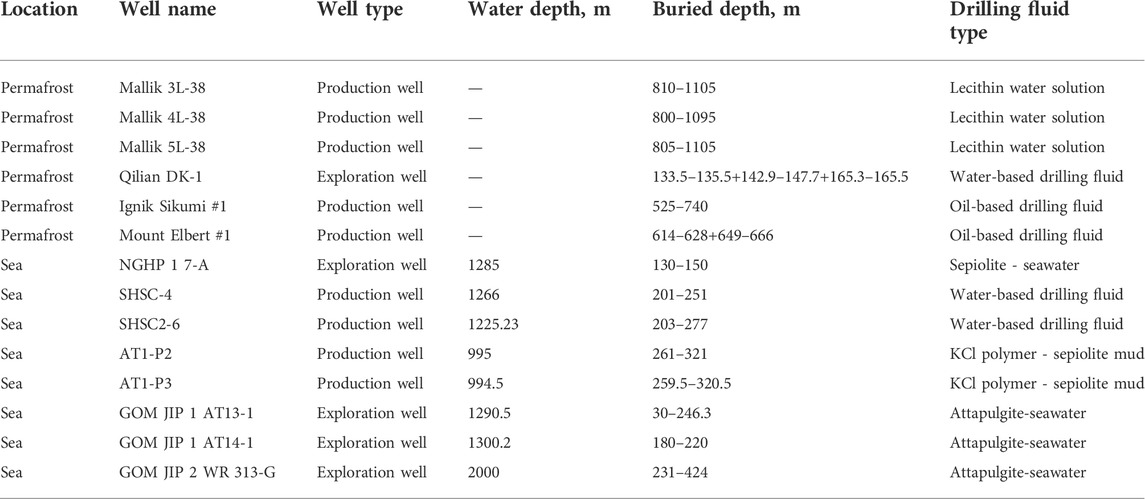
TABLE 3. Gas hydrate drilling fluid system (Burger et al., 2006; Collett et al., 2015; Merey, 2016; Merey, 2019; Wu et al., 2021).
As we know, drilling fluids play an important role in hydrate drilling engineering because they have many functions, such as preventing ice blockage, wellbore lubrication, wellbore cleaning, controlling filtration, and maintaining wellbore stability, etc. (Rana et al., 2021). Reasonable mud density, sufficient inhibition, and sealing ability are the basic indicators for evaluating gas hydrate drilling fluid. Therefore, a low-temperature hydrate drilling fluid system with strongly inhibitive ability, good sealing ability, and enhanced wellbore stability is the key research direction. Currently, the innovation of water-based hydrate drilling fluid mainly focuses on gas hydrate inhibitors, fluid loss control additives, and rheology modifiers. Gas hydrate inhibitors, one of the most concerned groups, are divided into thermodynamic hydrate inhibitors (THIs), kinetic hydrate inhibitors (KHIs), and antiagglomerant hydrate inhibitors (AAs) (Ke and Chen, 2019; Kiran and Prasad, 2021). The functional mechanism of THIs is to change the thermodynamic balance between water molecules and gas molecules by changing the chemical potential of the aqueous solution or hydrate phase to avoid hydrate generation (Kiran and Prasad, 2021). Methanol, ethylene glycol, sodium chloride, calcium chloride, potassium chloride, potassium formate, and amino acids are typical THIs (Sa et al., 2011). However, oil and gas fields are finding it more difficult to endure the pressures of heavy use and the high cost of THIs. For comparison, injection of low-dose KHIs can reduce the cost and harm to the environment compared with injection of high-dose THIs. KHIs are adsorbed on the surfaces of hydrate particles at the initial stage of hydrate crystallization, nucleation, and growth. Shortly afterwards, the cyclic structure of KHIs combines with the hydrate cavity structure through hydrogen bonds, delaying and interfering with hydrate crystal nucleation and preventing further crystal growth (Mozaffar et al., 2016). KHIs are mostly low molecular weight polymers dissolved in the aqueous phase, including polyvinylpyrrolidone (PVP), polyethylene caprolactam, poly-N-vinyllactam polymers, and hyper-branched polyesteramides (Mozaffar et al., 2016; Roostaei et al., 2021). Another kind of low-dosa agent, AAs, such as quaternary ammonium surfactants, alkylaryl sulfonates, and phosphonium salt surfactants, can emulsify the oil-water phase and disperse the water in the oil phase into small water droplets (Chua and Kelland, 2013; Nagappayya et al., 2015). Although the emulsified water droplets in the oil phase can also form hydrates with gas, the generated hydrates are difficult to agglomerate. Thus, gas hydrate cannot be formed normally. Low dosage hydrate inhibitors (LDHIs), including KHIs and AAs, have attracted more and more attention from the oil and gas industry in recent years because of their low consumption, high economy, and good environmental benefits (Kelland, 2006).
As mentioned above, fluid loss control additives and rheology modifiers are the focus of drilling fluid research. Fluid loss control additives such as bentonite, carboxymethyl cellulose (CMC), polyanionic cellulose (PAC), and starch are the materials in drilling mud to reduce filtration rate and improve mud cake characteristics. Rheology-modifier additives such as xanthan gum (XG), guar gum (GG) and polyacrylamide (PAM) have a great impact on the rheological properties of the drilling fluid formula. Some rheological modifier additives can also be good fluid loss control additives; for instance, the widely used and environmentally friendly additives GG and XG can effectively improve the viscosity of the drilling fluid and reduce the filtration. In recent years, some new materials have been widely used to improve the performance of hydrate drilling fluids. On the one hand, it has been discovered that drilling fluid with nanoparticles has a tiny particle size and a large surface area, which has a promising future. The nanomaterials can effectively seal the pore throat of the reservoir and enhance the stability of the wellbore, thus realizing the role of reservoir protection (Ngata et al., 2022; Zamora-Ledezma et al., 2022). On the other hand, the drilling fluid with polymers has also become one of the most widely used drilling fluid systems after years of development. It has good rheological properties and the ability to maintain wellbore stability (Ghozatloo et al., 2015; Gupta and Sangwai, 2019). Therefore, there are many reports about nanoparticles and polymers in the hydrate drilling fluid in recent years. Liu, Das, Maiti et al. (Liu et al., 2016b; Wang et al., 2017; Zhao et al., 2019; Zhang et al., 2021b; Maiti et al., 2021; Yuha et al., 2021; Das et al., 2022b) have developed some drilling fluids with hydrate inhibition, respectively, and compared and verified the effects of these drilling fluids through experiments. See Table 4 for details. A glance at open sources indicates that some traditional ideas and old formulations are not suitable for the new application scenario of hydrate drilling, and new ideas and formulations are emerging. When encountering various complex downhole conditions, only by studying and improving the corresponding drilling fluid technology can we effectively solve the above-mentioned technical problems and challenges encountered in the drilling process. It is necessary to draw on existing experience and comprehensively apply multidisciplinary knowledge to deeply study the mechanism and internal laws behind these problems. The new technology emerging as the times require may effectively solve and improve the overall level of hydrate drilling fluid and completion fluid technology.
Drilling equipment, tools and instruments
Gas hydrate drilling is mainly carried out with the help of general technical equipment in the conventional oil and gas industry, and downhole data is mostly obtained by LWD. The drilling technologies for permafrost hydrate and marine hydrate are distinct because of their varied environments, and as a result, so are the drilling equipment, tools, and instruments that are employed. In most cases, risers are required in the process of marine hydrate pilot production and offshore drilling, but considering the factors such as cost, efficiency, operation window, drilling success rate, etc., most gas hydrate exploration wells are operated on floating drilling vessels or platforms with dynamic positioning systems (DPS) by using riserless drilling technology (i.e., open hole drilling, and the rock cuttings generated are scattered on the seabed), such as the GMGS1 survey missions (Merey, 2016; Cheng et al., 2019; Merey, 2019). In the process of riserless drilling, the drill bit is directly lowered to the seabed through the drill pipe for drilling, and the drilling fluid is injected through the drill pipe and then directly discharged into the sea water or recovered after returning to the seabed (Aird et al., 2019; Cheng et al., 2019; Hancock et al., 2019; Chen et al., 2021; Kulkarni and Heinze, 2022). The main equipment used includes drilling equipment, DPS, and a mud treatment system. The drilling equipment includes a drilling control system and a lifting system. The DPS includes three modes: DP1, DP2, and DP3(DNV, 2013; Hogenboom et al., 2020). The mud treatment system includes a desilter, a desander, a vibrating screen, a centrifuge, a degasser, and other tools and instruments. As the carriers for these devices, jack-up drilling platforms, semi-submersible drilling platforms, and drilling vessels have become the main platforms for marine resource exploration and development with their flexibility and good adaptability. Therefore, regardless of the drilling technology utilized to explore gas hydrate deposits, large-scale drilling platforms or drilling vessels are indispensable.
Large-scale deep-water rigs are mobile national territory and strategic weapons, and people from all walks of life have paid great attention to them. So far, the drilling vessels or platforms used in hydrate drilling internationally include JOIDES Resolution, Fugro Synergy, Fugro Voyager, Chikyu, REM Etive, Helix Q4000, Hai Yang Shi You 708, Uncle John, Helix Q-4000, Blue Whale I and II, etc. Table 5 displays the parameters of some vessels or platforms (Graham and Reed, 1969; Rabinowitz and Garrison, 1985; Gabitto and Barrufet, 2009; Li et al., 2018b; FUGRO SYNERGY, 2021; Graber et al., 2021; Jin, 2021; Liang et al., 2021). Thereinto, Fugro’s geotechnical engineering vessel is a modular drilling unit (FUGRO SYNERGY, 2021), which can provide high-quality geotechnical data and play an important role in several gas hydrate field surveys and scientific drilling activities. And JOIDES Resolution is a specifically designed ODP drilling vessel that is outfitted with laboratories for on-board cores and borehole data processing and analysis. Drilling and coring with ship heave up to 4.9 m is possible with the Active and Passive Heave Compensator (AHC/PHC) systems on JOIDES Resolution (Graber et al., 2021). The schematic diagram of drilling equipment on the JOIDES Resolution is shown in Figure 7. Furthermore, compared to the specifically designed drilling vessels JOIDES Resolution and Chikyu, sister drilling platforms Blue Whale I and Blue Whale II adopt the more advanced DP3 dynamic positioning system, which has reliable, efficient, and sufficient drilling capacity. In addition to those that have been put into commercial operation, some new drilling vessels or platforms are under construction. Recently, China is building a scientific drilling vessel, Meng Xiang, which can be used for gas hydrate exploration and development. In any case, gas hydrate drilling in the ocean is mainly done by large-scale drilling vessels, which virtually increases the exploration cost. In the future, small drilling vessels and submersible drilling equipment with rolled casing shall be developed and improved to reduce costs. MPD, UBD, CWD, and insulated riser drilling, etc., shall be fully applied to solve the problems such as wellhead collapse, wellbore instability and submarine landslide that may be caused in the process of gas hydrate drilling.
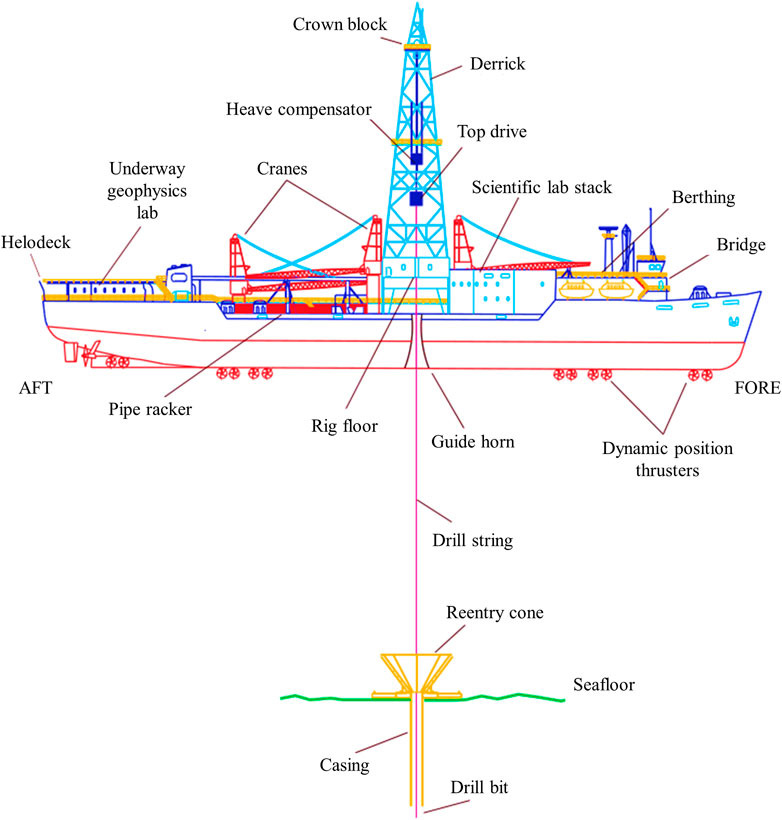
FIGURE 7. Schematic diagram of drilling equipment on R/V JOIDES Resolution (reproduced from (Graber et al., 2021)).
Drilling efficiency, well quality, coring quality, and coring efficiency are closely related to the performance of the bit during gas hydrate drilling. To ensure the coring speed and drilling efficiency of the bit, the commonly used drill bits at present include solid carbide bits and polycrystalline diamond composite (PDC) bits. In addition to selecting the drill bits according to the characteristics of formation lithology before drilling, it is also necessary to carry out targeted design and calculation for drill bit contour, hydraulic parameters, tooth layout, tooth cutting parameters, etc. Many research teams have made great contributions to the development and selection of drill bits for hydrate drilling. In 2002, the Mallik 3L-38 well drilled in the Mackenzie Delta used 7.87-inch Reed PMCKP TJ3210 and TJ3212 bits. The Mallik 4L-38 well used 7.87-inch Reed PMCKP TJ3224 and Hughes JG4XP bits and the Mallik 5L-38 well used 8.74-inch Reed PMCKP Hughes GIHXP and Hughes JD4XP bits (Zhang and Zhu, 2011; Merey, 2016; Wu et al., 2021). The Mount Elbert # 1 well in the North Slope of Alaska used 12 1/4-inch bits between the depths of 0 and 594 m. Due to the defects of water-based mud, oil-based drilling fluid and 7 7/8-inch bits were used below the bottom of the permafrost, effectively improving the drilling rate. Similar operations occurred in the Ignik Sikumi field. The target horizon of the Ignik Sikumi #1 well is between 525 and 740 m, and oil-based drilling fluid and 9 7/8-inch Smith XR + PS5130 and Smith XR + CPS5126 bits are used for drilling (Schoderbek et al., 2013; Merey, 2016). Ignik Sikumi #1 well is designed for hydrate production experiments by the replacement method and the depressurization method. A state-of-the-art gas mixing skid (GMS) designed by ConocoPhillips was used in the experiment (Boswell et al., 2017). During the production of Ignik Sikumi #1, the specially designed GMS, line heater, pump system, and low gas-rate measurement skid ensure the success of the test. In addition, the research institutions led by Jilin University have successfully applied their wear-resistant bionic coupling impregnated diamond bit to the drilling experiment of the MK-2 hole in Mohe County, China. The non-smooth surface of this bionic bit imitates the characteristics of pits in the front chest and back plates of dung beetles. The bottom surface of the drill bit is designed and manufactured into a non-smooth surface (as shown in Figure 8) (Wang et al., 2016; Gao et al., 2018), which makes the bit more wear-resistant and then improves the rock crushing efficiency and service life. Field tests show that the bionic coupling impregnated diamond bit is more suitable for the exploration and drilling of gas hydrates than the ordinary diamond bit. The average drilling rate is increased by about 30%, and the service life of the bit is increased by more than 37% (Sun et al., 2012). Other hydrate drilling bits also include atypical water jet mining heads specially designed for deep-sea shallow surface weak cemented hydrate solid-state fluidization mining (Wang et al., 2019b; Wang et al., 2020c), the straight-rotating mixed nozzles and the porous and rotating porous jet nozzles for cavitating jet drilling radial horizontal wells (Li et al., 2020c).
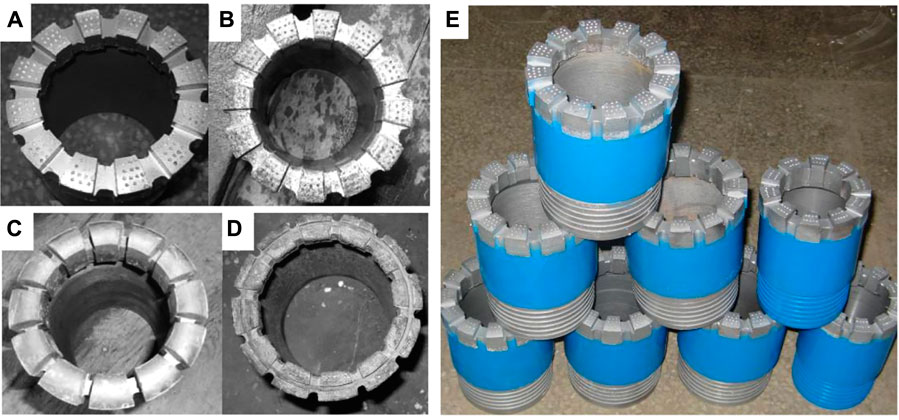
FIGURE 8. Bionic coupling impregnated diamond bit and normal diamond drill bit samples (modified from (Sun et al., 2012)). (A) A new bionic coupling impregnated diamond bit, (B) a used bionic coupling impregnated diamond bit, (C) a new normal diamond drill bit, (D) a used normal diamond bit, and (E) the front view of bionic coupling impregnated diamond bit samples.
To open up the laboratory-exploration-drilling-completion-production-transportation-user chain of gas hydrate development, it is imperative to strengthen the research and application of production equipment systems. For example, the deep-water geotechnical drilling vessel is a major piece of equipment in offshore oil engineering. The design and manufacture of drilling vessels is the integration of many technologies, including ship technology, offshore geophysical exploration technology, drilling technology, and experimental equipment. At present, the drilling of gas hydrates in the ocean is mostly done by large-scale drilling vessels, which virtually increases the cost. In the future, efficient and low-cost drilling equipment combinations should be improved and developed to reduce costs. Devices that should be studied more in the future include but are not limited to economic semi-submersible drilling and production platforms suitable for hydrate exploitation; small drilling vessels; deep-water light drilling systems; seabed drilling tools, riser systems, logging and coring systems, as well as supporting equipment for complex well construction, well trajectory control, pressure control, downhole sand control and other drilling technologies. Table 6 shows the typical technical equipment for offshore gas hydrate drilling (Fu et al., 2020). In the current situation, there is a lack of special equipment for gas hydrate drilling. Conventional oil and gas production equipment plays an important role in hydrate drilling and development and yet they are not perfectly compatible. Moreover, whether the mining technology and equipment used in the short-term pilot production can withstand the test of long-term commercial mining still needs further verification. There will be tougher tests to come.
Gas hydrate drilling coring
Gas hydrate sampling technology consists of seabed sampling and geotechnical drilling (Day, 2006; Ren et al., 2013; Su et al., 2020). The former is used to collect samples of hydrate-bearing sediments on the seabed or in shallow strata, and is a direct method of locating gas hydrate on the sea floor. Seabed samplers mainly include gravity samplers, box samplers, grab samplers, vibration piston samplers, gravity piston samplers, etc. (Su et al., 2020). The recoilless piston corer Advanced Piston Corer (APC) used in ODP, the stationary piston corer STACOR developed by France, the Multiple Autoclave Corer (MAC) and Dynamic Autoclave Piston Corer (DAPC) used in R.V. SONNE cruise in Germany, and the pressure tight piston corer (PTPC) developed by Zhejiang University in China all belong to the above categories (Lunne and Long, 2006; Tommasi et al., 2019). The maximum depth that can be reached by seabed sampling is between a few and tens of meters, but the actual sampling depth is influenced by the geological environment in addition to sampler performance. These samplers frequently fail to sustain pressure during sampling and are unable to assist researchers in getting in-situ samples from deep hydrate reservoirs. High-quality samples can be taken hundreds of meters underground using gas hydrate drilling and coring techniques. On this account, gas hydrate coring technology is considered to be the most direct way to identify and recover gas hydrate in the deep ocean.
The physical properties of hydrate cores under atmospheric temperature and pressure will be very different from native hydrate-bearing sediments (Xie et al., 2016). However, the geotechnical coring tools used in the past are not equipped with the pressure and temperature preservation (PTP) devices, so it is difficult to obtain gas hydrate cores under natural conditions. This situation calls for stringent technical requirements for coring tools, core analysis and transfer systems. So as not to change the physical and chemical characteristics of samples, these devices must be able to prevent gas hydrate from dissociation. Pressure core analysis is considered to be the keystone of hydrate investigation (Schultheiss et al., 2008), but more importantly, the premise of pressure core analysis is to deploy the pressure coring system in hydrate exploration (Sun et al., 2015a; Li et al., 2016b; Hu et al., 2022). The pressure coring system can maintain the pressure of gas and liquid in the rock sample. Compared with traditional methods, pressure coring can improve the evaluation accuracy and avoid more than 50% gas loss (Li et al., 2021c).
The United States and European countries almost monopolize the development and sales of pressure coring technology and equipment. These professional core samplers usually allow drilling to be controlled from the seabed, drilling vessel or drilling platform. Several representative wireline pressure coring systems have been successfully deployed, including the Pressure Coring Barrel (PCB) developed in DSDP (Peterson, 1984; Sun et al., 2015a); The Pressure Coring Sampler (PCS) (Pettigrew, 1992; Dickens et al., 2000) designed by Pettigrew to replace the PCB, which applies to the drill string size used on JOIDES Resolution; The Hydrate Autoclave Coring Equipment (HYACE) developed by the EU-sponsored HYACINTH program, which is a coring tool system developed based on the experience of using PCS during ODP navigation (Amann et al., 1997); Two wireline coring tools, the HYACE Rotary Corer (HRC) for cutting rotary cores in lithified sediment and the Fugro (Rotary) Pressure Corer (FPC or FRPC) for sampling unlithified sediments, are included in the HYACE; The Pressure Temperature Coring System (PTCS) invented by Aumann & Associates Inc. (AAI) and the Japan National Oil Corporation (JNOC: now the Japan Oil, Gas and Metals National Corporation, JOGMEC) (Qin et al., 2016); Pressure Core Tool with Ball Valve (PCTB) developed by Geotek. Because the core size of PTCS does not match the widely used Geotek’s Pressure Core Analysis and Transfer System (PCATS), JAMSTEC and AAI designed and manufactured the Hybrid Pressure Coring System (Hybrid PCS) using PTCS technology (Kubo et al., 2014; Yamamoto, 2015). Other drilling coring devices used in the field include the pressure and temperature preservation system (PTPS) developed by the China University of Petroleum (Beijing) and Southwest Petroleum University (Zhu et al., 2013), Wire-line pressure and temperature core system (WPTCS) developed by the Chinese Academy of Geoscience (Zhang et al., 2014), SUGAR corer (SUCO) in South Korea, and MeBo-Druckkern-Probennehmer (MDP) used in Nigeria Delta during the SUGAR 1 (Abid1 et al., 2015). See Table 7 for the comparison of their operation conditions (Kubo et al., 2014; Zhu et al., 2013; Zhang et al., 2014; Abid1 et al., 2015; Zhu et al., 2011; Bohrmann et al., 2007; Chen et al., 2013; Dickens et al., 2003; Inada and Yamamoto, 2015; Yang et al., 2017; Schultheiss et al., 2009; Ryu et al., 2013; Flemings et al., 2019). A glance at open sources indicates that FPC, HRC, Hybrid PCS, and PCTB are the main coring tools used in large hydrate drilling activities in the past decade. In 2010, the researchers recovered 25 pressure cores using FPC, FRC, and FRPC equipped on the D/V Fugro Synergy during UBGH2 (Ryu et al., 2013). In June 2012, MH21 used Hybrid PCS equipped on the D/V Chikyu to conduct pressure coring (Yamamoto, 2015). During the NGHP2 in 2015, 17 holes were drilled and/or cored with conventional coring tools (HPCS/ESCS) or pressure coring tools (PCTB) equipped on the D/V Chikyu (Kumar et al., 2019). During the GMGS5 in 2018, the conventional coring tools and PCTB on the Fugro Voyager drill vessel were used to coring at four drilling sites: W01, W07, W08, and W09 (Wei et al., 2019b). Here we can see that the driving force for the continued development of pressure coring technology comes largely from national programs.
Delivering high-quality, pressure-sealed, temperature-preserved gas hydrate samples is the tireless pursuit of exploration drilling. However, it is found that there are still many deficiencies in the coring tools after a rough comparison. Due to the limitations of the harsh environment in the drilling process, the use of the coring tools is restricted by the mechanical strength and tightness (Abid1 et al., 2015; Wang et al., 2015; Sun et al., 2015b). The most likely situation is that mechanical valves, sealing rings, and other seals cannot seal in-situ gas hydrate samples effectively, resulting in partial or complete loss of physical, chemical, and biological information. The pressure bearing range of the commercially used coring tools is 20–30 MPa and the maximum pressure does not exceed 70 MPa. The ice valves developed based on in-situ drilling fluid can improve the pressure-preserved performance of the valve to the level of 25 MPa or even higher 47 MPa (LuoPeng et al., 2015; Li et al., 2016b; Zhang et al., 2016). Although there have been some new designs that claim to increase the maximum bearing capacity of pressure corers above 100 MPa in recent years (Li et al., 2021c), they have not been applied in engineering. On the other hand, to reduce the influence of temperature change on the core, temperature preservation devices are added to the pressure-preserved drilling tools. This poses another world-class difficulty. That is, the key to the temperature preservation coring technology of gas hydrate is to prevent the temperature from rising, which is contrary to the previous temperature preservation technology. PCS, PCB, FPC, HRC, MAC, and DAPC do not have the function of temperature preservation, and the later developed PTCS, Hybrid PCS, PCTB, PTPS, WPTCS, and hole-bottom freezing sampling (HBFS) drilling tools (Sun et al., 2018; Guo et al., 2020) use vacuum insulation, thermal insulation material, cooling medium, thermoelectric-refrigeration technique, or external cooling device for temperature preservation. The heat preservation effect is limited. Higher core recovery needs to overcome pressure-related defects, and gas hydrate temperature-preservation coring techniques should be further developed, especially the active temperature-preservation techniques.
Conclusion
Drilling techniques are a series of practical technologies that combine empirical rules, qualitative judgment, and semi-quantitative interpretation. Successful, good, fast, and economical has always been the goal of drilling. However, with the development of oil and gas exploration and development of unconventional, low permeability, deep-water, and other complex oil and gas fields, drilling engineering is facing a series of problems and challenges in terms of safety, economy, and efficiency. Risks and challenges such as temperature and pressure fluctuations, drill string vibration, shallow water and gas flow, safe mud density window, wellhead/wellbore stability, flow assurance, methane leakage, etc. are still and will be difficult problems faced by hydrate drilling for a long time. Although the focus of this paper is to drill gas hydrate in the marine environment, especially in deep water, these situations also apply to drilling gas hydrate on land. The measures taken by the drilling project, whether on land or in the ocean, are to minimize the changes in the in-situ temperature and pressure of the reservoir to prevent hydrate dissociation. Thus, to avoid accidents such as wellbore instability and reservoir collapse. It is recommended to adopt the micro overbalance drilling method and develop drilling fluid with excellent performance to deal with the risk of drilling fluid invasion and hydrate dissociation in drilling activities.
Over the years, certain experience has been accumulated in scientific theory, technical equipment, engineering construction, environmental impact assessment in the field of gas hydrate. However, it is still necessary to develop advanced drilling technology and innovative drilling systems for use in the challenging conditions of drilling hydrate reservoirs. Through reasonable well layout, scientific well design, qualified casing and tubing, efficient drilling fluid, pressure-temperature preserved hydrate coring, successful completion, and sustainable development measures, methane can be produced safely and efficiently from hydrate reservoirs. Anyway, we must give full play to the advantages and characteristics of industry, universities, and research institutes. Multidisciplinary knowledge and the latest achievements in other fields should be comprehensively applied to overcome these difficulties. Only by deeply studying their mechanisms and internal laws can they be effectively solved. This requires proposing new scientific problems, establishing new theoretical viewpoints, developing new methods, new materials, new tools, and forming new technologies.
Author contributions
NW: Investigation, writing–original draft, review and editing, supervision, funding acquisition. JP: Investigation, methodology, writing - original draft, review and editing. JZ: Conceptualization, investigation, review. LZ: Investigation, methodology, visualization. SZ: Methodology, investigation, review. PL: Investigation, methodology, review. HL: Investigation. JW: Writing - editing. NW and JP contributed equally to this work and share first authorship.
Funding
This work was financially supported by the National Natural Science Foundation of China (U20B6005-05, 51874252), National Key Research and Development Program of China (2021YFC2800903), Sichuan High-end Foreign Talent Introduction Project (SYZ202124), High-end Foreign Experts Recruitment Plan of China (G2021036005L), 111 Project (D21025), and Open Fund of State Key Laboratory of Oil and Gas Reservoir Geology and Exploitation (Southwest Petroleum University) (PLN 2021-01, PLN 2021-02, PLN 2021-03).
Acknowledgments
The authors would like to thank the reviewers and editors for their helpful comments and suggestions.
Conflict of interest
The authors declare that the research was conducted in the absence of any commercial or financial relationships that could be construed as a potential conflict of interest.
Publisher’s note
All claims expressed in this article are solely those of the authors and do not necessarily represent those of their affiliated organizations, or those of the publisher, the editors and the reviewers. Any product that may be evaluated in this article, or claim that may be made by its manufacturer, is not guaranteed or endorsed by the publisher.
References
Abid1, K., Spagnoli, G., Teodoriu, C., and Falcone, G. (2015). Review of pressure coring systems for offshore gas hydrates research. Uw. Tech. Int. J. Soc. Uw. Tech. 33, 19–30. doi:10.3723/ut.33.019
Aird, P. (2019). Chapter 12 - deepwater “riserless” drilling, Editor(s): P. Aird, and D. Drilling, Oxford, United Kingdom: Gulf Professional Publishing, 441–475.
Amann, H., Hohnberg, H. J., and Reinelt, R. (1997). HYACE—A novel autoclave coring equipment for systematic offshore gashydrate sampling. Dtsch. Wiss. Ges. für Erdgas Kohle e.V. (DGMK) 9706, 37–49.
Balanza, J. A., Justiniano, L. C., and Poletzky, I. (2015). “Implementation of managed pressure casing drilling and managed pressure cementing techniques in unconventional reservoirs,” in SPE/IADC Drilling Conference and Exhibition, London, England, UK, March 17–19, 2015.
Bohrmann, G., Pape, T., and participants., C. (2007). Report and preliminary results of R/V METEOR cruise M72/3, istanbul–trabzon–istanbul. Marine gas hydrates of the eastern Black Sea. Berichte, Fachbereich Geowiss. Univ. Brem. Nr. 261, 176pp.
Boswell, R., Hancock, S., Yamamoto, K., Collett, T., Pratap, M., and Lee, S. R. (2020). Natural gas hydrates: Status of potential as an energy resource. Amsterdam, Netherlands: Elsevier, 111–131. Future Energy.
Boswell, R., Schoderbek, D., Collett, T. S., Ohtsuki, S., White, M., and Anderson, B. J. (2017). The iġnik Sikumi field experiment, Alaska North Slope: Design, operations, and implications for CO2–CH4 exchange in gas hydrate reservoirs. Energy fuels. 31 (1), 140–153. doi:10.1021/acs.energyfuels.6b01909
Briner, A., Busaidi, Y., Adawi, A., Hilditch, R., Sanchez, F., Sanderson, M., et al. (2015). “CwD technology improves economics in tight gas exploration project in the sultanate of Oman”. In: Paper presented at the Abu Dhabi International Petroleum Exhibition and Conference, November 9–12, 2015, Abu Dhabi, UAE.
Burger, J., Gupta, D., Jacobs, P., and Shillinglaw, J. (2006). Overview on hydrate coring, handling and analysis. DOE Report, DE-FC26–02NT41327.
Chen, G. M., Yin, Z. M., Xu, L. B., and Jiang, S. Q. (2007). Rev. Deep. dual gradient Drill. Technol. 34 (2), 246–252. (in Chinese with English Abstract).
Chen, J. W., Fan, W., Bingham, B., Chen, Y., Gu, L. Y., and Li, S. L. (2013). A long gravity-piston corer developed for seafloor gas hydrate coring utilizing an in situ pressure-retained method. Energies 6 (7), 3353–3372. doi:10.3390/en6073353
Chen, L., and Merey, S. (2021). Chapter 13 - emerging technologies in methane hydrate projects, Editor(s): L. Chen, and S. Merey, Oceanic methane hydrates, Oxford, United Kingdom: Gulf Professional Publishing, 349–390.
Chen, X. J., Lu, H. L., Gu, L. J., Shang, S. L., Zhang, Y., Huang, X., et al. (2022). Preliminary evaluation of the economic potential of the technologies for gas hydrate exploitation. Energy 243, 123007. doi:10.1016/j.energy.2021.123007
Chen, Y., Sun, B. J., Gao, Y. H., Liu, K., Li, H., and Zhao, X. X. (2019). Pressure effects on heat transfer in hydrate-bearing deposit with drilling fluid invasion by lab simulation. Int. J. Green Energy 16 (10), 770–777. doi:10.1080/15435075.2019.1641104
Cheng, W., Ning, F. L., Sun, J. X., Liu, Z. C., Jiang, G. S., and Li, X. D. (2019). A porothermoelastic wellbore stability model for riserless drilling through gas hydrate-bearing sediments in the Shenhu area of the South China Sea. J. Nat. Gas Sci. Eng. 72, 103036. doi:10.1016/j.jngse.2019.103036
Chibura, P. E., Zhang, W., Luo, A. J., and Wang, J. (2022). A review on gas hydrate production feasibility for permafrost and marine hydrates. J. Nat. Gas Sci. Eng. 100, 104441. doi:10.1016/j.jngse.2022.104441
Chong, Z. R., Yang, S. H. B., Babu, P., Linga, P., and Li, X. S. (2016). Review of natural gas hydrates as an energy resource: Prospects and challenges. Appl. Energy 162, 1633–1652. doi:10.1016/j.apenergy.2014.12.061
Chua, P. C., and Kelland, M. A. (2013). Study of the gas hydrate anti-agglomerant performance of a series of n-Alkyl-tri(n-butyl)ammonium bromides. Energy fuels. 27 (3), 1285–1292. doi:10.1021/ef3018546
Chuvilin, E., Ekimova, V., Davletshina, D., Sokolova, N., and Bukhanov, B. (2020). Evidence of gas emissions from permafrost in the Russian arctic. Geosciences 10 (10), 383. doi:10.3390/geosciences10100383
Colin, F., Ker, S., Riboulot, V., and Sultan, N. (2020). Irregular BSR: Evidence of an ongoing reequilibrium of a gas hydrate system. Geophys. Res. Lett. 47 (20), 1–10. doi:10.1029/2020gl089906
Collett, T., Riedel, M., Boswell, R., Presley, J., Kumar, P., Sathe, A., et al. (2015). U.S. Geological Survey Scientific Investigations Report 2012–5054[R]. Denver, CO: USGS. Indian national gas hydrate program expedition 01 report.
Crutchley, G. J., Pecher, I. A., Gorman, A. R., Henrys, S. A., and Greinert, J. (2010). Seismic imaging of gas conduits beneath seafloor seep sites in a shallow marine gas hydrate province, Hikurangi Margin, New Zealand. Mar. Geol. 272 (1), 114–126. doi:10.1016/j.margeo.2009.03.007
Dallimore, S. R., Collett, T. S., Weber, M., and Uchida, T. (2002). Drilling program investigates permafrost gas hydrates. Eos Trans. AGU. 83 (18), 193–198. doi:10.1029/2002eo000129
Das, S., Mahto, V., Udayabhanu, G., Lall, M., Singh, K., and Deepak, M. (2022). Experimental evaluation of Sarcosine as an eco-friendly green hydrate inhibitor for the drilling of gas hydrate bearing formations. J. Petroleum Sci. Eng. 208, 109764. doi:10.1016/j.petrol.2021.109764
Das, S., Mahto, V., Udayabhanu, G., Lall, M., Singh, K., and Deepak, M. (2022). Experimental evaluation of Sarcosine as an eco-friendly green hydrate inhibitor for the drilling of gas hydrate bearing formations. J. Petroleum Sci. Eng. 208, 109764. doi:10.1016/j.petrol.2021.109764
Day, R. W. (2006). Subsurface exploration. Fundation engineering handbook. New York, United States: McGraw-Hill.
Demirbas, A. (2010). Methane hydrates as potential energy resource: Part 2–methane production processes from gas hydrates. Energy Convers. Manag. 51 (7), 1562–1571. doi:10.1016/j.enconman.2010.02.014
Dickens, G. R., Schroeder, D., and Hinrichs, K. U.the Leg 201 Scientific Party (2003). “The pressure core sampler (PCS) on Ocean Drilling Program Leg 201: general operations and gas release,” in Proceedings ODP, initial reports 201. S. L. D’Hondt, B. B. Jørgensen, and D. J. Miller Editors et al. (College Station, TX: Ocean Drilling Program), 1–22.
Dickens, G. R., Wallace, P. J., Paull, C.K., and Borowski, W. S. (2000). Detection of methane gas hydrate in the pressure core sampler (PCS): Volume-pressure-time relations during controlled degassing experiments. Proc. Ocean. Drill. Progr. Sci. Results 164, 113–126.
Dong, H., and Zeng, G. (2017). Flammable ice' — Extract with caution. Nature 546, 599. doi:10.1038/546599a
Dong, L., Wan, Y. Z., Li, Y. L., Liao, H. L., Liu, C. L., Wu, N. Y., et al. (2022). 3D numerical simulation on drilling fluid invasion into natural gas hydrate reservoirs. Energy 241, 122932. doi:10.1016/j.energy.2021.122932
Fakher, S., Elgahawy, Y., and Abdelaal, H. (2019). “A comprehensive review on gas hydrate reservoirs: formation and dissociation thermodynamics and rock and fluid properties,” in International Petroleum Technology Conference, Beijing, China, March 26–28, 2019.
Fereidounpour, A., and Vatani, A. (2014). An investigation of interaction of drilling fluids with gas hydrates in drilling hydrate bearing sediments. J. Nat. Gas Sci. Eng. 20, 422–427. doi:10.1016/j.jngse.2014.07.006
Flemings, P., Phillips, S. C., Boswell, R., Collett, T. S., Cook, A. E., Dong, T., et al. (2019). Pressure coring a Gulf of Mexico deep-water turbidite gas hydrate reservoir: Initial results from the university of Texas–Gulf of Mexico 2-1 (UT-GOM2-1) hydrate pressure coring expedition. Am. Assoc. Pet. Geol. Bull. 104 (9), 1847–1876. doi:10.1306/05212019052
Foschi, M., Aanoni, M., Cartwright, J. A., and Idiz, E. (2019). Microbial vs thermogenic gas hydrates in the South falkland basin: BSR distribution and fluid origin. Mar. Petroleum Geol. 102, 695–703. doi:10.1016/j.marpetgeo.2019.01.023
Fu, Q., Wang, G. R., Zhou, S. W., Zhong, L., and Wang, L. Z. (2020). Development of marine natural gas hydrate mining technology and equipment. Chin. J. Eng. Sci. 22 (6), 32–39. (in Chinese with English Abstract). doi:10.15302/j-sscae-2020.06.005
Fugro Synergy, (2021) Fugro SYNERGY. Available at: https://media.fugro.com/media/docs/default-source/about-fugro-doc/vessels/fugro-synergy_a4_lr.pdf?sfvrsn=9cfa0c1a_26.
Gaafar, G. R., Tewari, R. D., and Zain, Z. M. (2015). “Overview of advancement in core analysis and its importance in reservoir characterisation for maximising recovery,” in SPE Asia Pacific Enhanced Oil Recovery Conference, Kuala Lumpur, Malaysia, August 11–13, 2015 (Kuala Lumpur, Malaysia: Society of Petroleum Engineers), 222–238.
Gao, K., Sun, Y. H., Ren, L. Q., Cao, P. L., Li, W. T., and Fan, H. K. (2018). Design and analysis of ternary coupling bionic bits. J. Bionic Eng. 5, 53–59. doi:10.1016/s1672-6529(08)60072-4
Gao, Y. H., Liang, J., Liu, H. S., Zhang, S. Y., Chen, L., and Chen, B.H. (2017). Analysis and research on gas hydrate drilling fluid application in Qilian Muli area, Qinghai Province. J. Qinghai Univ. Sci. 35 (3), 52–57+64. (in Chinese).
Gerivani, H., Putans, V. A., Merklin, L. R., and Modarres, M. H. (2020). Characteristics of features formed by gas hydrate and free gas in the continental slope and abyssal plain of the Middle Caspian Sea. Mar. Georesources Geotechnol. 39 (4), 419–430. doi:10.1080/1064119x.2019.1709585
Ghozatloo, A., Hosseini, M., and Shariaty-Niassar, M. (2015). Improvement and enhancement of natural gas hydrate formation process by Hummers’ graphene. J. Nat. Gas. Sci. Eng. 27, 1229–1233. doi:10.1016/j.jngse.2015.09.069
Graber, K. K., Pollard, E., Jonasson, B., and Schulte, E. (2021) Overview of Ocean Drilling program engineering tools and hardware. http://www-odp.tamu.edu/publications/tnotes/tn31/jr/jr.htm.
Graham, J. R., and Reed, J. A. (1969). Glomar challenger deep sea drilling vessel. J. Petroleum Technol. 21 (10), 1263–1274. doi:10.2118/2180-pa
Grigg, R. B., and Lynes, G. L. (1992). Oil-based drilling mud as a gas-hydrates inhibitor. SPE Drill. Eng. 7 (1), 32–38. doi:10.2118/19560-pa
Guo, W., Zhang, P. Y., Yang, X., Lei, J., Sun, Y. H., Rui, J., et al. (2020). Development and application of hole-bottom freezing drilling tool for gas-hydrate-bearing sediment sampling. Ocean. Eng. 203, 107195. doi:10.1016/j.oceaneng.2020.107195
Gupta, P., and Sangwai, J. S. (2019). formation and dissociation kinetics of methane hydrate in aqueous oilfield polymer solutions (polyacrylamide, xanthan gum, and guar gum) and their performance evaluation as low-dosage kinetic hydrate inhibitors (LDHI). Energy fuels. 33 (7), 6335–6349. doi:10.1021/acs.energyfuels.9b01204
Hancock, S., Boswell, R., and Collett, T. (2019). “Development of deepwater natural gas hydrates,” in Paper presented at the Offshore Technology Conference, Houston, Texas, May 6–9, 2019.
Hannegan, D. M. (2005). “Methane hydrate drilling technology,” in Paper presented at the Offshore Technology Conference, Houston, Texas, USA, May 2–5, 2005.
Hannegan, D. M., Todd, R. J., Pritchard, D. M., and Jonasson, B. (2005). “MPD-uniquely applicable to methane hydrate drilling,” in Paper presented at the SPE/IADC Underbalanced Technology Conference and Exhibition, Houston, Texas, USA, October 11–12, 2004.
He, Y. L., Liang, J. Q., Kuang, Z. G., Deng, W., Ren, J. F., Lai, H. F., et al. (2022). Migration and accumulation characteristics of natural gas hydrates in the uplifts and their slope zones in the Qiongdongnan Basin. China, China Geol. 5 (2), 234–250.
He, Y., Long, Z., Lu, J. S., Shi, L., Yan, W., and Liang, D. (2021). Investigation on methane hydrate formation in water-based drilling fluid. Energy fuels. 35 (6), 5264–5270. doi:10.1021/acs.energyfuels.0c04409
Heidari, H., Akbari, M., Souhankar, A., and Hafezi, R. (2022). Review of global energy trends towards 2040 and recommendations for Iran oil and gas sector. Int. J. Environ. Sci. Technol. (on line) 19, 8007–8018. doi:10.1007/s13762-022-03963-w
Heydari, A., and Peyvandi, K. (2020). Study of biosurfactant effects on methane recovery from gas hydrate by CO2 replacement and depressurization. Fuel 272, 117681. doi:10.1016/j.fuel.2020.117681
Hogenboom, S., Rokseth, B., Vinnem, J. E., and Utne, I. B. (2020). Human reliability and the impact of control function allocation in the design of dynamic positioning systems. Reliab. Eng. Syst. Saf. 194, 106340. doi:10.1016/j.ress.2018.12.019
Hou, Y., Liu, C. S., and Liu, D. (2022). Drilling fluid technology of horizontal well in shallow soft formation of natural gas hydrate in sea area, (in Chinese). Drill. Eng. 49 (2), 16–21.
Hsiung, K. H., Saito, S., Kanamatsu, T., Sanada, Y., and Yamada, Y. (2019). Regional stratigraphic framework and gas hydrate occurrence offshore eastern India: Core-log-seismic integration of National Gas Hydrate Program Expedition 02 (NGHP-02) Area-B drill sites. Mar. Petroleum Geol. 108, 206–215. doi:10.1016/j.marpetgeo.2018.06.021
Hu, C. Y., Yang, T. F., Burr, G. S., Chuang, P. C., Chen, H. W., Walia, M., et al. (2017). Biogeochemical cycles at the sulfate-methane transition zone (SMTZ) and geochemical characteristics of the pore fluids offshore southwestern Taiwan. J. Asian Earth Sci. 149, 172–183. doi:10.1016/j.jseaes.2017.07.002
Hu, G. W., Bu, Q. T., Lyu, W. J., Wang, J., Chen, J., Li, Q., et al. (2021). A comparative study on natural gas hydrate accumulation models at active and passive continental margins. Nat. Gas. Ind. B 8 (2), 115–127. doi:10.1016/j.ngib.2021.03.001
Hu, Y. Q., Xie, J., Xue, S. N., Xu, M., Fu, C. H., He, H. L., et al. (2022). Research and application of thermal insulation effect of natural gas hydrate freezing corer based on the wireline-coring principle. Petroleum Sci. 19 (3), 1291–1304. doi:10.1016/j.petsci.2021.11.019
Huang, T. J., Zhang, Y., Wang, Y., Li, X. S., and Chen, Z. Y. (2020). An experimental drilling apparatus used for evaluating drilling risks related to natural gas hydrate. MethodsX 7, 101019. doi:10.1016/j.mex.2020.101019
Inada, N., and Yamamoto, K. (2015). Data report: Hybrid Pressure Coring System tool review and summary of recovery result from gas-hydrate related coring in the Nankai Project. Mar. Petroleum Geol. 66, 323–345. doi:10.1016/j.marpetgeo.2015.02.023
Isaac, O. T., Pu, H., Oni, B. A., and Samson, F. A. (2022). Surfactants employed in conventional and unconventional reservoirs for enhanced oil recovery—a review. Energy Rep. 8, 2806–2830. doi:10.1016/j.egyr.2022.01.187
Jin, C. Y. (2021). Research report on technologies and equipment for exploitation of marine combustible ice resources. IOP Conf. Ser. Earth Environ. Sci. 647, 012117. doi:10.1088/1755-1315/647/1/012117
Jin, G. R., Peng, Y. Y., Liu, L. H., Su, Z., Liu, J., Li, T., et al. (2022). Enhancement of gas production from low-permeability hydrate by radially branched horizontal well: Shenhu Area, South China Sea. Energy 253, 124129. doi:10.1016/j.energy.2022.124129
John, J., Sin, G. H., Steve, M., and Jim, F. (2020). A case study of natural gas hydrates (NGH) in offshore NW Sabah: Identification, shallow geohazard implication for exploration drilling, extraction challenges and potential energy resource estimation. Bull. Geol. Soc. Malays. 70, 57–75. doi:10.7186/bgsm70202005
Kadaster, A. G., Millheim, K. K., and Thompson, T. W. (2005). “The planning and drilling of hot ice #1 - gas hydrate exploration well in the alaskan arctic,” in The SPE/IADC Drilling Conference, Amsterdam, Netherlands, February 23–25, 2005.
Ke, W., and Chen, D. Y. (2019). A short review on natural gas hydrate, kinetic hydrate inhibitors and inhibitor synergists. Chin. J. Chem. Eng. 27 (9), 2049–2061. doi:10.1016/j.cjche.2018.10.010
Kelland, M. A. (2006). History of the development of low dosage hydrate inhibitors. Energy fuels. 20 (3), 825–847. doi:10.1021/ef050427x
Khabibullin, T., Falcone, G., and Teodoriu, C. (2011). Drilling through gas-hydrate sediments: Managing wellbore-stability risks. SPE Drill. Complet. 26 (2), 287–294. doi:10.2118/131332-pa
Khabibullin, T., Falcone, G., and Teodoriu, C. (2011). Drilling through gas-hydrate sediments: Managing wellbore-stability risks. SPE Drill. Complet. 26 (02), 287–294. doi:10.2118/131332-pa
Kim, G. Y., Yi, B. Y., Kang, N. K., Yoo, D. G., and Lee, Yong, M. J. (2022). Comparison of logging-while-drilling and wireline logging data from gas hydrate-bearing deep-sea sediments, the Ulleung Basin, East Sea. Explor. Geophys. 53 (1), 92–103. doi:10.1080/08123985.2021.1898942
Kiran, B. S., and Prasad, P. S. R. (2021). Inhibition of methane hydrates using biodegradable additives. ACS Omega 6 (12), 8261–8270. doi:10.1021/acsomega.0c06328
Kubo, Y., Mizuguchi, Y., Inagaki, F., and Yamamoto, K. (2014). A new hybrid pressure-coring system for the drilling vessel <i>Chikyu</i>. Sci. Dril. 17 (17), 37–43. doi:10.5194/sd-17-37-2014
Kulkarni, N., and Heinze, L. (2022). “Assess the impact of shallow water flow geohazard on drilling operations in the riserless sections of deepwater well construction,” in Paper presented at the SPE Canadian Energy Technology Conference, Calgary, Alberta, Canada, March 16–17, 2022.
Kumar, P., Collett, T. S., Shukla, K. M., Yadav, U. S., Lall, M. V., and Vishwanath, K. (2019). India national gas hydrate program expedition-02: Operational and technical summary. Mar. Petroleum Geol. 108, 3–38. doi:10.1016/j.marpetgeo.2018.11.021
Kvamme, B., and Saeidi, N. (2021). A zero emission scheme for producing energy from natural gas hydrates and conventional natural gas. Petroleum 7 (4), 364–384. doi:10.1016/j.petlm.2021.10.003
Li, B., Li, X. S., Li, G., and Chen, Z. Y. (2015). Evaluation of gas production from Qilian Mountain permafrost hydrate deposits in two-spot horizontal well system. Cold Regions Sci. Technol. 109, 87–98. doi:10.1016/j.coldregions.2014.08.002
Li, C., Xie, H. P., Gao, M. Z., Chen, L., Zhao, L., Li, C. B., et al. (2021). Novel designs of pressure controllers to enhance the upper pressure limit for gas-hydrate-bearing sediment sampling. Energy 227, 120405. doi:10.1016/j.energy.2021.120405
Li, G., Li, X. S., Yang, B., Duan, L. P., Huang, N. S., Zhang, Y., et al. (2013). The use of dual horizontal wells in gas production from hydrate accumulations. Appl. Energy 112, 1303–1310. doi:10.1016/j.apenergy.2013.03.057
Li, G. S., Tian, S. C., and Zhang, Y. Q. (2020). Research progress on key technologies of natural gas hydrate exploitation by cavitation jet drilling of radial wells. Petroleum Sci. Bull. 5 (3), 349–365. (In Chinese with English abstract).
Li, H. T., Ge, Z. L., Wei, N., Sun, W. T., Zhang, Y., Xue, J., et al. (2021). Research on intelligent judgment method of natural gas hydrate drilling risk. Petroleum 7 (4), 439–450. doi:10.1016/j.petlm.2021.10.014
Li, H. Y., Zhang, M., Lau, H. C., and Fu, S. W. (2020). China's deepwater development: Subsurface challenges and opportunities. J. Petroleum Sci. Eng. 195, 107761. doi:10.1016/j.petrol.2020.107761
Li, J. F., Ye, J. L., Qin, X. W., Qiu, H. J., Wu, N. Y., Lu, H. L., et al. (2018). The first offshore natural gas hydrate production test in South China Sea. China Geol. 1 (1), 5–16. doi:10.31035/cg2018003
Li, L. J., Peng, J. M., Gao, Q., Sun, M, Z., Liu, Y., Li, M., et al. (2016). Pressure retaining method based on phase change for coring of gas hydrate-bearing sediments in offshore drilling. Appl. Therm. Eng. 107, 633–641. doi:10.1016/j.applthermaleng.2016.06.174
Li, L. L., Yang, J., Zhou, G. D., Yan, L., Yin, Q. S., Zhou, B., et al. (2018). “A new method based on simulation experiment to evaluate subsea wellhead stability of drilling from gas hydrate sediments in deep water,” in Paper presented at the Offshore Technology Conference, Houston, Texas, USA, April 30–May 3, 2018.
Li, L., Yang, J., Zou, X., Yan, L., Zhang, C. C., Yang, P. Y., et al. (2021) “Monitoring technology of gas hydrate in polar drilling,” in Paper presented at the The 31st International Ocean and Polar Engineering Conference, Rhodes, Greece, June 20–25, 2021.
Li, Q. M., Zhang, Y. Q., Yin, H., et al. (2017). Application of horizontal docking well drilling technology in gas hydrate test production. Explor. Eng. Soil Drill. Tunneling) 44 (8), 13–17. (in Chinese with English Abstract).
Li, W. L., Gao, D. L., and Yang, J. (2019). Challenges and prospects of well drilling and completion in Marine gas hydrate formation. Oil Drill. Prod. Technol. 41 (6), 681–689. (in Chinese).
Li, X. M., Zhang, Y. Q., Yin, H., Liang, J., Wang, H. B., et al. (2017). Application of drilling technology of horizontally butted well for gas hydrate trial-producing. Explor. Eng. Soil Drill. Tunneling 44 (8), 13–17. (in Chinese with English Abstract).
Li, X. S., Xu, C. G., Zhang, Y., Ruan, X. K., Li, G., and Wang, Y. (2016). Investigation into gas production from natural gas hydrate: A review. Appl. Energy 172, 286–322. doi:10.1016/j.apenergy.2016.03.101
Li, X. S., Zhang, Y., Li, G., Chen, Z. Y., and Wu, H. J. (2011). Experimental investigation into the production behavior of methane hydrate in porous sediment by depressurization with a novel three-dimensional cubic hydrate simulator. Energy fuels. 25 (10), 4497–4505. doi:10.1021/ef200757g
Liang, J. Q., Deng, W., Lu, J. A., Kuang, Z. G., He, Y. L., Zhang, W., et al. (2020). A fast identification method based on the typical geophysical differences between submarine shallow carbonates and hydrate bearing sediments in the northern South China Sea. China Geol. 3 (1), 16–27. doi:10.31035/cg2020021
Liang, J. Q., Zhang, W., Lu, J. A., Wei, J. G., Kuang, Z. G., and He, Y. L. (2019). Geological occurrence and accumulation mechanism of natural gas hydrates in the eastern Qiongdongnan Basin of the South China Sea: Insights from site GMGS5-W9-2018. Mar. Geol. 418, 106042. doi:10.1016/j.margeo.2019.106042
Liang, Q. Y., Xiao, X., Zhao, J., Zhang, W., Li, Y., Wu, X. M., et al. (2022). Geochemistry and sources of hydrate-bound gas in the Shenhu area, northern south China sea: Insights from drilling and gas hydrate production tests. J. Petroleum Sci. Eng. 208, 109459. doi:10.1016/j.petrol.2021.109459
Liang, T., Chen, Y. L., and Zhao, Y. (2021) “Introduction to the drilling system of “Chikyu” drilling vessel”,” in The 20th National Exploration Engineering (Geotechnical drilling engineering) academic Exchange conference, Xining, Qinghai, China.
Liu, J., Yang, R., Wu, D. D., and Jin, G. R. (2019). Estimation of microbial hydrate resources based on hydrocarbon generation material balance method:Case study of deep water area in southwestern Qiongdongnan Basin. Nat. Gas. Geosci. 30 (04), 539–548. (in Chinese with English Abstract).
Liu, S. Y., Li, H. Y., Wang, B., and Sun, B. J. (2022). Accelerating gas production of the depressurization-induced natural gas hydrate by electrical heating. J. Petroleum Sci. Eng. 208, 109735. doi:10.1016/j.petrol.2021.109735
Liu, T. L., Jiang, G. S., Zhang, P., Sun, J., Sun, H., Wang, R., et al. (2016). A new low-cost drilling fluid for drilling in natural gas hydrate-bearing sediments. J. Nat. Gas Sci. Eng. 33, 934–941. doi:10.1016/j.jngse.2016.06.017
Liu, X. F., Liu, C. H., and Wu, J. J. (2019). Dynamic characteristics of offshore natural gas hydrate dissociation by depressurization in marine sediments. Geofluids 2019, 1–11. doi:10.1155/2019/6074892
Liu, Y. M., Fang, M., Jiang, Y. F., Li, T. F., Yang, X. T., Wang, L. S., et al. (2021). Field test of scattered renewal well controlled pressure casing drilling and completion technology in the third mining block. West-China Explor. Eng. 33 (08), 43–46+49. (in Chinese).
Liu, Y. M., Yang, Z. G., Yang, J. S., Ma, X. W., Huo, D., and Geng, J. W. (2020). Research and field test of controlled pressure casing drilling technology. Drill. Prod. Technol. 43 (4), 1–3. (in Chinese).
Lunne, T., and Long, M. (2006). Review of long seabed samplers and criteria for new sampler design. Mar. Geol. 226, 145–165. doi:10.1016/j.margeo.2005.07.014
Luo, Y. J., Peng, J. M., Sun, M. Z., Sun, Q., Ji, T. K., et al. (2015). An ice-valve-based pressure-coring system for sampling natural hydrate-bearing sediments: Proof-of-concept laboratory studies. J. Nat. Gas Sci. Eng. 27, 1462–1469. doi:10.1016/j.jngse.2015.10.011
Macelloni, L., Lutken, C. B., Garg, S., Simonetti, A., D'Emidio, M., Wilson, R. M., et al. (2015). Heat-flow regimes and the hydrate stability zone of a transient, thermogenic, fault-controlled hydrate system (Woolsey Mound northern Gulf of Mexico). Mar. Petroleum Geol. 59, 491–504. doi:10.1016/j.marpetgeo.2014.09.010
Mahmood, M. N., and Guo, B. Y. (2021). Productivity comparison of radial lateral wells and horizontal snake wells applied to marine gas hydrate reservoir development. Petroleum 7 (4), 407–413. doi:10.1016/j.petlm.2021.10.004
Maiti, M., Bhaumik, A. K., and Mandal, A. (2021). Performance of water-based drilling fluids for deepwater and hydrate reservoirs: Designing and modelling studies. Petroleum Sci. 18 (6), 1709–1728. doi:10.1016/j.petsci.2021.09.001
Makogon, Y. F. (2010). Natural gas hydrates – a promising source of energy. J. Nat. Gas Sci. Eng. 2 (1), 49–59. doi:10.1016/j.jngse.2009.12.004
Matsuzawa, M., Terao, Y., Hay, B., Wingstrom, L., Duncan, M., and Ayling, I. (2014). “A completion system application for the world’s first marine hydrate production test,” in Paper presented at the Offshore Technology Conference, Houston, Texas, USA, May 5–8, 2014.
Merey, Ş., and Chen, L. (2022). Numerical comparison of different well configurations in the conditions of the 2020-gas hydrate production test in the Shenhu Area. Upstream Oil Gas Technol. 9, 100073. doi:10.1016/j.upstre.2022.100073
Merey, Ş. (2016). Drilling of gas hydrate reservoirs. J. Nat. Gas Sci. Eng. 35, 1167–1179. doi:10.1016/j.jngse.2016.09.058
Merey, Ş. (2019). Evaluation of drilling parameters in gas hydrate exploration wells. J. Petroleum Sci. Eng. 172, 855–877. doi:10.1016/j.petrol.2018.08.079
Minshull, T. A., Marín-Moreno, H., Betlem, P., Bialas, J., Buenz, S., Burwicz, E., et al. (2020). Hydrate occurrence in europe: A review of available evidence. Mar. Pet. Geol. 111, 735–764. doi:10.1016/j.marpetgeo.2019.08.014
Monteleone, V., Marín-Moreno, H., Bayrakci, G., Best, A., Shaon, F., Hossain, M. M., et al. (2022). Seismic characterization and modelling of the gas hydrate system in the northern Bay of Bengal, offshore Bangladesh. Mar. Petroleum Geol. 141, 105690. doi:10.1016/j.marpetgeo.2022.105690
Moridis, G. J., Reagan, M. T., and Zhang, K. (2008). “The use of horizontal wells in gas production from hydrate accumulations,” in Paper presented at the Proceedings of the 6th International Conference on Gas Hydrates, Vancouver, British Columbia, Canada.
Motghare, P. D., and Musale, A. (2017). “Unconventional hydrocarbons: Gas hydrates - drilling challenges and suitable technology,” in Paper presented at the SPE Oil and Gas India Conference and Exhibition, Mumbai, India, April 4–6, 2017.
Mozaffar, H., Anderson, R., and Tohidi, B. (2016). Reliable and repeatable evaluation of kinetic hydrate inhibitors using a method based on crystal growth inhibition. Energy fuels. 30 (12), 10055–10063. doi:10.1021/acs.energyfuels.6b00382
Nagappayya, S. K., Lucente-Schultz, R. M., Nace, V. M., and Ho, V. M. (2015). Antiagglomerant hydrate inhibitors: The link between hydrate-philic surfactant behavior and inhibition performance. J. Chem. Eng. Data 60 (2), 351–355. doi:10.1021/je500611d
Naser, G., Bin, G., and Sajjad, N. (2022). Evaluating the effect of new gas solubility and bubble point pressure models on PVT parameters and optimizing injected gas rate in gas-lift dual gradient. Drilling 15 (3), 1212.
Ngata, M. R., Yang, B. L., Aminu, M. D., Iddphonce, R., Omari, A., Shaame, M., et al. (2022). Review of developments in nanotechnology application for formation damage control. Energy fuels. 36 (180), 80. doi:10.1021/acs.energyfuels.1c03223
Ning, F. L., Chen, Q., Sun, J. X., Wu, X., Cui, G. D., Mao, P., et al. (2022). Enhanced gas production of silty clay hydrate reservoirs using multilateral wells and reservoir reformation techniques: Numerical simulations. Energy 254, 124220. (Part A). doi:10.1016/j.energy.2022.124220
Ning, F. L., Zhang, K. N., Wu, N. Y., Li, G., Jiang, G. S., et al. (2013). Invasion of drilling mud into gas-hydrate-bearing sediments. Part I: Effect of drilling mud properties. Geophys. J. Int. 193, 1370–1384. doi:10.1093/gji/ggt015
Ning, F. (2005). Research on wellbore stability in gas hydrate formation. China: China University of Geosciences.
Ning, F., Wu, N., Yu, Y., Zhang, K., Jiang, G., Zhang, L., et al. (2013). Invasion of drilling mud into gas-hydrate-bearing sediments. Part II: Effects of geophysical properties of sediments. Geophys. J. Int. 193, 1385–1398. doi:10.1093/gji/ggt016
Olga, G., Sergei, M., and Pavel, S. (2022). Key areas of gas hydrates study: Review. Energies 15 (5), 1799. doi:10.3390/en15051799
Pang, X. Q., Jia, C. Z., Chen, Z. X., Shi, H. S., Chen, Z. H., Hu, T., et al. (2022). Reduction of global natural gas hydrate (NGH) resource estimation and implications for the NGH development in the South China Sea. Petroleum Sci. 19 (1), 3–12. doi:10.1016/j.petsci.2021.12.006
Park, S. Y., Son, B. K., Choi, J., Jin, H., and Lee, K. (2022). Application of machine learning to quantification of mineral composition on gas hydrate-bearing sediments, Ulleung Basin, Korea. J. Petroleum Sci. Eng. 209, 109840. doi:10.1016/j.petrol.2021.109840
Pelley, J. (2008). Gas hydrates on the front burner: Flammable ice could create a bridge to a sustainable energy future. Environ. Sci. Technol. 42 (20), 7550–7551. doi:10.1021/es802250e
Peterson, M. N. A. (1984). Deep sea drilling project development engineering technical report note 16. La Jolla, CA: OSTI.GOV.Design and operation of a wireline pressure core barrel.
Pettigrew, T. L. (1992). Design and operation of a wireline pressure core sampler (PCS), Ocean drilling program technical note 17.
Prassl, W. F., Peden, J. M., and Wong, K. W. (2004). “Mitigating gas hydrate related drilling risks: A process-knowledge management approach,” in Paper presented at the SPE Asia Pacific Oil and Gas Conference and Exhibition, Perth, Australia, October 18–20, 2004.
Pui, G., Bhandari, J., Arzaghi, E., Abbassi, R., and Garaniya, V. (2017). Risk-based maintenance of offshore managed pressure drilling (MPD) operation. J. Petroleum Sci. Eng. 159, 513–521. doi:10.1016/j.petrol.2017.09.066
Qin, H. W., Cai, Z., Hu, H. M., Wang, J. J., Ye, W., and Chen, Y. (2016). Numerical analysis of gravity coring using coupled Eulerian-Lagrangian method and a new corer. Mar. Georesources Geotechnol. 34 (5), 403–408. doi:10.1080/1064119x.2014.958880
Rabinowitz, P. D., and Garrison, L. E. (1985). Operational and laboratory capabilities of "JOIDES resolution. College Station, TX: Texas A & M University.
Rana, A., Khan, I., and Saleh, T. A. (2021). Advances in carbon nanostructures and nanocellulose as additives for efficient drilling fluids: Trends and future perspective—a review. Energy fuels. 35 (9), 7319–7339. doi:10.1021/acs.energyfuels.0c04341
Ren, H., Han, L. J., Xu, J. L., and Wu, Z. H. (2013). “Research on marine gas hydrate drilling sampler of China,” in Paper presented at the tenth ISOPE ocean mining and gas hydrates symposium (Poland: Szczecin).
Roostaei, M., Javanmardi, J., Rasoolzadeh, A., and Mohammadi, A. H. (2021). Experimental determinations of the complete inhibition, the slow growth, and the rapid failure regions of methane hydrate formation in the presence of polyvinylpyrrolidone and polyvinylcaprolactam aqueous solutions. Energy fuels. 35 (5), 3780–3787. doi:10.1021/acs.energyfuels.0c03562
Roostaie, M., and Leonenko, Y. (2020). Analytical investigation of gas production from methane hydrates and the associated heat and mass transfer upon thermal stimulation employing a coaxial wellbore. Energy Convers. Manag. 209, 112616. doi:10.1016/j.enconman.2020.112616
Roostaie, M., and Leonenko, Y. (2020). Gas production from methane hydrates upon thermal stimulation, an analytical study employing radial coordinates. Energy 194, 116815. doi:10.1016/j.energy.2019.116815
Rosenberg, S., Kotow, K., Wakefield, J., Sampietro, S., and Hulett, B. (2022). “Riserless casing drilling for mitigation of shallow hazards - a paradigm shift in deepwater well construction,” in Paper presented at the IADC/SPE international drilling conference and exhibition (Galveston, Texas, USA).
Ruan, X. K., Li, X. S., and Xu, C. G. (2021). A review of numerical research on gas production from natural gas hydrates in China. J. Nat. Gas. Sci. Eng. 85, 103713. doi:10.1016/j.jngse.2020.103713
Ruan, X. K., Yang, M. J., Li, Y. H., Song, Y. C., and Liang, H. F. (2012). A review on the selection of extraction technologies for different forms of natural gas hydrate reservoirs. Nat. Gas Explor. Dev. 35 (2), 39–43+88. (in Chinese).
Ruppel, C. (2020). Map of gas hydrate locations . Available at: https://www.usgs.gov/media/images/map-gas-hydrate-locations-known-and-inferred.
Ryu, B. J., Collett, T. S., Riedel, M., Kim, G. Y., Chun, J., Bahk, J. J., et al. (2013). Scientific results of the second gas hydrate drilling expedition in the Ulleung basin (UBGH2). Mar. Petroleum Geol. 47, 1–20. doi:10.1016/j.marpetgeo.2013.07.007
Sa, J. H., Lee, B. R., Park, D. H., Han, K., Chun, H. D., and Lee, K. H. (2011). Amino acids as natural inhibitors for hydrate formation in CO2 sequestration. Environ. Sci. Technol. 45 (13), 5885–5891. doi:10.1021/es200552c
Sahu, C., Kumar, R., and Sangwai, J. S. (2020). Comprehensive review on exploration and drilling techniques for natural gas hydrate reservoirs. Energy fuels. 34 (10), 11813–11839. doi:10.1021/acs.energyfuels.0c02202
Sain, K., and Gupta, H. (2012). Gas hydrates in India: Potential and development. Gondwana Res. 22 (2), 645–657. doi:10.1016/j.gr.2012.01.007
Sánchez, F., and Al-Harthy, M. H. (2011). Risk analysis: Casing-while-Drilling (CwD) and modeling approach. J. Petroleum Sci. Eng. 78 (1), 1–5. doi:10.1016/j.petrol.2011.04.017
Saumya, S., Narasimhan, B., Singh, J., Yamamoto, H., Vij, J., Sakiyama, N., et al. (2019). Acquisition of logging-while-drilling (LWD) multipole acoustic log data during the India national gas hydrate program (NGHP) expedition 02. Mar. Petroleum Geol. 108, 562–569. doi:10.1016/j.marpetgeo.2018.10.011
Schoderbek, D., Farrell, H., Hester, K., Howard, J., Raterman, K., Silpngarmlert, S., et al. (2013). ConocoPhillips gas hydrate production test final technical report. Houston, TX: ConocoPhillips Co.
Schultheiss, P., Holland, M., and Humphrey, G. (2009). Wireline coring and analysis under pressure: Recent use and future developments of the HYACINTH system. Sci. Dril. 7 (7), 44–50. doi:10.5194/sd-7-44-2009
Schultheiss, P., Holland, M., and Roberts, J. (2008). “Pressure core analysis: The keystone of a gas hydrate investigation,” in Proceedings of the 6th International Conference on Gas Hydrates Vancouver, British Columbia, July 6-10, 2008.
Singh, R. P., Lall, D., and Vishal, V. (2022). Prospects and challenges in unlocking natural-gas-hydrate energy in India: Recent advancements. Mar. Petroleum Geol. 135, 105397. doi:10.1016/j.marpetgeo.2021.105397
Song, Y., Yang, L., Zhao, J., Liu, W., Yang, M., Li, Y., et al. (2014). The status of natural gas hydrate research in China: A review. Renew. Sustain. Energy Rev. 31, 778–791. doi:10.1016/j.rser.2013.12.025
Su, P. B., Liang, J. Q., Zhao, Q. X., Lu, J. A., Su, X., and Yang, T. (2020). Exploration technology of natural gas hydrate resources in sea area. Beijing: Science Press.
Sun, W. T., Pei, J., Wei, N., Zhao, J. Z., Xue, J., Zhou, S. W., et al. (2021). Sensitivity analysis of reservoir risk in marine gas hydrate drilling. Petroleum 7 (4), 427–438. doi:10.1016/j.petlm.2021.10.013
Sun, W. T., Wei, N., Zhao, J. Z., Kvamme, B., Zhou, S. W., Zhang, L. H., et al. (2022). Imitating possible consequences of drilling through marine hydrate reservoir. Energy 239, 121802. doi:10.1016/j.energy.2021.121802
Sun, Y., Goldberg, D., Collett, T., and Hunter, R. (2011). High-resolution well-log derived dielectric properties of gas-hydrate-bearing sediments, Mount Elbert gas hydrate stratigraphic test well, Alaska north slope. Mar. Pet. Geol. 28, 450–459. doi:10.1016/j.marpetgeo.2010.03.001
Sun, Y. H., Ma, Y. L., Huang, S. H., Gao, K., Liu, B. C., Li, X. Y., et al. (2012). Development and application of bionic coupling impregnated diamond bit for gas hydrate exploration. J. Jilin Unviersity Earth Sci. Ed. 42 (S3), 295–300. (in Chinese with English Abstract).
Sun, Y. H., Wang, Y., Guo, W., Jia, R., Chen, G., and Zhang, P. (2018). Hole-bottom freezing technique based on phase change heat transfer for gas-hydrates sampling: Efficiency optimization of refrigeration change of phase. Appl. Therm. Eng. 130, 722–734. doi:10.1016/j.applthermaleng.2017.11.012
Sun, Y. H., Wang, Y., Lü, X. S., Jia, R., and Guo, W. (2015). Hole-bottom freezing method for gas hydrate sampling. J. Nat. Gas Sci. Eng. 25, 271–283. doi:10.1016/j.jngse.2015.05.011
Sun, Y., Wang, Y., Lü, X., Jia, R., and Guo, W. (2015). Hole-bottom freezing method for gas hydrate sampling. J. Nat. Gas. Sci. Eng. 25, 271–283. doi:10.1016/j.jngse.2015.05.011
Sung, W., Lee, H., and Lee, C. (2002). Numerical study for production performances of a methane hydrate reservoir stimulated by inhibitor injection. Energy sources. 24 (6), 499–512. doi:10.1080/00908310290086527
Teymouri, M., Sanchez, M., and Santamarina, J. C. (2020). A pseudo-kinetic model to simulate phase changes in gas hydrate bearing sediments. Mar. Pet. Geol. 120, 104519. doi:10.1016/j.marpetgeo.2020.104519
Thakur, N. K. (2010). Gas hydrates as alternative energy resource - seismic methods. Curr. Sci. 99 (2), 181–189.
Todd, R. J., Hannegan, D. M., and Harrall, S. (2006). “New technology needs for methane hydrates production,” in Paper presented at the Offshore Technology Conference, Houston, Texas, USA, May 1–4, 2006.
Tommasi, P., Avalle, A., Budillon, F., Romeo, R., Caburlotto, A., Conforti, A., et al. (2019). Evaluation of disturbance induced on soft offshore sediments by two types of gravity piston coring techniques. Mar. Geol. 417, 106005. doi:10.1016/j.margeo.2019.106005
Uchida, T., Ikeda, I. Y., Takeya, S., Kamata, Y., Ohmura, R., Nagao, J., et al. (2005). Kinetics and stability of CH4–CO2 mixed gas hydrates during formation and long-term storage. ChemPhysChem 6 (4), 646–654. doi:10.1002/cphc.200400364
Vedachalam, N., Srinivasalu, S., Rajendran, G., Ramadass, G. A., and Atmanand, M. A. (2015). Review of unconventional hydrocarbon resources in major energy consuming countries and efforts in realizing natural gas hydrates as a future source of energy. J. Nat. Gas Sci. Eng. 26, 163–175. doi:10.1016/j.jngse.2015.06.008
Vrielink, H. J., Bradford, J. S., Basarab, L., and Ubaru, C. C. (2008). “Successful application of Casing-While-Drilling technology in a Canadian Arctic permafrost application,” in Paper presented at the IADC/SPE Drilling Conference, Orlando, Florida, USA, March 4–6, 2008.
Wang, C., Liu, Y. P., Hou, W., Liu, C., Zheng, Y. Y., and Wang, G. R. (2020). “Dynamic risk analysis on offshore natural gas hydrate production test based on DBN-GO method,” in Paper presented at the The 30th International Ocean and Polar Engineering Conference, Virtual, October 11–16, 2020.
Wang, G. R., Huang, R., Zhong, L., Wang, L, Z., Zhou, S, W., and Liu, Q. Y. (2019). An optimal design of crushing parameters of marine gas hydrate reservoirs in solid fluidization exploitation. Nat. Gas. Ind. B 6 (3), 257–261. doi:10.1016/j.ngib.2018.10.006
Wang, G. R., Zhong, L., Liu, Q. Y., and Zhou, S. W. (2019). Research on deep-sea oil and gas hydrate exploitation technology based on double gradient double tube. Ocean Eng. Equip. Technol. 201, 225–233.
Wang, L., Li, Y., Wu, P., Shen, S., Liu, T., Leng, S., et al. (2020). Physical and mechanical properties of the overburden layer on gas hydrate-bearing sediments of the South China sea. J. Pet. Sci. Eng. 189, 107020. doi:10.1016/j.petrol.2020.107020
Wang, L. Z., Wang, G. R., Mao, L. J., Fu, Q., and Zhong, L. (2020). Experimental research on the breaking effect of natural gas hydrate sediment for water jet and engineering applications. J. Petroleum Sci. Eng. 184, 106553. doi:10.1016/j.petrol.2019.106553
Wang, S., Zhang, C., and Yuan, C. P. (2017). Rheological properties of polymer drilling fluid developed for permafrost natural gas hydrate drilling[J]. Chem. Technol. Fuels Oils 53 (2), 274–285.
Wang, Y. B., and Gao, D. L. (2022). Study on the marine environment limiting conditions of deepwater drilling for natural gas hydrate. Appl. Energy 312, 118802. doi:10.1016/j.apenergy.2022.118802
Wang, Y., Sun, Y. H., Lv, X. S., Jia, R., and Guo, W. (2015). Hole-bottom freezing method for gas hydrate sampling. J. Nat. Gas. Sci. Eng. 25, 271–283. doi:10.1016/j.jngse.2015.05.011
Wang, Z. Z., Gao, K., Sun, Y. H., Zhang, Z. H., Zhang, S. Y., Liang, Y., et al. (2016). Effects of bionic units in different scales on the wear behavior of bionic impregnated diamond bits. J. Bionic Eng. 13 (4), 659–668. doi:10.1016/s1672-6529(16)60337-2
Wei, J. G., Liang, J. Q., Lu, J. G., Zhang, W., and He, Y. L. (2019). Characteristics and dynamics of gas hydrate systems in the northwestern South China Sea - results of the fifth gas hydrate drilling expedition. Mar. Petroleum Geol. 110, 287–298. doi:10.1016/j.marpetgeo.2019.07.028
Wei, N., Zhao, J. Z., Sun, W. T., Zhou, S. W., Zhang, L. H., Li, Q. P., et al. (2019). Non-equilibrium multiphase wellbore flow characteristics in solid fluidization exploitation of marine gas hydrate reservoirs. Nat. Gas. Ind. B 6 (3), 282–292. doi:10.1016/j.ngib.2018.10.008
Wei, W. N., Li, B., Gan, Q., and Li, Y. L. (2022). Research progress of natural gas hydrate exploitation with CO2 replacement: A review. Fuel 312, 122873. doi:10.1016/j.fuel.2021.122873
Winters, W. J., Dugan, B., and Collett, T. S. (2008). Physical properties of sediments from Keathley Canyon and Atwater Valley, JIP Gulf of Mexico gas hydrate drilling program. Mar. Petroleum Geol. 25 (9), 896–905. doi:10.1016/j.marpetgeo.2008.01.018
Wu, N. Y., Li, Y. L., Wan, Y. Z., Sun, J. Y., Huang, L., and Mao, P. X. (2021). Prospect of marine natural gas hydrate stimulation theory and technology system. Nat. Gas. Ind. B 8 (2), 173–187. doi:10.1016/j.ngib.2020.08.003
Xie, H. P., Liu, T., Gao, M. Z., Chen, L., Zhou, H. W., Ju, Y., et al. (2016). Research on in-situ condition preserved coring and testing systems. Petroleum Sci. 18 (6), 1840–1859. doi:10.1016/j.petsci.2021.11.003
Xu, Z., Hu, T., Pang, X. Q., Wang, E. Z., Liu, X. H., Wu, Z. Y., et al. (2022). Research progress and challenges of natural gas hydrate resource evaluation in the South China Sea. Petroleum Sci. 19 (1), 13–25. doi:10.1016/j.petsci.2021.12.007
Yamamoto, K. (2015). Overview and introduction: Pressure core-sampling and analyses in the 2012–2013 MH21 offshore test of gas production from methane hydrates in the eastern Nankai Trough. Mar. Petroleum Geol. 66, 296–309. doi:10.1016/j.marpetgeo.2015.02.024
Yamamoto, K., Terao, Y., Fujii, T., Ikawa, T., Seki, M., Matsuzawa, M., et al. (2014). “Operational overview of the first offshore production test of methane hydrates in the Eastern Nankai Trough,” in Paper presented at the Offshore Technology ConferenceMay 5–8, 2014, Houston, Texas, USA.
Yamamoto, K., Wang, X. X., Tamaki, M., and Suzuki, K. (2019). The second offshore production of methane hydrate in the Nankai Trough and gas production behavior from a heterogeneous methane hydrate reservoir. RSC Adv. 9 (45), 25987–26013. doi:10.1039/c9ra00755e
Yang, M. J., Gao, Y., Zhou, H., Chen, B. B., and Li, Y. H. (2019). Gas production from different classes of methane hydrate deposits by the depressurization method. Int. J. Energy Res. 43 (10), 5493–5505. doi:10.1002/er.4669
Yang, S., Liang, J., Lei, Y., Gong, Y., Xu, H., Wang, H., et al. (2017). GMGS4 gas hydrate drilling expedition in the South China Sea. Fire Ice Methane Hydrate Newsl. 17, 1–5.
Ye, H. Y., Wu, X. Z., and Li, D. Y. (2021). Numerical simulation of natural gas hydrate exploitation in complex structure wells: Productivity improvement analysis. Mathematics 9 (18), 2184. doi:10.3390/math9182184
Ye, J. L., Qin, X. W., Xie, W. W., Lu, H. l., Ma, B. j., Qiu, H. j., et al. (2020). The second natural gas hydrate production test in the South China Sea. China Geol. 3 (2), 197–209. doi:10.31035/cg2020043
Yevgeny, S., Volodymyr, K., and Valentina, Y. (2021). Mud-volcanic deposits of methane gas hydrates” in the Black Sea. E3S Web Conf. 230, 01005. doi:10.1051/e3sconf/202123001005
Yu, T., Guan, G. Q., Abudula, A., Wang, D. Y., and Song, Y. C. (2021). Numerical evaluation of free gas accumulation behavior in a reservoir during methane hydrate production using a multiple-well system. Energy 218, 119560. doi:10.1016/j.energy.2020.119560
Yuha, Y. B. M., Lal, B., Bavoh, C. B., and Keong, L. K. (2021). A comparison study on the performance between Tetrametylammonium chloride and PolyvinylPyrrolidone as drilling mud additives for gas hydrates. Mater. Today Proc. 47 (6), 1258–1262. doi:10.1016/j.matpr.2021.02.791
Zamora-Ledezma, C., Narváez-Muñoz, C., Guerrero, V. H., Medina, E., and Meseguer-Olmo, L. (2022). Nanofluid formulations based on two-dimensional nanoparticles, their performance, and potential application as water-based drilling fluids. ACS Omega 7 (24), 20457–20476. doi:10.1021/acsomega.2c02082
Zeng, Z. W., Zhu, H. T., Yang, X. H., and Cao, X. R. (2022). Three-dimensional seismic analysis of a polygonal fault system (PFS) in the Northern Carnarvon Basin, Australia: Implications for fluid flow migration and gas hydrate system. J. Petroleum Sci. Eng. 215, 110602. doi:10.1016/j.petrol.2022.110602
Zhang, J. H., Fan, B., and Liu, R. J. (2020). Current situation and drilling technology of gas hydrate drilling. Sci. Technol. Eng. 20 (35), 14343–14351. (in Chinese).
Zhang, L., Feng, R., Geng, S. H., Meng, M. M., Li, X., Ren, B., et al. (2021). Influence of geological structures on hydrate accumulation in subsea shallow formations. J. Nat. Gas Sci. Eng. 90, 103898. doi:10.1016/j.jngse.2021.103898
Zhang, P. P., Zhang, Y. Q., Zhang, W. H., and Tian, S. C. (2022). Numerical simulation of gas production from natural gas hydrate deposits with multi-branch wells: Influence of reservoir properties. Energy 238, 121738. (Part A). doi:10.1016/j.energy.2021.121738
Zhang, X. X., Peng, J. M., Sun, M. Z., Gao, Q., and Wu, D. Y. (2016). Development of applicable ice valves for ice-valve-based pressure corer employed in offshore pressure coring of gas hydrate-bearing sediments. Chem. Eng. Res. Des. 111, 117–126. doi:10.1016/j.cherd.2016.05.001
Zhang, Y. B., Qiu, Z. S., Zhao, X., Zhong, H., Huang, W., and Mu, J. (2021). Experimental study on ultra-low temperature drilling fluid in Arctic permafrost. Polar Sci. 28, 100645. doi:10.1016/j.polar.2021.100645
Zhang, Y. Q., Liang, J., Li, K., Wang, H. B., Li, X. M., and Wu, J. X. (2014). Research progress of drilling coring and mining on the permafrost natural gas hydrates in China. Procedia Eng. 73, 362–367. doi:10.1016/j.proeng.2014.06.210
Zhang, Y. Q., Zhang, L. X., and Wang, H. B. (2015). “The reverse circulation (RC) drilling techniques for the mineral resources exploration and production in ocean,” in Paper presented at the eleventh ocean mining and gas hydrates symposium (Hawaii, USA: Kona). Paper Number: ISOPE-M-15-207.
Zhang, Y. Q., and Zhu, Y. H. (2011). Research and application of the natural gas hydrate drilling technique in Qilian Mountain permafrost. Geol. Bull. China 30 (12), 1904–1909. (in Chinese with English Abstract).
Zhao, X., Qiu, Z. S., Zhao, C., Xu, J., and Zhang, Y. (2019). Inhibitory effect of water-based drilling fluid on methane hydrate dissociation. Chem. Eng. Sci. 199, 113–122. doi:10.1016/j.ces.2018.12.057
Zhong, G. F., Zhang, D., and Zhao, L. X. (2021). Current states of well-logging evaluation of deep-sea gas hydrate-bearing sediments by the international scientific ocean drilling (DSDP/ODP/IODP) programs. Nat. Gas. Ind. B 8 (2), 128–145. doi:10.1016/j.ngib.2020.08.001
Zhou, S. W., Li, Q. P., Chen, W., Zhou, J. L., Wei, N., Guo, P., et al. (2018). Optimal design of the engineering parameters for the first global trial production of marine natural gas hydrates through solid fluidization. Nat. Gas. Ind. B 5 (2), 118–131. doi:10.1016/j.ngib.2018.01.004
Zhou, S. W., Li, Q. P., Lv, X., Fu, Q., and Zhu, J. (2022). Key issues in development of offshore natural gas hydrate. Front. Energy 14 (3), 433–442. doi:10.1007/s11708-020-0684-1
Zhu, H. Y., Liu, Q. Y., Deng, J. E., Wang, G. R., Xiao, X. H., Jiang, Z. L., et al. (2011). Pressure and temperature preservation techniques for gas-hydrate-bearing sediments sampling. Energy 36 (7), 4542–4551. doi:10.1016/j.energy.2011.03.053
Zhu, H. Y., Liu, Q. Y., Wang, G. R., Xiao, X. H., Zhu, X. H., Jiang, Z. L., et al. (2013). A pressure and temperature preservation system for gas-hydrate-bearing sediments sampler. Petroleum Sci. Technol. 31 (6), 652–662. doi:10.1080/10916466.2010.531352
Zhu, Y. H., Zhang, Y. Q., Fang, H., Lu, Z. Q., Pang, S. J., Zhang, S., et al. (2020). Research progress of natural gas hydrate in China. Geol. Surv. China 7 (04), 1–9. (in Chinese).
Keywords: gas hydrate, hydrate reservoir, drilling technique, drilling fluid, wellbore structure, drilling equipment, pressure coring technique, challenge
Citation: Wei N, Pei J, Zhao J, Zhang L, Zhou S, Luo P, Li H and Wu J (2022) A state-of-the-art review and prospect of gas hydrate reservoir drilling techniques. Front. Earth Sci. 10:997337. doi: 10.3389/feart.2022.997337
Received: 18 July 2022; Accepted: 05 September 2022;
Published: 27 September 2022.
Edited by:
Zhifeng Wan, Sun Yat-sen University, ChinaReviewed by:
Ren Wang, CNPC Engineering Technology R & D Company Limited, ChinaJiafei Zhao, Dalian University of Technology, China
Copyright © 2022 Wei, Pei, Zhao, Zhang, Zhou, Luo, Li and Wu. This is an open-access article distributed under the terms of the Creative Commons Attribution License (CC BY). The use, distribution or reproduction in other forums is permitted, provided the original author(s) and the copyright owner(s) are credited and that the original publication in this journal is cited, in accordance with accepted academic practice. No use, distribution or reproduction is permitted which does not comply with these terms.
*Correspondence: Na Wei, d2VpbmE4MDgxQDE2My5jb20=; Jun Pei, YXNoYXJleUB5ZWFoLm5ldA==