- 1School of Geosciences and Engineering, Hebei University of Engineering, Handan, China
- 2Institute of Mountain Hazards and Environment, CAS, Chengdu, China
To study the mechanical effect of clay under acidic and basic conditions, typical clay minerals, montmorillonite and illite, were taken as the main research objects in this study. The variation law and mechanism of the cohesive force and internal friction angle were studied by immersing the remoulded soil in HNO3 solution with pH = 3 and NaOH (alkaline waste liquid) with pH = 13.5, respectively. It was found that, under acidic conditions, a corrosion reaction between clay minerals and nitric acid occurred. Except for the medium-term, the cohesion generally shows a decreasing trend, and the internal friction angle has little change. Under alkaline conditions, the cohesion of montmorillonite-quartz sand remoulded soil decreased briefly in the early immersion stage of and increased in the middle and late stages. The internal friction angle increases steadily with the extension of immersion time. The cohesion of illite-quartz sand remoulded soil also decreased first and then increased, while the internal friction angle changed little. X-ray diffraction analysis shows that montmorillonite and illite will corrode under acidic conditions, and no new material will be generated, resulting in a decrease in soil cohesion. Under alkaline conditions, montmorillonite was seriously depleted, resulting in the formation of zeolite minerals (zeolite X, garronite) and new cement hydrated calcium silicate CSH (xonotlite). Strong alkali reacts with illite to generate sodium metaaluminate (NaAlO2) and liquid cement Na2SiO3 (sodium silicate). The formation of new cements is the main reason for the increase in cohesion under acid-base conditions, and chemical corrosion and ion exchange cause a decrease in cohesion.
1 Introduction
Water is the key factor in soil landslides. Statistics show that water is the main inducing factor for more than 90% of landslide events (Yan et al., 2013; Xu and Liu, 2019; Zhu and Zhang, 2019). The chemical interaction between water and soil plays an important role in the breeding process of soil landslides. Especially in recent years, environmental problems such as acid rain and alkaline waste discharge caused by the rapid development of industry and agriculture have occurred frequently. These chemically active aqueous solutions contact the soil, which will inevitably have a water‒soil chemical interaction and a profound impact on the mechanical properties of the soil.
The influence of soil‒water chemistry on soil mechanical properties is long-term and complex. As early as the 1980s, Gu (1981; 1984; 1984; 1988) carried out related research on the uneven settlement of an alkali recovery workshop in a paper mill in Fujian and the erosion hole of a wall foundation in a glucose workshop in Shanghai. Through field sampling, immersion tests and indoor analysis and identification, it was confirmed that the decrease in soil strength caused by acid and alkali waste liquid erosion was the main reason for the above events. Subsequently, the soil‒water chemical mechanical effect quickly attracted the attention of researchers, and a series of related experimental studies were carried out to explore the trends of changes in the properties of soil under different aqueous chemical environments. For example, Tiwari et al., 2005 studied the effect of pore water salinity on the residual shear strength of soil and found that high concentrations of sodium chloride and calcium chloride aqueous solution had an obvious weakening effect on the strength of soil. Zhao et al. (2007; 2009; 2011) analysed the mineral composition and structure of the sliding surface and studied the influence of soil shear strength variation on landslide formation under acid rain through laboratory tests. Wang et al. (2010) took the subgrade of the Wuhan-Guangzhou high-speed railway as the test background, tested water samples and soil samples at different mileages, analysed the influence of solution chemical properties on the shear strength parameters of soil, and found that ion mass concentration had a strong correlation with cohesion. Gratchev and Towhata (2010; 2013) studied the effect of acid solution on undisturbed soil. The cement calcium carbonate (CaCO3) in the soil corroded in acid, resulting in a decrease in soil cohesion and an increase in compressibility. Yu et al. (2019) also used different concentrations of NaCl solution to soak clay remoulded soil samples and obtained similar results. He et al. (2020) explored the influence of water‒soil chemical processes on the stability of loess slope landslides under the action of acid rain. The leaching test showed that, under acidic conditions, H+ promoted the corrosion of carbonate rocks and made the soil stability worse. In addition, some researchers conducted a series of studies on slope stability under the effect of rainfall by obtaining landslide deformation data and established a coupling model of soil‒water interactions and landslide deformation, which provided a basis for predicting natural disasters such as landslides induced by rainfall infiltration (Ma et al., 2021; Liu et al., 2022; Yang et al., 2022; Zhou et al., 2022). In summary, although previous studies have carried out a great deal of work on the influence of water‒soil chemical interactions on soil strength, it is difficult to clarify the mechanism of the water‒soil chemical mechanical effect due to the complexity of the soil mineral composition. As the main secondary mineral, clay minerals have complex physical and chemical interactions with water, which have a great influence on the mechanical properties of soil. However, few people have carried out systematic studies on the mechanical effect and mechanism of clay under water‒soil chemical interactions.
Cohesion and internal friction angle are important indicators for evaluating the stability of soil slopes. To explore the water‒soil chemical mechanical effect of clay, in this paper, the typical clay minerals montmorillonite and illite were taken as the cement to make up montmorillonite-quartz sand remoulded soil and illite-quartz sand remoulded soil. The variation laws and mechanisms of cohesion and internal friction angle of soil under the action of acid rain and alkaline waste liquid were systematically studied, which not only provide a theoretical basis for revealing the breeding mechanism of soil landslides but also have important practical significance for the early warning and prevention of soil landslides.
2 Materials and methods
2.1 Test materials
In this study, the basic parameters of montmorillonite and illite samples are selected as shown in Table 1. The purity of the montmorillonite sample was 97%, and the chemical formula was Ca0.2(Al,Mg)2SiO10(OH)2.4H2O, belonging to calcium montmorillonite. Due to the difficulty in the purification of illite, the purity of illite samples used in this study was low, 88.46%. In addition to illite, the samples also contained impurities such as quartz and mica. By X-ray diffraction (XRD) analysis, the chemical formula of illite in the sample was (K,H3O)Al2Si3AlO10(OH)2, which is a potassium-based illite. The average particle size of the quartz sand used in the experiment is approximately 192.59 μm, the silicon content is more than 99.5%, and the hardness is 7.8, which meets the experimental requirements.
In terms of aqueous solution, concentrated nitric acid (mass fraction: 66.5%) and NaOH (mass fraction: 96%) of analytical purity were used in this study. HNO3 solution with pH = 3 and NaOH solution with pH = 13.5 were prepared to simulate acid rain and alkaline waste liquid, respectively. The mechanical parameters of the remoulded soil and the physical-chemical parameters of the solution under the action of acid and alkali were studied through immersion tests.
2.2 Experimental design
In this study, the density of the remoulded soil is 2.0 g/cm3 (clay ρ = 1.8–2.0 g/cm3 (Gong et al., 1996)), and the clay mineral content is 10% (when the clay mineral content reaches 5%, the strength of the soil can be significantly affected (Yong et al., 1984), and 10% content should be sufficient to reflect its mechanical effect). The preparation process of the remoulded soil was as follows: clay minerals (montmorillonite or illite) and quartz sand were fully mixed on the test bench first, a certain amount of chemical solution (HNO3 solution at pH = 3 or NaOH solution at pH = 13.5) was added, and the mixture was fully mixed again. After reaching the control moisture content (17.65%) throughout the sample, 120 g of wet soil sample was weighed and compacted in the 60 cm3 cutting ring (Φ61.8 × 20 mm). Five remoulded soil samples were placed in a 2 L large-calibre beaker with a cutting ring (five remoulded soil samples were Group 1). The soil samples were separated by permeable stone, injected with 1.8 L of the corresponding chemical solution and sealed with fresh-keeping film. The soil samples were placed in a biochemical incubator at constant temperature (20°C) for a certain time (1, 3, 5, 10 days, 15 days). The pH and conductivity of the solution were measured, and the direct shear test (fast shear) was immediately performed on the remoulded soil samples to measure the cohesion and internal friction angle.
3 Results
3.1 Variations in the solution parameters and mechanical parameters of montmorillonite-quartz sand remoulded soil
3.1.1 Variations in HNO3 solution parameters and cohesion of montmorillonite-quartz sand remoulded soil
Acid rain is a serious environmental problem caused by fossil fuel combustion and automobile exhaust emissions. In recent years, with the adjustment of energy resources and the development of transportation, the type of acid rain has gradually changed from the sulfuric acid type to the mixed type and nitric acid type, and the acidity has shown an increasing trend (Zhang et al., 2010; Qiao et al., 2017). The pH of acid rain in some areas is even less than 3, and the minimum is approximately 2.8 (Sun et al., 1994). To explore the influence of acid rain on the mechanical properties of clay exposed to environmental pollution, HNO3 solution with pH = 3 was selected for the immersion test in this study. The variations under acidic conditions are shown in Figure 1.
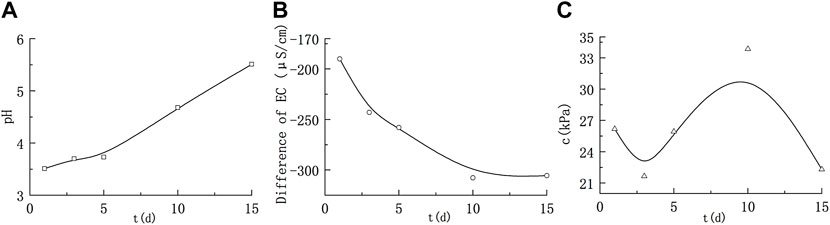
FIGURE 1. Variations in the solution parameters and cohesion of montmorillonite remoulded soil under acidic conditions. (A) Variation of pH; (B) Variation of electrical conductivity; (C) Variation of cohesion.
Under acidic conditions, the pH values were 3.51, 3.7, 3.73, 4.73 and 5.51 after soaking for 1, 3, 5, 10 and 15 days, respectively; the variation values of conductivity were -190, -242.9, -258, -307.7 and -305.5 μS/cm, respectively. With the extension of soaking time, the pH of the soaking solution showed an increasing trend and finally reached a nearly neutral value. The conductivity EC showed an overall decrease, and gradually slowed down in the later stage. Quartz sand was resistant to corrosion, and only montmorillonite could react with nitric acid, which consumed H+ in the solution and generated corresponding nitrates. At the same concentration, the conductivity of salt solution was usually weaker than the one of corresponding strong acid. So, with the reaction, the conductivity gradually decreased and finally stabilized, indicating that the reaction reached equilibrium.
It can be seen from Figure 1C the cohesion of montmorillonite remoulded soil under acid conditions shows a trend of first decreasing, then increasing and then decreasing. When soaked for 10ays–15 days, the cohesion decreased from 33.84 kPa to 22.31 kPa, which was lower than the cohesion value at the initial stage of soaking. Overall, except for the middle point (10 days), the cohesion decreased generally. However, after many repeated tests, the phenomenon of cohesion increase in the middle stage always exists and cannot be ignored.
3.1.2 Variations in NaOH solution parameters and cohesion of montmorillonite-quartz sand remoulded soil
The alkaline waste liquid produced in the process of industrial production in China is mostly discharged from paper mills, with pH as high as 12.94 (Gu, 1988) and strong alkalinity. In addition, the pH value of highly alkaline pore water formed during the operation of the nuclear waste repository is usually greater than 13, up to 13.5 (Ramirez, 2002), and the solutes are mainly NaOH and KOH (Berner, 1992). Direct contact between these alkaline waste liquids and soil will also cause changes in soil mechanical properties. In this study, NaOH solution with pH = 13.5 was used for the immersion test. The variations in the solution parameters and cohesion of montmorillonite-quartz sand remoulded soil are shown in Figure 2.
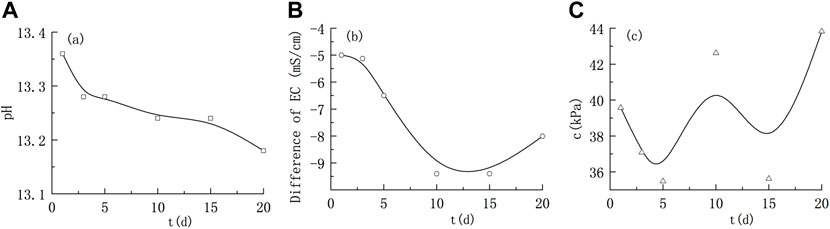
FIGURE 2. Variations in the solution parameters and cohesion of montmorillonite remoulded soil under alkaline conditions. (A) Variation of pH; (B) Characteristics of electrical conductivity; (C) Variation of cohesion.
Under alkaline conditions, the pH and conductivity of the solution showed an overall decreasing trend, indicating that montmorillonite reacted with a strong alkali and consumed OH− in the solution. At the initial stage of the reaction, the pH and electrical conductivity decreased significantly. From 1 d to 5 days, the pH and electrical conductivity changed from 13.36 and -5.0 mS/cm to 13.28 and -6.55 mS/cm, respectively. After 10 days, the pH decreased slowly, while the electrical conductivity rebounded. Finally, the pH value was 13.18, and the electrical conductivity changed to 8.01 mS/cm after 20 days.
Figure 2C shows that, under alkaline conditions, the cohesive force of montmorillonite-quartz sand remoulded soil showed an overall trend of first decreasing and then increasing. The cohesive force decreased briefly in the early stage of immersion (1 d–5 days), although the cohesive force fluctuated in the middle and late stages of immersion, it generally showed an increasing trend.
3.2 Variations in solution parameters and mechanical parameters of illite-quartz sand remoulded soil
3.2.1 Variations in hno3 solution parameters and cohesion of illite-quartz sand remoulded soil
The physical and chemical parameters and cohesion value under acidic conditions are shown in Figure 3. Under acidic conditions, the pH showed an increasing trend, while the conductivity EC decreased, indicating that the chemical reaction between illite and strong acid HNO3 consumed H+ in the solution and generated the corresponding nitrate. Under the same concentration conditions, the salt conductivity is usually weaker than the corresponding strong acid conductivity, so the overall conductivity decreased. At the same time, of the above changes in pH and conductivity, the cohesion value showed the characteristics of first decreasing, then increasing and then decreasing. Under acidic conditions, the cohesion of illite remoulded soil increased in the middle stage, which was similar to that of montmorillonite remoulded soil. In general, except for the middle stage of immersion, the cohesion showed a decline.
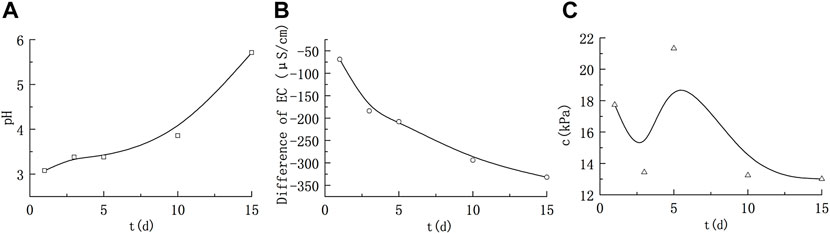
FIGURE 3. Variations in the solution parameters and cohesion of illite remoulded soil under acidic conditions. (A) Variation of pH; (B) Variation of electrical conductivity; (C) Variation of cohesion.
3.2.2 Variations in naoh solution parameters and cohesion of iillite-quartz sand remoulded soil
Under alkaline conditions (Figure 4), the pH was generally decreased, indicating that illite reacted with the strong alkali and consumed OH−. However, with the progress of the reaction, the pH rebounded slowly. After 10 days of reaction, the pH range was stable at 12.81–12.83, indicating that alkaline substances were generated in the reaction, and the pH gradually increased with the increase in alkaline substances. The overall electrical conductivity showed an increasing trend, indicating that the reaction generated soluble substances with strong conductivity, which increased the ion concentration of solution, such as K+ between illite layers.
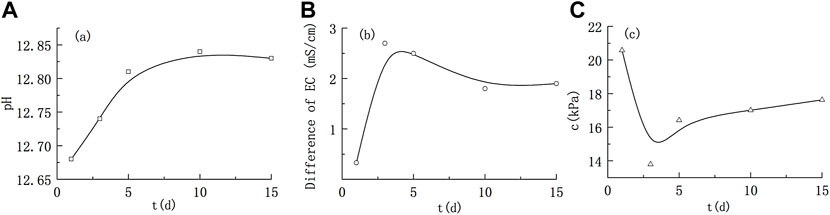
FIGURE 4. Variations in the solution parameters and cohesion of illite remoulded soil under alkaline conditions. (A) Variation of pH; (B) Variation of electrical conductivity; (C) Variation of cohesion.
The cohesion of illite-quartz sand remoulded soil showed a trend of decreasing first and then increasing. On the 5th day of reaction, the cohesion entered a stage of slow increase, and the later change gradually stabilized, with the range of cohesion values ranging from 16.42 to 17.63 kPa.
3.3 Variation characteristics of the internal friction angle under acid-base conditions
The change in the internal friction angle of montmorillonite-quartz sand remoulded soil and illite-quartz sand remoulded soil under acid and alkali conditions is shown in Table 2. Whether under acidic or alkaline conditions, the internal friction angle of illite-quartz sand remoulded soil showed little change. For montmorillonite-quartz sand remoulded soil, the internal friction angle remained basically unchanged under acidic conditions, while the internal friction angle increased steadily as the immersion time was prolonged under alkaline conditions.
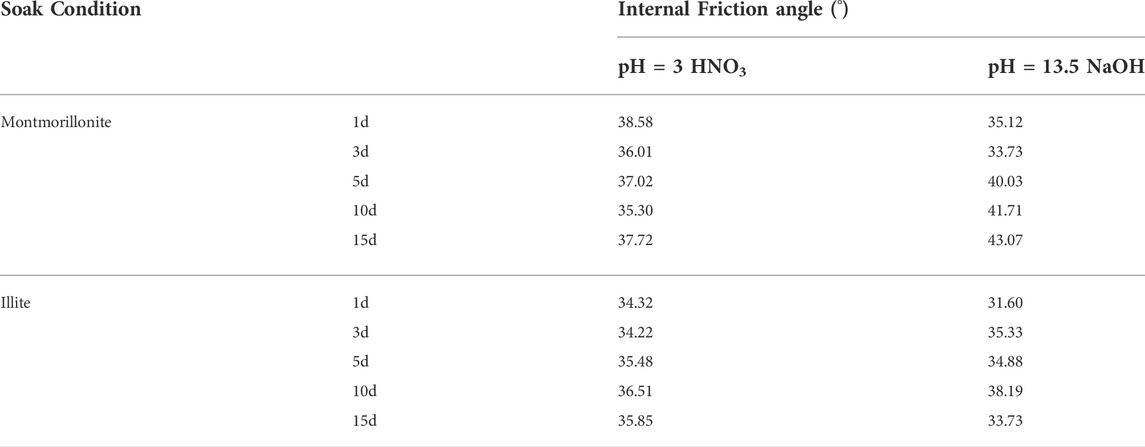
TABLE 2. Variations in the internal friction angle of clay mineral remoulded soil under acid-base conditions.
On the basis of the aforementioned changes in cohesion and internal friction angle, considering that the vertical pressure provided by the soil landslide is small (the thickness of the soil landslide is mainly concentrated in the two intervals of 0–5 m and 5–10 m (Qiu et al., 2012), and the density of the landslide soil is 2.0 g/cm3 (Gong, 1996), the vertical pressure values at thicknesses of 5 m and 10 m are only approximately 0.1 MPa and 0.2 MPa, respectively), the shear strength of illite-rich soil under acid and alkali action is consistent with the change in cohesion. For montmorillonite-rich soil, the change in shear strength is consistent with the change in cohesion under acidic conditions, and the shear strength increases overall under alkaline conditions.
4 Mechanism analysis of cohesion change
Cement is an important source of soil cohesion. In this study, the cement of the remoulded soil is mainly clay minerals. Under the action of acid and alkali, the chemical reaction between clay minerals and solution is an important reason for the change in cohesive force of remoulded soil. Therefore, based on the X-ray diffraction (XRD) analysis of the mineral composition changes of montmorillonite and illite samples before and after immersion, this study studied the change mechanism of remoulded soil cohesion under different water‒soil chemical interactions, combined with the changes of solution parameters.
4.1 Variation mechanism of cohesion of montmorillonite-quartz sand remoulded soil under acid-base action
4.1.1 Variation mechanism of cohesion of montmorillonite-quartz sand remoulded soil under acidic conditions
The XRD patterns of montmorillonite soaked in pH = 3 HNO3 and the original samples were superimposed and analysed. The results are shown in Figure 5. The intensity of the characteristic peaks of montmorillonite minerals changed. The intensity of the characteristic peaks with a diffraction angle of 5.7° decreased greatly from 1990 to 1,204, while the diffraction angles were consistent, which were 5.71° and 5.79° before and after soaking, respectively. The characteristic peak intensity of montmorillonite minerals at approximately 29° also decreased from 495 to 201, and the corresponding angles before and after soaking were 29.47° and 29.06°, respectively, which were consistent. The intensity of the characteristic peaks of other components in the sample did not change significantly, nor did the characteristic peaks of new substances appear. This shows that only montmorillonite minerals have a large degree of loss, and no new substances are generated after the montmorillonite samples reacted with HNO3. The chemical reactions in this process are shown in Eq. 4-1:
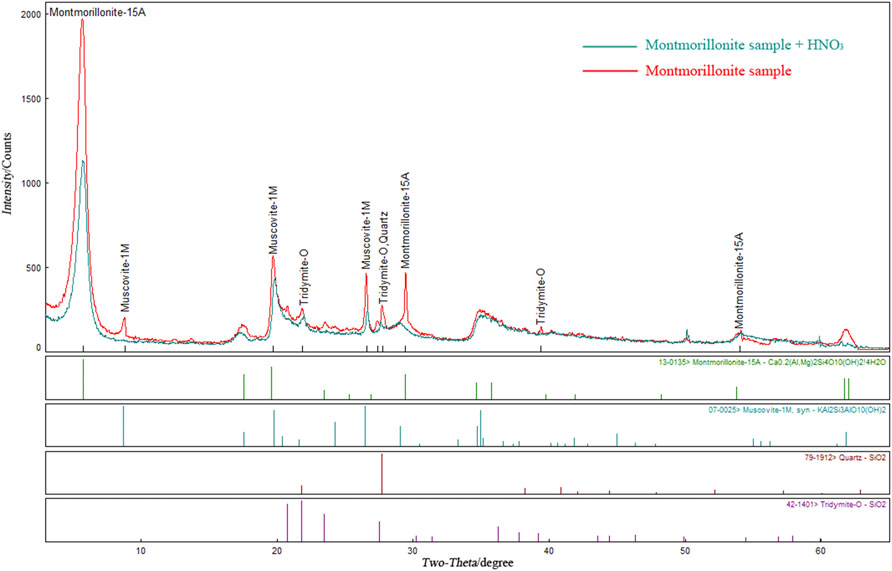
FIGURE 5. Comparison of XRD results of montmorillonite samples before and after immersion in HNO3 solution.
Based on XRD pattern analysis, combined with the variations of solution parameters and cohesion under acid conditions described in the previous section, it is considered that HNO3 will corrode and consume montmorillonite, resulting in the cementation being reduced in the sample, which was macroscopically manifested as a decrease in the cohesion of the remoulded soil. However, the increase in cohesion in the middle stage indicated that new cements were generated, which can provide greater cohesion for the soil, while no new material was found in the XRD pattern.
4.1.2 Variation mechanism of cohesion of montmorillonite-quartz sand remoulded soil under alkaline conditions
The XRD pattern of montmorillonite soaked in pH = 13.5 NaOH solution was superimposed with the original pattern of montmorillonite, and the results are shown in Figure 6. Compared with the XRD pattern of the original sample, the XRD pattern of the soaked samples changed significantly. The characteristic peak of montmorillonite minerals at approximately 5.7° shifted from 5.71° to 6.53°, and the intensity of the characteristic peak decreased sharply from 1990 to 519. The conversion from calcium montmorillonite (Ca0.2(Al,Mg)2SiO10(OH)2.4H2O) to sodium montmorillonite (Na0.3(Al,Mg)2Si4O10(OH)2·4H2O) indicates that Ca2+ in the montmorillonite structural layer was replaced by Na+ in the NaOH solution. The characteristic peak intensity of montmorillonite minerals at approximately 29° decreased from 495 to 204, and the corresponding angles before and after soaking were 29.47° and 29.42°, respectively, which were consistent. In addition, there are new characteristic peaks in the XRD patterns of the soaked montmorillonite samples. Comparative interpretation of the diffraction card database (PDF2) demonstrates that these new substances are mainly zeolite minerals (zeolite X, garronite) and calcium silicate hydrate CSH (xonotlite). Among these, calcium silicate hydrate CSH is a common cement in industrial cement, which includes ionic compounds connected with each other by chemical bonds; its bond strength is much larger than the van der Waals force between the montmorillonite layers.
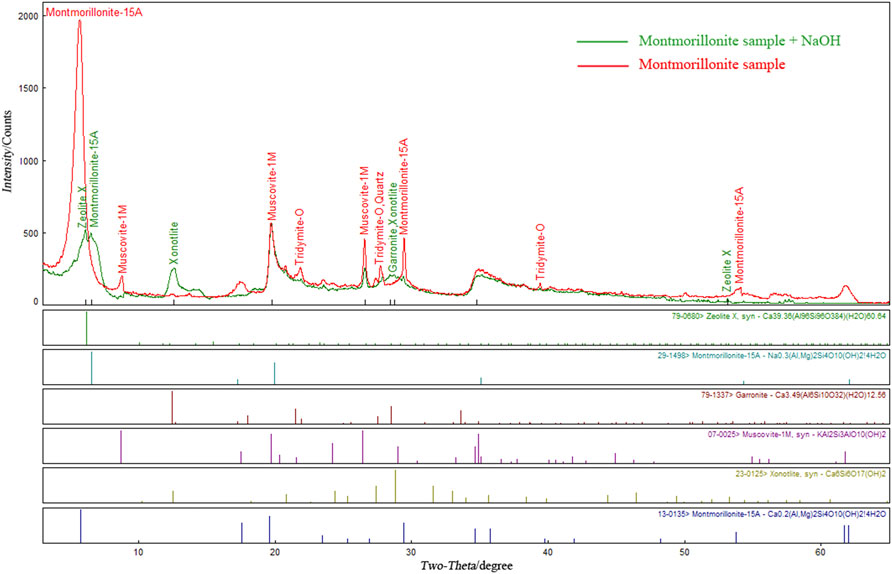
FIGURE 6. Comparison of XRD results of montmorillonite samples before and after immersion in NaOH solution.
Combined with the XRD analysis results, the reaction process mainly includes the following steps:
1) Ion exchange shown in eqs. 4–2 and 4–3:
2) The main components of montmorillonite minerals, silicon (SiO2) and aluminium (Al(OH)3), react with Ca(OH)2 by the chemical reactions shown in Eqs 4–4 and 4–5:
3) The formation of zeolite minerals and hydrated calcium silicate CSH
Calcium silicate CaSiO3 and calcium metaaluminate Ca(AlO2)2 further reacted to form aluminium-rich silicate mineral-zeolite minerals with higher Al/Si (atomic ratio of 0.5) than montmorillonite minerals (the Al/Si atomic ratios of zeolite X and garronite were 1.0 and 0.6, respectively). The residual Si atoms are retained in the form of cement hydrated calcium silicate CSH (xonotlite), which provides new and greater cohesion for the remoulded soil.
Combined with the variation in pH and EC, the change mechanism of the cohesive force of montmorillonite-quartz sand remoulded soil under alkaline conditions is analysed as follows:
1) At the beginning of the reaction, the strong alkali solution will corrode clay minerals so that the montmorillonite content in remoulded soil is greatly decreased, resulting in a decrease in cohesion. The montmorillonite denaturation (that is, from calcium montmorillonite to sodium montmorillonite) caused by ion exchange also plays a certain role in promoting the process.
2) The strong alkali reacts with montmorillonite to form a new cement hydrated calcium silicate CSH. Since CSH is connected by chemical bonds, with the increase in CSH production, the cohesion of remoulded soil stops decreasing and increases.
3) The pH value remained essentially unchanged in the middle and late stages, indicating that the reaction between the strong alkali solution and montmorillonite was largely complete. This may be due to the attachment of calcium silicate hydrate CSH and zeolite minerals to the surface of the montmorillonite, which blocked the contact between the strong alkali solution and montmorillonite. However, this does not affect the ion exchange between the high-valence exchangeable cation Ca2+ between the montmorillonite layers and the low-valence Na+ in the solution, resulting in an increase in the thickness of the dielectric layer of montmorillonite minerals, a decrease in the force between layers, and ultimately a decrease in cohesion.
4) The solubility of Ca(OH)2 is low. With the continuous exchange action, the concentration of Ca(OH)2 in the soil solution reaches saturation and precipitates. Since the precipitation of Ca(OH)2 involves ionic compounds, the cohesion rebounds in the later stage of immersion.
4.2 Variation mechanism of cohesion of lllite-quartz sand remoulded soil under acid-base action
4.2.1 Variation mechanism of cohesion of illite-quartz sand remoulded soil under acidic conditions
The illite samples soaked in pH = 3 HNO3 for some period of time were tested by XRD, and the patterns were superimposed and analysed with the original illite. The results are shown in Figure 7.
Figure 7 shows that, after the sample was soaked in pH = 3 HNO3, the intensity of the characteristic peaks of illite minerals at a diffraction angle of approximately 9° decreased significantly from 155.60×103 to 132.97×103, and the corresponding angles of the characteristic peaks before and after soaking were 8.9° and 8.98°, respectively, which were consistent. The characteristic peak intensity of illite minerals at approximately 17.8° also decreased from 61.56×103 to 50.63×103, and the corresponding angles before and after soaking were 17.78° and 17.86°, respectively, which were also consistent. There is no obvious change in the intensity of characteristic peaks of other components in the sample, and there is no formation of characteristic peaks of new substances. This indicates that only illite has a large degree of loss after interacting with HNO3, and no new substance is generated. Combined with the chemical formula of illite, the chemical reaction involved in this process is as follows (4–6):
Considering the changes in pH and conductivity EC of the acid solution, it can be considered that HNO3 corroded illite, which reduced the content of illite in the sample and led to a decrease in cohesion of the remoulded soil. The cohesion increased firstly and then decreased after the first decline. This growth process suggests the presence of substances that provide greater cohesion, but the XRD pattern shows that no new substances were generated. The phenomenon of increased cohesion in the middle stage has appeared more than once in this study, and its mechanism is still unclear.
4.2.2 Variation mechanism of cohesion of illite-quartz sand remoulded soil under alkaline conditions
The XRD pattern of the illite sample soaked in pH = 13.5 NaOH solution was superimposed with that of the original illite sample, and the results are shown in Figure 8.
Compared with the original illite sample, the map of the illite sample soaked in strong alkali changed to a certain extent, mainly showing a decrease in the intensity of various mineral characteristic peaks, but no new material characteristic peaks were generated. Figure 8 shows that, after soaking in pH = 13.5 NaOH solution, the intensity of the characteristic peaks of illite minerals at diffraction angles of approximately 9° and 17.8° decreased to 151.77×103 and 58.26×103, and the corresponding angles of the characteristic peaks after soaking were 9.0° and 17.9°, respectively, which were consistent. In addition, the intensity of the characteristic peaks corresponding to quartz in the sample also changed significantly, the intensity of the characteristic peaks at a diffraction angle of approximately 27° decreased significantly from 731.41×103 to 659.10×103, and the corresponding angles of the characteristic peaks before and after soaking were 26.68° and 26.8°, respectively, which were consistent. The characteristic peak intensity of quartz at approximately 21° also decreased from 159.86×103 to 150.92×103, and the corresponding angles before and after soaking were 20.9° and 21.02°, respectively, which were also consistent.
This indicates that illite and quartz in the sample were both depleted after reaction with strong alkali, especially quartz, but no new solid material was generated. The chemical reaction of quartz and the main components of illite minerals (silica (SiO2) and aluminium (Al(OH)3)) with NaOH are shown in Eqs. 4–7 and 4–8:
Sodium silicate (Na2SiO3) and sodium metaaluminate (NaAlO2) are strong alkaline and weak acid salts, respectively. During the reaction, the amount of production increases gradually, resulting in a slow recovery of pH. At the same time, since the above two products are soluble in water, they were not found in the XRD pattern of the samples after washing, filtering and drying. Combined with the above variations in pH and conductivity EC, it can be considered that NaOH corroded illite at the initial stage, which reduced the content of illite in the remoulded soil, so the cohesion fell. In addition, Na2SiO3 generated by the reaction of strong alkali with illite, also known as sodium silicate, has a certain degree of adhesion. As the amount of Na2SiO3 increased, the cohesion of the remodeled soil stopped decreasing and increased instead.
5 Conclusion and discussion
5.1 Conclusion
1) Under acidic conditions, for both montmorillonite-quartz sand remoulded soil and illite-quartz sand remoulded soil, as the reaction proceeded, the pH showed an increasing trend, the conductivity EC decreased overall, and cohesion showed the law of first decreasing, then increasing and then decreasing. On the whole, except for the medium immersion period, the cohesion decreased overall.
2) Under alkaline conditions, for montmorillonite-quartz sand remoulded soil, the cohesion decreased briefly in the early immersion stage and increased in the middle and late stages. The pH and EC showed a decreasing trend and gradually slowed down in the late stage. The cohesive force of illite-quartz sand remoulded soil first decreased and then increased. As the reaction proceeded, the pH value showed a slow upwards trend in the decreasing environment, and the conductivity EC increased overall.
3) Under the action of acid and alkali, the internal friction angle of illite-quartz sand remoulded soil has little overall change, and the change in soil shear strength is consistent with that of cohesion. For montmorillonite-quartz sand remoulded soil, the internal friction angle remains essentially unchanged under acidic conditions, and the change in shear strength is consistent with the change in cohesion. Under alkaline conditions, the internal friction angle increases steadily with the extension of soaking time, and the shear strength increases overall.
4) Under acidic conditions, montmorillonite and illite are corroded, and no new material is generated, resulting in a decrease in soil cohesion. The reason for the increase in cohesion in the middle stage is not clear. Under alkaline conditions, montmorillonite was corroded seriously, resulting in the formation of zeolite minerals (zeolite X, garronite) and new cement hydrated calcium silicate CSH (xonotlite). The strong alkali reacts with illite to form sodium metaaluminate (NaAlO2) and liquid cement Na2SiO3 (sodium silicate). The formation of new cements is the main reason for the increase in cohesion under alkaline conditions, and chemical corrosion and ion exchange cause a decrease in cohesion.
Discussion
1) The different effects and mechanisms of acid-base effects on soil cohesion provide a new prevention and control idea for soil landslides. For example, a certain amount of alkali can be applied to greatly improve the cohesion of soil and promote the formation of the internal cementation of the soil slope. Chemical measures can also be combined with structural engineering measures, internal bonding and external anti-sliding, which not only maximize the effect of prevention and control but also avoid disasters caused by engineering failure when the engineering life is exhausted. On the other hand, in areas with severe acid rain, considering the long-term cumulative effects of acid rain on the attenuation of soil cohesion, it is necessary to improve the design parameters of soil slope protection engineering and use an acid rain drainage groove (avoiding contact between acid rain and soil) to reduce the negative impact of acid rain on the stability of the soil slope to realize the full use of protective engineering.
2) The cohesive force of soil is controlled by the amount of cement, and the reduction and generation of cement are restricted by soil‒water chemical interactions, which, as a chemical reaction, must follow the chemical reaction kinetics and be controlled by the rate equation of the chemical reaction. Therefore, it is very necessary to carry out the quantitative model research of soil cohesion based on cement and the kinetics of water and soil chemical reaction, so as to realize the quantitative calculation of soil cohesion. This has important practical significance for the stability analysis of soil slopes and the prediction and early warning of soil landslides under the water‒soil chemical interaction.
Data availability statement
The original contributions presented in the study are included in the article/Supplementary Material, further inquiries can be directed to the corresponding author.
Author contributions
JL is the first author and corresponding author, is responsible for directing the experiments and paper writing. YG is the second author and is responsible for the processing of experimental data. ZS is the third author and is responsible for the production of figures and tables. HW is the fourth author and is responsible for checking the structure of this paper.
Funding
This work was supported by the Natural Science Foundation of Hebei Province, China (Grant No. E2019402361, E2020402075), Natural Science Foundation of Shanxi Province, China (Grant No. 201901D211549), Hebei Provincial Key Research Projects, China (Grant No. 21374104D), Science and Technology Research Projects of Higher Education Institutions in Hebei Province, China (Grant No. ZD2021309), Postdoctoral Research Projects of Hebei Province, China (Grant No. B2020003010), Innovation Fund Project of Hebei University of Engineering (Grant No. BSJJ 1930, BSHJJ 1904).
Conflict of interest
The author declares that the research was conducted in the absence of any commercial or financial relationships that could be construed as a potential conflict of interest.
Publisher’s note
All claims expressed in this article are solely those of the authors and do not necessarily represent those of their affiliated organizations, or those of the publisher, the editors and the reviewers. Any product that may be evaluated in this article, or claim that may be made by its manufacturer, is not guaranteed or endorsed by the publisher.
References
Berner, U. (1992). Evolution of pore water chemistry during degradation of cement in a radioactive waste repository environment. Waste Manag. 12 (2), 201–219. doi:10.1016/0956-053X(92)90049-O
Gratchev, I., and Towhata, I. (2011). Compressibility of natural soils subjected to long-term acidic contamination. Environ. Earth Sci. 64 (1), 193–200. doi:10.1007/s12665-010.0838-2
Gratchev, I., and Towhata, I. (2013). Stress-strain characteristics of two natural soils subjected to long-term acidic contamination. Soils Found. 53 (3), 469–476. doi:10.1016/j.sandf.2013.04.008
Gu, J. W. (1984). Influence of acid-base waste water pollution on engineering properties of clay. Shanghai Geol. 02, 42.
Gu, J. W. (1988). The influence of acid-base waste liquid erosion on foundation soil on engineering quality. J. Geotechnical Eng. 04, 72–78.
He, C., Shi, T. T., Zeng, W., Lin, G. H., and Lian, Z. P. (2020). Study on the leaching test of the sliding zone soil of huangtupo riverside 1# landslide. Saf. Environ. Eng. 04, 53–60. doi:10.13578/j.cnki.issn.1671-1556.2020.04.008
Liu, Y., Qiu, H. J., Yang, D. D., Liu, Z. J., Ma, S. Y., Pei, Y. Q., et al. (2022). Deformation responses of landslides to seasonal rainfall based on InSAR and wavelet analysis. Landslides 19 (1), 199–210. doi:10.1007/s10346-021-01785-4
Ma, S., Qiu, H., Hu, S., Dongdong, I., and Liu, Z. J. (2021). Characteristics and geomorphology change detection analysis of the Jiangdingya landslide on July 12, 2018, China. Landslides 18 (1), 383–396. doi:10.1007/s10346-020-01530-3
Qiao, X., Du, J., Kota, S. H., Ying, Q., Xiao, W. Y., and Tang, Y. (2017). Wet deposition of sulfur and nitrogen in Jiuzhaigou National Nature Reserve, Sichuan, China during 2015-2016: Possible effects from regional emission reduction and local tourist activities. Environ. Pollut. 233, 267–277. doi:10.1016/j.envpol.2017.08.041
Qiu, H. J. (2012). Study on the regional landslide characteristic analysis and hazard assessment: A case study of ningqiang countyXi’an. United States: Northwest University.
Ramirez, S., Cuevas, J., Vigil, R., and Leguey, S. (2002). Hydrothermal alteration of “La Serrata” bentonite (Almeria, Spain) by alkaline solutions. Appl. Clay Sci. 21 (5), 257–269. doi:10.1016/S0169-1317(02)00087-X
Sun, C. X., Zhang, J. H., Shu, L. C., and Chen, Y. M. (1994). The influence of acid rain and acid mist on clean insulators’ AC flashover performance. High. Volt. Appar. 26 (2), 14–18.
Tiwari, B., Tuladhar, G. R., and Marui, H. (2005). Variation in residual shear strength of the soil with the salinity of pore fluid. J. Geotech. Geoenviron. Eng. 131, 1456. doi:10.1061/(asce)1090-0241(2005)131:12(1445)
Wang, J., Cao, P., Zhao, Y. L., and Chai, H. B. (2010). Influence of chemical action of water-soil on soil shear strength. J. Central South Univ. Sci. Technol. 41 (1), 245–250.
Xu, H., and Liu, H. Z. (2019). Multi-scale rainfall characteristics of rainfall-induced landslides. Mt. Res. 037 (6), 858–867. doi:10.16089/j.cnki.1008-2786.000476
Yan, R. M., Teng, W. F., and Yan, R. X. (2013). Research on shear strength properties and microstructure of sliding soil in landslides under water-soil interaction. Yangtze River 44 (22), 82–55. doi:10.16232/j.cnki.1001-4179.2013.22.002
Yang, D. D., Qiu, H. J., Ma, S. Y., Liu, Z. J., Du, C., Zhu, Y. R., et al. (2022). Slow surface subsidence and its impact on shallow loess landslides in a coal mining area. Catena 209, 105830. doi:10.1016/j.catena.2021.105830
Yong, R. N., Sadana, M. L., and Gohl, W. B. (1984). “Compositional control on swelling behaviour of an expansive soil,” in Proceedings of the fifth international conference on expansive soils, Australia, 21-23 May 1984. (Vermont: ARRB Group Limited).
Yu, H. H., and Sun, D. A. (2019). Shear strength of weakly expansive soils in differernt solution. Chin. J. Undergr. Space Eng. 15 (2), 423–427.
Zhang, X. M., Chai, F. H., Wang, S. L., Sun, X. Z., and Han, M. (2010). Research progress of acid precipitation in China. Res. Environ. Sci. 3 (5), 527–532. doi:10.13198/j.res.2010.05.3.zhangxm.005
Zhao, Y., Cui, P., Hu, L. B., and Hueckel, T. (2007). “First north American landslide conference, landslides and society: Integrated science,” in Evolution of shear strength of clayey soils in a landslide due to acid rain: a case study in the area of Three Gorges, China. Landslide Conference, June 3-8, 200 7 (Colorado USA: Vail), 1656–1666.
Zhao, Y., Cui, P., and Hu, L. B. (2009). Relation between evolution of clay shear strength and landslide induced by acid rain: Taking landslides in Three Gorges reservoir area for example. Chin. J. Rock Mech. Eng. 28 (3), 576–582. doi:10.3321/j.issn:1000-6915.2009.03.017
Zhao, Y., Cui, P., Hu, L. B., and Tomasz, H. (2011). Multi-scale chemo-mechanical analysis of the slip surface of landslides in the Three Gorges, China. Sci. China Technol. Sci. 54 (7), 1757–1765. doi:10.1007/s11431-011-4370-8
Zhou, W. Q., Qiu, H. J., Wang, L. Y., Pei, Y. Q., Tang, B. Z., Ma, S. Y., et al. (2022). Combining rainfall-induced shallow landslides and subsequent debris flows for hazard chain prediction. Catena 213, 106199. doi:10.1016/j.catena.2022.106199
Keywords: water-soil chemical interaction, acid-base action, montmorillonite, illite, mechanical effect
Citation: Liu J, Guan Y, Shao Z and Wang H (2022) Mechanical effect of clay under the acid-base action: A case study on montmorillonite and illite. Front. Earth Sci. 10:991776. doi: 10.3389/feart.2022.991776
Received: 12 July 2022; Accepted: 19 July 2022;
Published: 16 August 2022.
Edited by:
Haijun Qiu, Northwest University, ChinaReviewed by:
Wu Pengfei, Taiyuan University of Technology, ChinaXingqian Xu, Yunnan agriculture university, China
Copyright © 2022 Liu, Guan, Shao and Wang. This is an open-access article distributed under the terms of the Creative Commons Attribution License (CC BY). The use, distribution or reproduction in other forums is permitted, provided the original author(s) and the copyright owner(s) are credited and that the original publication in this journal is cited, in accordance with accepted academic practice. No use, distribution or reproduction is permitted which does not comply with these terms.
*Correspondence: Jian Liu, NTEwMjEzNUAxNjMuY29t