- 1Guangdong Research Institute of Water Resources and Hydropower, Guangdong Engineering Laboratory of Estuary Hydropower, Guangzhou, China
- 2State Key Laboratory of Estuarine and Coastal Research, East China Normal University, Shanghai, China
- 3Section of Environmental Fluid Mechanics, Faculty of Civil Engineering and Geosciences, Delft University of Technology, Delft, Netherlands
Sediment is found throughout the world’s alluvial plain rivers, estuarine coasts and adjacent seas and is thereby a key factor in major ecosystems. Suspended mineral sediment can affect the biological activity of microorganisms and plants, by reducing light penetration in the water column or by binding to organic matter. Biological processes can, in turn, affect the physical and chemical properties of the sediment particles and influence the adhesion between particles. They can facilitate the sediment aggregation (flocculation) through bridging, patching and sweep, while biological decay will mainly help to disintegrate organic matter rich flocs. Biological activity also affects the properties of flocs (structure, density, sedimentation rate and composition). This activity is itself influenced by environmental conditions (like temperature, light and nutrient fluxes). Sediment flocculation thus involves complex relationships between several physical, chemical and biological factors. The role of biology in particular needs to be better integrated in sediment transport models, through the interaction between mineral clay particles, microorganisms and their excreted polymers (Extra Polymeric Substances, i.e., EPS). In this article, a summary of the state-of-the-art research regarding sediment flocculation is given. In particular, the action of organic matter on fine-grained sediment flocculation is discussed. The aim of the article is to provide a more comprehensive understanding of bio-sediment dynamics and give an outlook on remaining research questions.
1 Introduction
Sediment is fundamental to the evolution of natural aquatic environments such as rivers, estuaries and lakes (van Leussen, 1988; Eisma and Irion, 1993; van Leussen, 1994; Manning et al., 2010b). Suspended sediment dynamics play an important role in geomorphological evolution, biogeochemical cycling, transport of pollutants, and aquatic biological activity (McAnally and Mehta, 2001). Cohesive sediment refers to a mixture of clay- and silt-size particles with a small amount of fine sand-size particles. This cohesive sediment is composed of mineral clay, organic matter and water in various amounts. Even though the proportion of clay-size particles and organic matter is only 5%–10% in most cohesive sediment, their presence results in remarkable rheological properties (yield stress, viscosity) of the overall sediment and can lead, in the water column, to a process called flocculation (the aggregation of mineral clay particles with themselves and/or with organic matter) through electrochemical and biochemical interactions (Dyer, 1989; Winterwerp and Kesteren, 2004; Chassagne, 2019; Chassagne et al., 2021).
Over 90% of the total volume of fine suspended sediment in an aquatic environment exists in the form of aggregates, i.e., flocs (Droppo and Ongley, 1994). Figure 1 is adapted from a classical picture illustrating the behavior of cohesive sediment in aquatic systems. It was adapted so as to show the effects of some organic parameters (EPS, short for Extra Polymeric Substances, and living microorganisms such as microalgae) on suspension, flocculation/break-up, settling and deposition. Mineral clay particles come in the water column either by hydrodynamic transport or resuspension by erosion from the bed (labels 1 and 2 in Figure 1). Under the action of microscopic forces which are linked to the particles’ surface charge (van Leussen, 1988; Gregory, 2005), particles will flocculate, and form flocs, which can be large in volume and size. Flocs are usually large when they are made of a high content of organic matter, as organic matter has a low density and therefore relatively large organic matter particles can be found in the whole water column and thus aggregate with mineral sediment. To give an order of magnitude, flocs are usually found in the size range 20–200 μm, and flocs of highest size in this range (including flocs of even higher sizes) are organic-matter rich (Safar, 2022). This organic matter comes either from the water column (microalgae and their EPS) or from the bed, where biofilms can be created (Droppo, 2009; Lai et al., 2018; Ho et al., 2022). Pure mineral clay flocs (flocs composed of mineral clay aggregated through electrostatic interactions) remain limited in size by the Kolmogorov microscale (Mietta, 2010). Flocs can be divided into smaller or primary flocs under the action of shear stress or grow larger by collision with other particles (labels 3–5 in Figure 1). As the environmental factors are changing, it is argued that flocs stay in a dynamic process of aggregation and break-up (Burban et al., 1989; Eisma, 1991; Lick et al., 1993). This classical picture should however be reconsidered in the light of organic matter properties: when organic matter consists of polymeric substances (such as proteins, sugars or DNA), flocs display elastic properties and show a high resistance to shear (Shakeel et al., 2020). Increasing shear rate then does not lead to break-up of flocs but rather to a decrease in volume, for a constant mass (the flocs become denser).
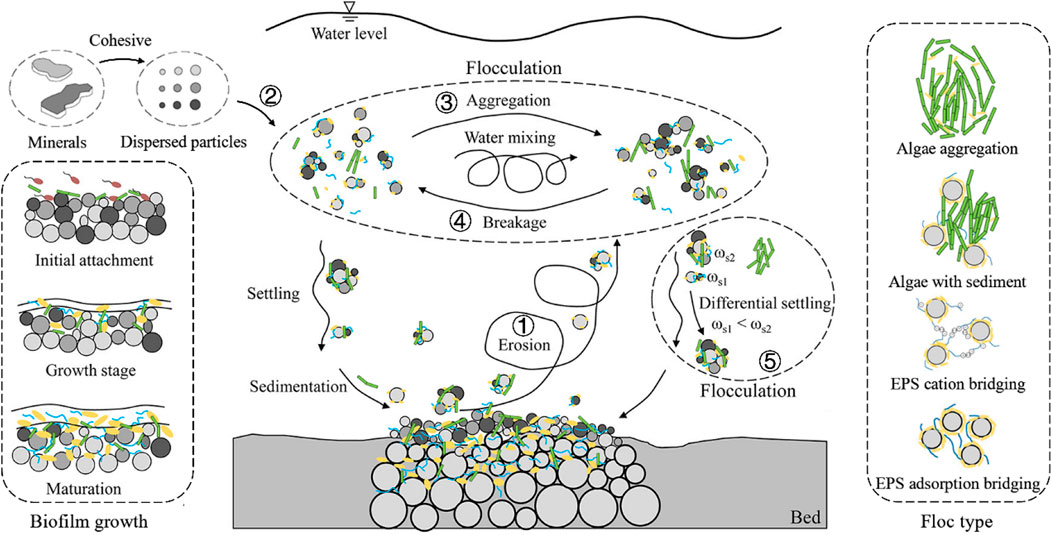
FIGURE 1. Flocculation processes including biological effects (the figure is modified after Maggi, 2005; Guo, 2018; Lai et al., 2018; Deng, 2022). The processes are: 1—Erosion: In the original figure, these particles were represented as mineral clay particles. It is now established that organic matter plays a crucial role in erosion, due to the presence of biofilms (Droppo et al., 2015; Horemans et al., 2021). The growth of biofilms is illustrated on the left. 2—Mineral clay particles enter the system by advection; the particles of clay and silt size can be composed of layers of aluminosilicates sheets that are bound together more of less strongly, depending on the clay mineralogy; the surface charge of these particles will determine their ability to flocculate.3—Aggregation: Mineral clay particles can bind together by salt-induced aggregation, but most often, in coastal environments, mineral clay particles will bind to organic matter (Deng et al., 2019; Deng et al., 2021). The different types of flocs are illustrated on the right. 4—Break-up: Under the action of shear, flocs might break. It was recently established that in the presence of organic matter flocs predominantly become smaller by reconformation (Chassagne and Safar, 2020; Shakeel et al., 2020). 5—differential settling. Due to the density difference between flocs (based primarily on their organic matter content), flocculation can occur by differential settling (Deng et al., 2019; Deng et al., 2021).
Flocculation is affected by three main types of factors: physical factors, chemical factors, and biological factors. There is quite some overlap between these different types, and one could well speak of physico-chemical factors or bio-chemical factors. Physical factors include hydrodynamic conditions (such as shear stress and vertical mixing) and sediment particle characteristics (such as particle size distribution and sediment concentration); chemical factors consist of environmental conditions (such as salinity, temperature, pH), mineral composition and surface charge of sediment particles; biological factors mainly refer to the action of microorganisms and their excreted biopolymers (Gibbs, 1985; Dyer and Manning, 1999; Winterwerp and Kesteren, 2004; Mietta et al., 2009a; Droppo et al., 2015; Fettweis and Lee, 2017). Many factors depend on each other (the role of biopolymers in flocculation is for instance related to salinity and temperature) which makes parametrizing of flocculation models extremely complex.
In the past 30 years, a lot of research has been performed on flocculation but the effect of biological processes was seldom taken into account (Thomas et al., 1999; Winterwerp, 1999; Maggi, 2005; Mietta, 2010). As there is a significant biological activity in aquatic environments, it has long been recognized that organic matter has a great impact on flocculation (Avnimelech et al., 1982). It is therefore not surprising that research on the effect of organic matter on sediment flocculation is gradually becoming more extensive. Most of the studies, however, are focused on the qualitative description of such flocculation. The distinction between different bio-flocculation mechanisms, the different floc types and their link to the factors cited above are still lacking. In this review, those correlations are discussed. The purpose of the review article is to summarize the state-of-the-art regarding sediment flocculation and presenting some of the open questions to be answered in the future.
2 The population balance model
In the standard model used to describe flocculation (the Population Balance Equation, PBE in short), a size class i is defined as a collection of particles with concentration ni, all particles in a same class having the same size (diameter)
Aggregation and break-up processes of particles are expected to occur simultaneously and dynamically in the PBE model (Winterwerp and Van Kesteren, 2004; Chassagne, 2019; Chassagne et al., 2021).
Two parameters are related to the particles’ aggregation ability: the collision frequency and the collision efficiency. The aggregation rate of particles depends on both these parameters.
2.1 Collision frequency
There are three main expressions for the collision frequency, based on the different approach mechanisms between particles. Particles can approach one another by Brownian motion, mixing by shear or differential settling (McCave, 1984; van Leussen, 1994; Tsai and Hwang, 1995). So, if a collision occurs between a particle of diameter
Brownian motion (BM):
Mixing by shear (SH):
Differential settling (DS):
The parameter
Brownian motion is caused by the random thermal motion of liquid molecules, so in general it is only effective for sediment particles under the colloidal size of 1–2 μm (Dronkers et al., 1988; Lick et al., 1993; Partheniades, 1993). Brownian-induced flocculation is a slow process and in estuarine systems, where the water column experiences hydrodynamic mixing, it is not expected to be the dominant cause for flocculation.
The effect of shear rate is considered to be important for flocculation in estuarine systems. Many studies (Mehta and Partheniades, 1975; Eisma, 1991; Partheniades, 1991, 1993; Manning and Dyer, 1999; Winterwerp et al., 2006; Mietta et al., 2009a) have provided a better understanding about the relations between shear rate and flocculation. As is shown in Eq. 2, the collision frequency increases with increasing shear rate, thus promoting flocculation and the growth in particle size. However, when the particle size of flocs reaches the Kolmogorov microscale η, the increase upon shear rate will be limited. Eisma (1986) and van Leussen (1997) in particular argued that the maximum diameter of flocs should be equivalent to the Kolmogorov microscale (minimum turbulent vortex scale) and this was also experimentally verified for salt-induced flocculation of mineral clay particles (Mietta et al., 2009a; 2009b). The Kolmogorov microscale is defined in Eq. 6.
2.2 Collision efficiency
The collision efficiency is mainly driven by the interaction forces between particles upon approach. These forces are linked to physical, chemical and biological processes, and include electrostatic forces, Van der Waals force (VDW), hydrophobic interactions and entropic forces. In the past decades, researchers have put forward a number of theories and models for sediment flocculation, among which there is the standard electrokinetic theory (named DLVO, for Derjaguin, Landau, Verwey and Overbeek), polymer bridging, cation bridging, sweep flocculation and others (Kruyt, 1949; van Leussen, 1988, 1994; Higgins and Novak, 1997; Bolto and Gregory, 2007; Lee et al., 2011). When studying the aggregation of similar primary particles (the primary particles being similar is one of the underlying hypothesis of the PBE model), the DLVO theory can provide a useful parameter (the ζ-potential) which helps to predict whether aggregation will or will not occur.
2.2.1 DLVO theory
The DLVO theory models the interaction potential between two approaching colloidal particles in a solvent (usually water). The interaction is made of two terms: a Coulombic repulsion and a van der Waals’ attraction (Kruyt et al., 1952; Chassagne, 2019). The two particles have a surface charge of same sign and are surrounded by a so-called double layer which is composed, in majority, of counterions. The double layer is the result of the attractive interaction between the surface charge of sediment particles (usually negatively charged for mineral sediment) and the positively charged ions (cations) found in water. The ζ-potential is used to quantify the surface charge of a particle. It is defined as the electric potential at the surface of shear of the particle (the potential of reference is taken at infinity, where it is defined as zero). When this surface of shear is located at the surface of the particle, the ζ-potential is identical to the electric surface potential
As is seen in Figure 2A, the electric potential drops from
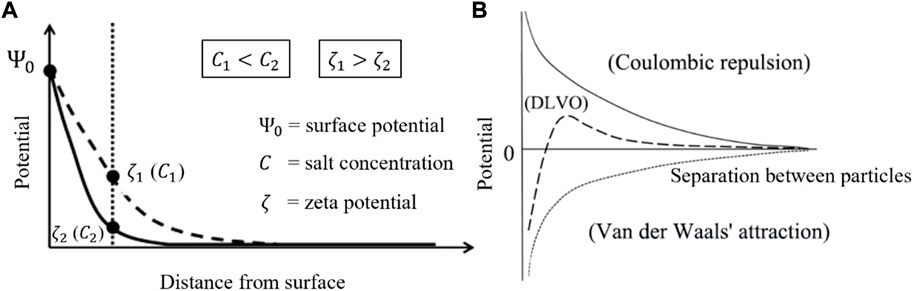
FIGURE 2. (A) Electric potential as function of the distance from the surface of a colloidal particle. The ζ–potential of particles is a function of salinity: the higher the salinity, the lower the ζ–potential. (B) The DLVO interaction energy between two particles as the sum of an attraction (van der Waals) and a repulsion (Coulomb). (figures modified after Chassagne et al., 2009).
For more details about DLVO theory, the reader is referred to Chassagne (2019). There exists a lot of studies about the ζ-potential and the surface charge of clays (Rand and Melton, 1977; Sondi et al., 1996; Kosmulski and Dahlsten, 2006; Mietta et al., 2009a; Chassagne et al., 2009; Mietta, 2010; Tsujimoto et al., 2013), and the results show that either a decrease in pH or an increase in ionic strength usually decreases the absolute value of the ζ-potential. A low ζ-potential is synonymous for aggregation ability: particles with a low ζ-potential have a weak Coulombic repulsion. For these particles, van der Waals attraction will dominate and lead to flocculation Figure 2B. It was shown in (Mietta et al., 2009a; 2009b) that the ζ-potential could be related to the collision efficiency.
2.3 Break-up mechanisms
When shear forces are stronger than the bonding force between particles, it is expected that flocs will break-up.
The break-up rate
where
where
In general, the floc break-up process is poorly understood (Thill et al., 2001). The empirical parameters should account for the bonding forces between particles as the stronger the bonds, the more resistant the floc is to any exterior force (Jarvis et al., 2005) or inner tensile stress (Yeung and Pelton, 1996), see Figure 5. The ability of flocs to resist damage (floc strength) is closely related to flocculation and the size and structure of flocs (Son and Hsu, 2009). Floc strength is a function of the electrochemical attraction between clay minerals and the agglutination of organisms and organic matter on particle surface (Passow, 2002; Bainbridge et al., 2012).
2.3.1 The Kolmogorov microscale
Shear stress is one of the most important external factors affecting the flocculation process and has been widely studied (e.g., Dyer and Manning, 1999; Mietta et al., 2009b; Partheniades, 1993; Winterwerp, 1998).
The turbulent shear rate G is often used to quantitatively describe the turbulent strength:
where
3 Flocculation by organic matter
Organic matter, and more specifically the polyelectrolytes produced by microorganisms bind to sediment particles in different ways, depending on their polymeric chain length (defined by the polymeric molecular weight), and charged groups. Their way to bind to sediment particles is also depending on salinity, pH and shear stresses. Polyelectrolyte-induced flocs can achieve sizes much larger than the Kolmogorov microscale, owing to the polyelectrolyte shape (chain-like) and elastic nature (Ibanez Sanz, 2018; Shakeel et al., 2020).
Depending on the polyelectrolyte, flocculation between mineral sediment and polyelectrolyte can occur through bridging (Riley, 1963; Nabzar et al., 1988; Hicks, 1988; Winterwerp and Kesteren, 2004), patching mechanism (Bergaya and Lagaly, 2013) or sweep, as shown in Figure 3.
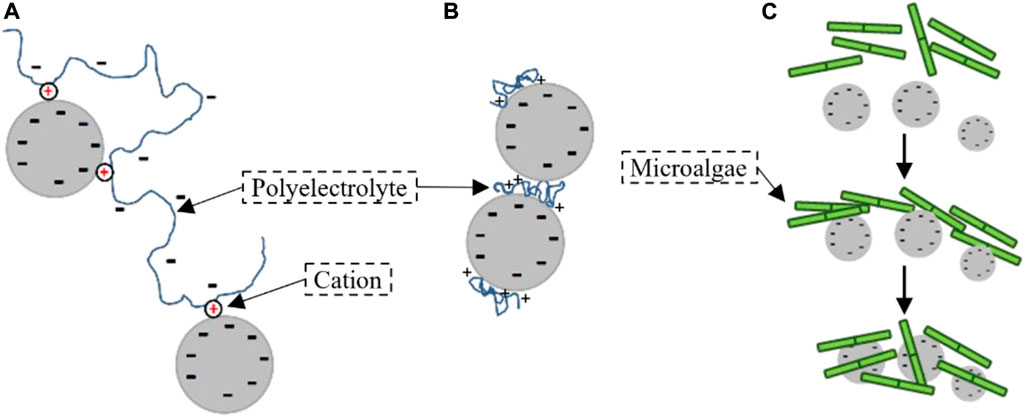
FIGURE 3. A schematic diagram of different types of flocculation. The grey disks symbolize negatively charged mineral clay particles (A) bridging flocculation. The anionic polyelectrolyte needs a cation (in red) to bridge to the clay; (B) patching flocculation. The cationic polyelectrolyte binds easily to the clay through attractive Coulombic forces; (C) sweep flocculation. (figures modified after Chassagne, 2019).
A polyelectrolyte can stick to certain points of the sediment’s surface as trains, separated from one another by loops and for much of its length it is able to extend into the solvent as tails (Figure 4). The attraction between the interacting molecular chains should be strong enough to overcome the entropy repulsive force caused by the decrease in freedom of the chains (Rosen, 2004).
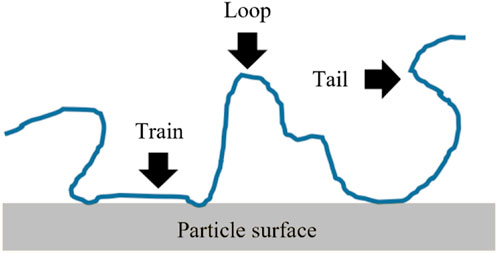
FIGURE 4. Attachment configurations of a polymer to a sediment particle surface: tail, train and loop. (figure modified after Chassagne, 2019).
Under suitable conditions, microbial organisms, such as diatoms, can produce polyelectrolytes (polysaccharides), which is a gelatinous organic matter (OM). These polyelectrolytes are called Transparent Exopolymer Particles (TEP) or Extracellular Polymeric Substances (EPS). They are polymeric chains which include a large amount of anionic polysaccharides like galacturonic acid that is the main component of pectin (Plude et al., 1991). The organic polymers can form biofilms on the surface of sediment particles and act as flocculating agent (Bar-Or and Shilo, 1988b; Bar-Or and Shilo, 1988a). Because of the ionization of their functional groups (such as carboxylic acid and phosphate), microbial cells and EPS have a high density of negative charges (Sheng et al., 2010), and anionic polysaccharides aggregate with negatively charged sediment particles through cationic bonding. These cations are salt ions found in the water, and their concentration is a function of salinity. The EPS secreted from microorganisms can also be a cationic polymer (Plude et al., 1991). Aly and Letey (1988) have found that flocculation by cationic polymers is different from flocculation by anionic polymers. Flocculation with cationic polymer is done by charge neutralization, while flocculation with anionic polymer is mainly done by bridging mechanism (Shakeel et al., 2020). Studies have shown that adding divalent cation can enhance flocculation (Yeh, 1988; Park et al., 2010).
3.1 Bridging aggregation
Bridging aggregation occurs when a free polyelectrolyte in solution captures suspended mineral particles (see Figure 3A). Usually, one finds that the optimum polymer concentration to achieve flocculation corresponds to half surface coverage for the polymer. Polymeric bridges are changing as function of shear. Bridging aggregation can even occur with polyelectrolytes having surface charges of same sign as the ones of the particles. In that case, aggregation is enabled by the presence of oppositely charged ions in the water. When polymer bridging flocculation happens, the bridging particles should have an available surface to connect polymer chain segments (Biggs et al., 2000). Flocs formed by bridging through polymer chains are flexible and stretchable (Otsubo, 1992; Gregory and Barany, 2011).
3.2 Patching aggregation
Patching aggregation occurs when polyelectrolytes have a charge that is opposite in sign to the one of the sediment particles (see Figure 3B). The polyelectrolyte then strongly binds to the sediment particle, and its tails do not extend much into the solvent. Aggregation is then made possible between one polymer patch of one particle and the bare surface area of another particle. Flocs formed through patching aggregation have generally a higher strength than those formed through other ways. The force is dependent on the number of bound segments (Swenson et al., 1998).
3.3 Sweep flocculation
As is visible under a microscope, microorganisms can form a polymeric network with holes and channels (Li and Ganczarczyk, 1990) through the bridging of their produced Extracellular Polymeric Substances (EPS) (Leppard, 1992; Jorand et al., 1995). This network has a large surface area and can absorb pollutants, nutrients and minerals (Costerton et al., 1987). At the same time, there are large and small interspaces inside the network. When sedimentation of this network occurs, some fine particles can be trapped by it and be embedded (see Figure 3C). This process is called sweep flocculation. Although sweep flocculation is usually studied in sewage treatment, a similar process has been observed in estuarine environments (Gregory, 2005; Lee et al., 2011). Sweep flocculation is a function of electrochemistry and other characteristics of the network. As a result, the network floc gets increasingly larger (Deng et al., 2019).
To summarize, the flocculation of fine sediment under the influence of organic matter is a complicated dynamic process involving chemistry, physics and biology. The time-dependence of flocs composed (in part) of organic matter is poorly understood, especially in relation with the living microorganisms that are known to bind to mineral sediment thanks to EPS (Deng et al., 2019; Deng et al., 2021; Safar et al., 2022).
Recent studies have found that organic matter-induced flocculation is also affected by biological decay and that the strength of bonds is time-dependent, see Figure 5. Flocs can therefore break without the influence of shear, simply by biodegradation (Mikutta et al., 2007; Jeldres et al., 2018).
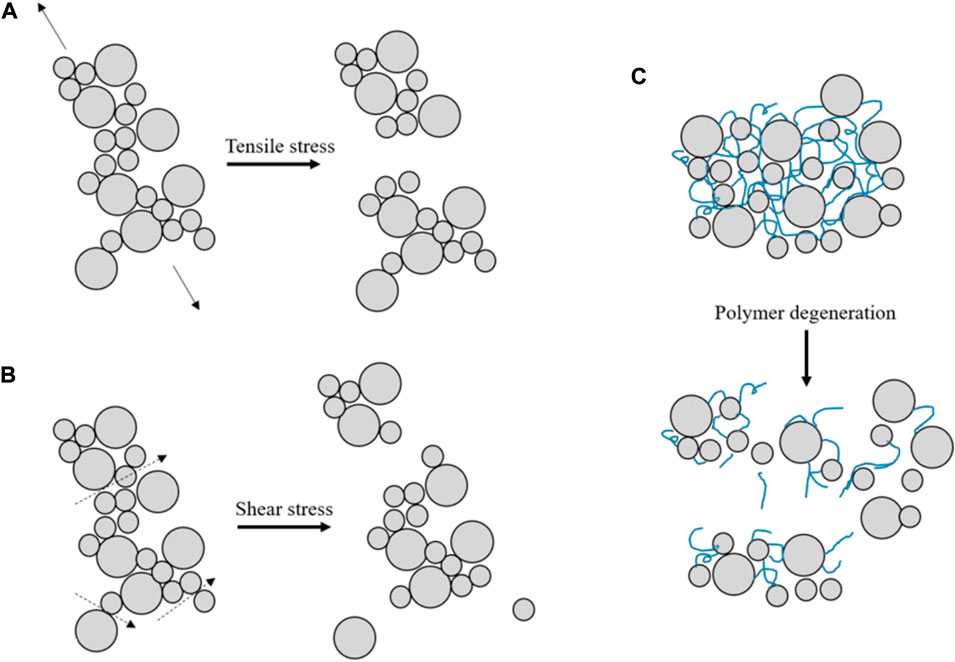
FIGURE 5. A schematic diagram of floc break-up processes, (A) Break up by tensile stress (B) Break up by shear stress (C) Break up by polymer degeneration (figure modified after Jarvis et al., 2005; Lai et al., 2018).
The rate of microbial degradation depends on the environment and leads to catabolism (Foree and McCarty, 1970). The degradation is different for different types of EPS (Zinkevich et al., 1996; Comte et al., 2006). Living microorganisms (that produce EPS and other types of polyelectrolytes) are function of grazing (Porter, 1973) and water nutrients (Chai et al., 2006; Li et al., 2014). Further study on the degradation of EPS in combination with its interaction with sediment is required.
4 Discussion on flocculation models
4.1 The PBE vs. the logistic growth model
Using the PBE model explained in Section 2, it is found that an increase in turbulent shear stress increases the collision frequency between sediment particles hereby promoting sediment particle aggregation. On the other hand, high shear stresses reduce the Kolmogorov microscale
Using the Population Balance Model, the steady-state average size of a floc will be the Kolmogorov microscale (Mietta, 2010). When a floc (in the model) has a size larger than the Kolmogorov microscale, it is expected to break-up into smaller flocs with a denser structure (Dyer, 1989; Winterwerp, 2002).
Previous studies suggest that the maximum size of flocs formed in turbulent flow is controlled by
Dyer (1989) first suggested that there exists a critical value of shear stress, below which the floc size increases and after which decreases and conceptualized this in a figure (Figure 6). The optimum in particle size as function of shear stress is only occurring if all experiments (for each shear stress) are done for a fixed flocculation time, and that the equilibrium floc size has not yet been reached. Indeed, if the equilibrium floc size would have been reached for all experiments, for fully suspended particles, flocs formed at the lowest shear would be the largest and the floc size as function of shear rate would be a monotonic decrease, as has been shown by Mietta et al. (2009b). For a fixed (short) flocculation time, it is expected that below a critical shear rate the flocculation rate is limited by the amount of collisions and above this critical shear by the Kolmogorov microscale. This is however not true for organic matter flocs—they can grow larger than the Kolmogorov microscale and their flocculation rate is extremely fast (Shakeel et al., 2020).
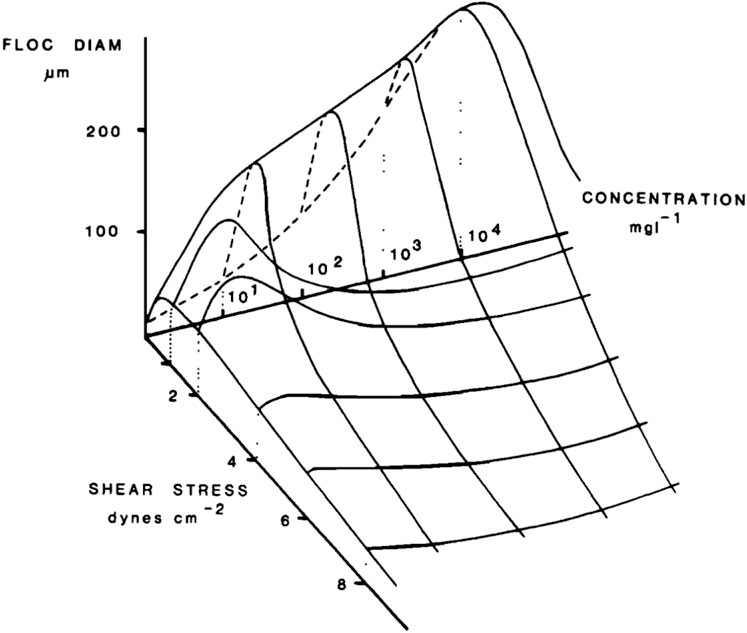
FIGURE 6. A conceptual diagram of the relationship between floc size, turbulent shear and suspended sediment concentration (figure following after Dyer, 1989).
Note that on Figure 6 there is also a maximum in floc size as function of concentration for a given shear rate. The reason for this optimum is unclear, as one would expect, for any flocculation time, either an increase in floc size as function of concentration (related to an increase in collision frequencies) or that the floc size remains constant (equal to the Kolmogorov microscale
The fact that flocs follow the Kolmogorov microscale is true for salt-induced flocs (Mietta, 2010), under the condition that the flocs have reached their equilibrium size and that they remain suspended. At low shear, it can be shown that for dense particles, not all particles remain in suspension and hence the average mean diameter decreases with the increasing number of particles that are not suspended (Mietta et al., 2009b). It should be noted that in the case of low turbulent shear stress, the flocculation process may change from being dominated by turbulent shear stress to being dominated by differential settling, thus affecting the flocculation process and the characteristics of the final flocculation (Lick et al., 1993; Chen et al., 1994; Fugate and Friedrichs, 2003).
The characteristic values of shear stress between these two regimes have been obtained in many studies, including laboratory experiments (e.g., Manning and Dyer, 1999; Mietta et al., 2009a; Kumar et al., 2010) and field observation studies (e.g., Markussen and Andersen, 2014; Sahin, 2014; Guo et al., 2017). Markussen and Andersen (2014) and Guo et al. (2017) showed that the characteristic turbulent shear rate in their in-situ studies was about 3–4 s−1, whereas the critical range obtained in other studies (Manning and Dyer, 1999; Serra et al., 2008; Kumar et al., 2010; Zhang et al., 2013; Sahin, 2014) was about 15–40 s−1, indicating that the flocs properties might be different in different estuaries.
In estuarine and coastal areas, the largest shear gradient is close to the channel bed (10%–20% of the water column above bed is affected), where about 80% of turbulence energy is present. This produces a powerful uplift force for particles and the local shear stress controls the maximum size of suspended flocs (Mehta and Partheniades, 1975). From a PBE modelling perspective, in an area with constant shear stress, the strength, size and density of flocs will be in a dynamic equilibrium. When the shear stress is varied from low to high, flocs will become smaller. When the shear rate is varied from high to low, flocs will grow larger. These processes are completely reversible when the PBE model is used, implying that the structure of a floc of a given size will always be the same, independently of its history.
One can question the validity of the PBE model in the case of in-situ flocculation, for several reasons. First of all, it is not proven, as the PBE assumes, that the size of a floc is governed by a dynamic balance between aggregation and break-up. As was shown in Chassagne and Safar (2020), it is well possible to model flocculation data by using a logistic growth model. This model, in contrast to the PBE model does not require break-up to ensure a realistic steady-state floc size. Indeed, without the break-up term, the PBE model would give an infinitively large equilibrium floc size. As discussed in Chassagne and Safar (2020), Chassagne et al., (2021), an equilibrium floc size can, for example, be reached because of a depletion of flocculating agent (like EPS). Over time, mineral sediment and EPS bind together until all available EPS is covered with mineral sediment and the floc size reaches a constant value. The amount of EPS is then the limiting factor for the equilibrium floc size (Shakeel et al., 2020). Using the PBE model, a steady-state floc size (not an equilibrium size) can be reached only when there is a balance between the aggregation and break-up terms, which then keeps the floc size constant over time. This type of balance is for instance found in thermodynamics, when one studies the adsorption/desorption of molecules from a surface (Langmuir, 1918), but is not representative for EPS-induced flocculation.
Secondly, it has been observed that flocs do not necessarily break under shear, but rather change shape as the organic matter they contain is rather elastic. This implies that, even at constant shear, sediment and organic matter concentration, floc sizes can decrease over time due to the fact that flocs become denser (less volume, same mass) (Shakeel et al., 2020). Using the PBE model, it is only possible to decrease a particle size by break-up, therefore this process cannot be modelled. Using a logistic growth model, on the other hand, it is possible to model this decrease in floc size and parameterized it (Safar et al., 2022).
The logistic growth model is therefore a promising new tool for flocculation studies, but it requires further investigation. In particular some work should be devoted to the parametrization of the model and its calibration using in-situ data.
4.2 The DLVO model and prediction of flocculation
In estuarine areas, the suspended sediment particle will, according to DLVO theory, be destabilized and flocculate because of the increase in salinity between the river fresh water and the sea. In the presence of organic matter however, DLVO theory cannot always be applied, as the flocculation mechanisms will be driven by the presence of polyelectrolytes and microorganisms which are not accounted for in the DLVO theory.
Hunter and Liss (1979) already demonstrated in 1979, by analyzing the surface properties of suspended particles in four rivers in the United Kingdom by electrophoresis (a technique to assess the ζ-potential) that because of the organic coatings of the particles no major difference could be found in the particles’ surface characteristics.
Different clay minerals with different particle sizes and surface charges are found in flocs. Goldberg (1991) found that the optimum flocculation salinity for different clay minerals is different. The optimum flocculating salinity of illite and kaolinite is 9–13 PSU, while that of montmorillonite is 20–24 PSU. This result is in line with DLVO theory, as montmorillonite clay, being delaminated, has a larger surface area than kaolinite or illite particles and hence the particles experience a larger Coulombic repulsion.
The general trend that was found is that the electrophoretic mobility (and hence the ζ-potential) is decreasing (in absolute values) with increasing salinity. This behavior is in line with the description given in Figure 2A and reflects the screening of the surface charge of the coated particles.
On the other hand, the DLVO approach, which predicts that the increase of ion concentration is beneficial for flocculation, cannot explain why an increase in sodium concentration will slow-down flocculation in some situations (Sobeck and Higgins, 2002). Physico-chemical models also do not take into consideration the uneven charge distribution on sediment surfaces and the charge distribution of EPS, which most probably have a great influence on bio-sediment flocculation. Moreover, the colony formation and growth of microorganisms is a dynamic process that will influence bio-sediment flocculation. Microbial activity is a complex process that is hard to quantitatively study, especially in field observations. So far, the biological activity is studied by quantifying the number of microorganisms present in the water, understanding their growth cycle, analyzing their EPS, etc. The link between biological activity and sediment flocculation remains a key open question for the study of sediment transport dynamics.
5 Characterization of floc properties
Properties of flocs include size, shape, effective density and composition. These parameters are important to understand the cohesion of flocs and their settling velocity. The settling velocity of flocs is an important parameter for sediment transport modeling (Winterwerp, 1998; Manning et al., 2011; Verney et al., 2011; Chassagne et al., 2021).
5.1 Floc sizes and shapes
Generally, floc size is assessed by laser diffraction techniques (with equipment like Malvern Mastersizer and LISST) (Dyer and Manning, 1999; Agrawal and Pottsmith, 2000; Mikkelsen and Pejrup, 2001; Mietta, 2010; Filippa et al., 2011; Guo et al., 2017). These techniques convert the raw data (diffracted light) into particle size by making use of the assumption that particles are spherical and represented by their equivalent mean diameter. The particle sizes are given in logarithmically-spaced size bins, and the particle size distribution (PSD) is given as a volume-% (volume occupied by particles of a sizes within a given bin compared to the volume occupied by all particles). It is usually assumed that the D50 of the distribution is representative for the mean particle size of the distribution (Maggi, 2005). Nonetheless, it often occurs that a PSD displays multiple size peaks (van Leussen, 1994), which reflects the fact that different types of particles are present in the sample, such as mineral sediment, flocs and microorganisms like algae (Simon et al., 2002; Grossart et al., 2003; Tang, 2007; Deng et al., 2019; Safar, 2022). Microorganisms generally develop extensive web-like networks on flocs, with a larger size and lower fractal dimension than those without microbial colonization (Kiørboe et al., 1990; Azetsu-Scott and Passow, 2004).
Multiple peaks in PSD also occur because of the shape of particles (Agrawal and Pottsmith, 2000; Safar, 2022). For example, Liu et al. (2007) found by scanning electron microscope technique that the sediment flocs of the Changjiang estuary at different salinities and organic matter have various shapes. Safar (2022) found, from underwater camera investigations, that flocs composed of a large amount or exclusively of organic matter are highly anisotropic, when this organic matter is composed of chain-like microalgae. Mhashhash et al. (2018) showed that at different suspended sediment concentrations (SSC) flocs of different shape are found. In high shear stress areas such as estuaries and coasts, flocs are usually small and spherical or ellipsoidal (van Leussen, 1994; Craig et al., 2020). Some typical flocs are given in Figure 7. In low shear stress environment, the flocs are usually elongated in a chain-like fashion due to their algal composition (Manning et al., 2007; Safar, 2022). The drag force on these flocs will be different and this will thus affect the aggregation between particles leading to larger flocs of various shapes (Maggi, 2005; Adachi et al., 2012). Anisotropic flocs containing a substantial amount of organic matter can withstand a certain extent of deformation, leading over to the reconformation of these flocs into fairly spherical flocs (Jeldres et al., 2018; Safar, 2022). Degradation of polymers can also lead to this phenomenon (Heath et al., 2006).
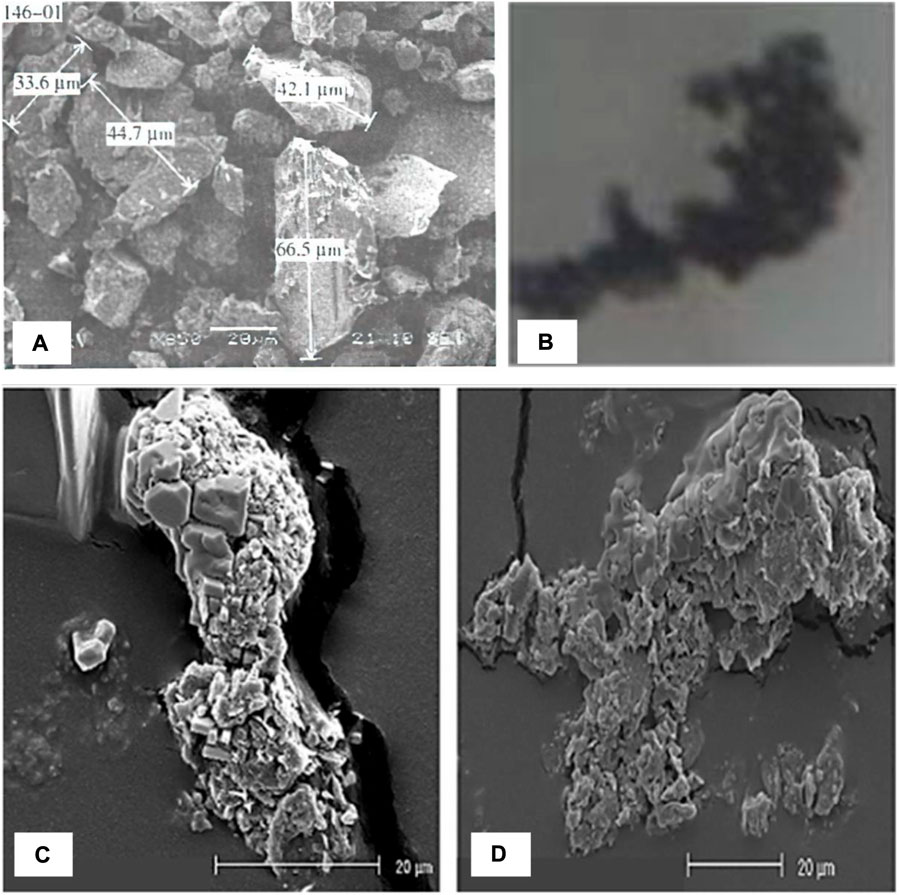
FIGURE 7. Photomicrography of flocs, (A) electron microscope photographs of flocs in the freshwater with humus, figure from Liu et al. (2007) (B) Camera photographs of flocs in the Rhine Region Of Freshwater Influence (Rhine ROFI), figure from (Safar, 2022) (C,D) SEM photographs of flocs at salinity 20, different SSC, c = 100 and d = 200 mg L−1, figures from: Mhashhash et al. (2018).
5.2 Floc density and settling velocity
Figure 8 shows the relationship between effective density (floc particle density minus water density) and floc size obtained from several published studies. The results are for many different rivers and estuaries, such as the upper Tamar River in the United Kingdom (Fennessy et al., 1994), the Po River Estuary in Italy (Fox et al., 2004) and the Changjiang River Estuary (e.g., Wang et al., 2013; Guo et al., 2017) and laboratory experiments with natural sediment (e.g., Gibbs, 1985; Manning and Dyer, 1999). The effective density of flocs displays a significant decrease as function of particle size which can be of several orders of magnitude. However (not shown) in all these experiments there were also quite some large density differences for a given particle size. These differences arise from the fact that flocs of a given size can have very heterogeneous composition (Chassagne and Safar, 2020).
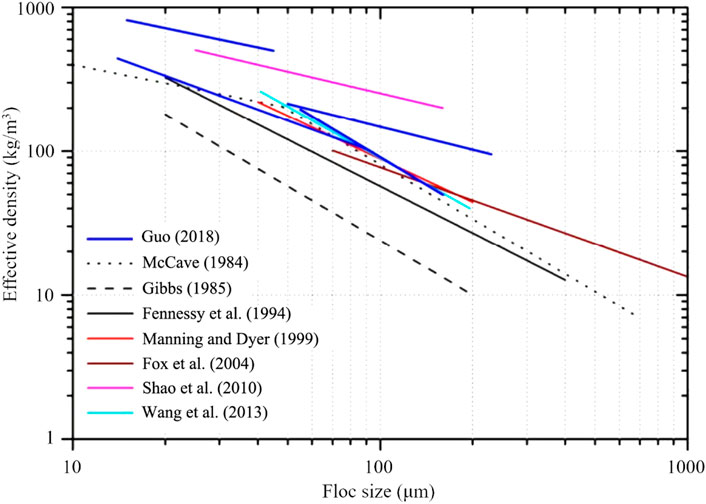
FIGURE 8. The change of effective density of flocs with particle size (McCave, 1984; Fennessy et al., 1994; Manning and Dyer, 1999; Fox et al., 2004; Shao et al., 2011; Wang et al., 2013; Guo, 2018).
To describe quantitatively the relation between floc size and floc effective density, Kranenburg (1994) proposed that the flocs should be treated as fractals. A fractal is defined as a self-similar object (Mandelbrot, 1982). In existing studies, there are two main descriptions of fractals: one is the two-dimensional fractal dimension
where
Usually the density of flocs is obtained from settling velocities experiments from which, using Stokes’ settling velocity, the density can be deduced. In general the settling velocity of a sinking object is expressed as:
in which
The particle Reynolds number is given by Re = ωs
For spherical particles,
In fine sediment transport models, the settling velocity is usually taken to be constant, and its value is about 0.05–0.1 mm s−1 (e.g., Geyer et al., 2004; Hu et al., 2009). This assumption of a constant settling velocity is an approximation, as it was discussed above that flocs can change shape and density as function of shear (and time).
Experiments in controlled conditions and field observations have given evidence that the organic matter (especially the EPS) has substantial effects on floc density, porosity and settling velocity that leads to a larger size but lower density and settling velocity as compared to the pure (without EPS) sediment particles (Droppo, 2001; Guenther and Bozelli, 2004; Passow and De La Rocha, 2006; Bowers et al., 2007; Droppo et al., 2015). On the other hand, some studies found that organic matter can increase floc density under certain conditions (Fall et al., 2021). Low density and large size flocs are predominantly formed in the water column where large particles of organic matter (like microalgae) are advected. Dense and small flocs with comparable settling velocity can be formed in regions where organic matter is less abundant, or where the shear is high, as by shearing flocs become denser and get a more spherical shape (by coiling) (Deng et al., 2019; Deng et al., 2021; Chassagne et al., 2021).
5.3 Floc composition and relation to flocculation and settling
It is usually assumed that flocs are composed of fine mineral sediment (clay and silt fraction) with some amount of organic matter (van Leussen, 1988, 1994). However, sand can also sometimes be entrapped in flocs (Deng et al., 2021).
5.3.1 Mineral sediment
The content of clay minerals in the suspended sediment of the Changjiang estuary is about 26%, of which 65%–70% are illite, and the rest are chlorite, montmorillonite and kaolinite. The settling velocity of illite is 9 times higher than that of montmorillonite when the salinity is 10 PSU. The main types of clay minerals vary as function of the tidal periods because the sediment transport and deposition mechanisms are different (Zhang, 1996). The laboratory experiments and field observations of the flocculation characteristics of fine sediment in the Changjiang Estuary show that the optimum flocculation salinity ranges from 4 to 16 PSU (Guan et al., 1996; Jiang et al., 2002; Wan et al., 2015). Manning et al. (2010a) studied the flocculation process with different mud/sand mixtures and demonstrated that different ratios of mud and sand can influence the flocs settling velocity.
5.3.2 Extracellular polymeric substances and microbial communities
Flocs can be regarded as individual microecosystems with autonomous and interactive chemical, physical, and biological reactions and processes within the floc matrices (Droppo et al., 1997; Evans et al., 1997; Brinkmann et al., 2019). EPS and other polymeric substances are produced by microorganisms. These microorganisms can be microscopic algae (such as diatoms), which can form large flocs by themselves, and afterwards capture sediment particles (Deng et al., 2019).
The presence of organic matter like EPS promotes the flocculation of fine sediment (e.g., Droppo and Ongley, 1992; Gratiot and Manning, 2004; Mietta et al., 2009b; Fettweis and Baeye, 2015). It was found that organic matter play a major role in the floc collision rate and floc strength in the observation of seasonal variation of flocs. Large flocs usually appeared at algae bloom seasons due to biologically induced aggregation (Mikkelsen, 2002b; Uncles et al., 2010; Lee et al., 2014; Fettweis and Baeye, 2015). Besides, the biological effects on sediment flocculation also are found in fresh water environments (Guo and He, 2011).
Therefore, the organic matter composition of flocs is not only sensitive to environmental conditions but also varies with spatiotemporal changes. An overview of different studies is given in Table 1.
Organic matter composition can only be assessed in the laboratory. In-situ observations do however nowadays include Chlorophyll a measurements, which gives some indication of the microalgae content of flocs (Fettweis and Lee, 2017; Deng et al., 2019, 2021; Ho et al., 2022).
5.3.3 Type of microalgae studied
Diatoms are the largest component of microalgae which goes by the name phytoplankton. Therefore, most of the research on the effects of microorganisms on sediment flocculation in estuaries are done with diatoms. Diatoms in healthy state will remain suspended due to turbulence and water current.
Phytoplankton is a large community with strong adaptability. These microorganisms can live under very low nutrient concentration, very weak light intensity and quite low temperature. They can not only grow in rivers, streams, lakes and oceans, but also in short-term ponding or humid places (Blum, 1956; Round, 1981).
In 1968, Walsby (1968) described the aggregation of N. nutans, by the EPS this microorganism is secreting. Avnimelech et al. (1982) studied the aggregation process of Chlamydomonas and collar algae with clay by electron microscope in 1982 (Figure 9). This is the earliest image of algae and sediment flocculation. Besides, Avnimelech et al. (1982) compared the effect of four different forms of algae on clay flocculation and showed that algae can significantly promote the flocculation of clay in the presence of electrolytes. Therefore, the reason why the growth of algae is restricted in high turbidity areas may not only be due to the limited light supply but also because of the fact that algae have flocculated with sediment particles. In addition, some algae species are prevented to flocculate due to steric effects linked to the spatial conformation of their filamentous strains (de Lucas Pardo, 2014).
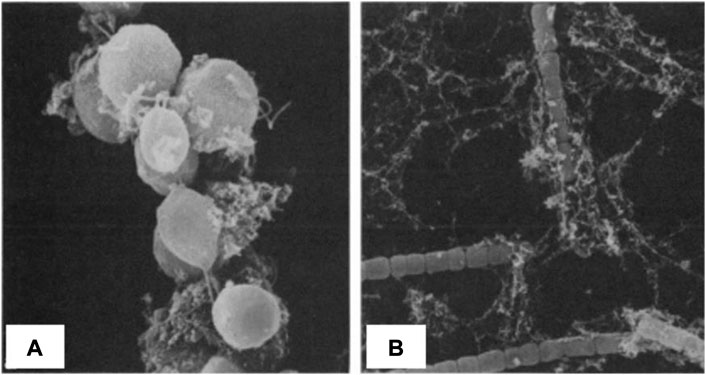
FIGURE 9. Flocs under scanning electron microscope (A) Chlamydomonas clay cluster, (B) Anabaena clay cluster, 1,200 times, 1,470 times; figures following after Avnimelech et al. (1982).
5.3.4 Effect of temperature and light
Temperature is the main factor affecting the geographical distribution of algae. Therefore, in the sea area where the water temperature changes greatly, the species changes greatly through the year. In winter, there are cold water algae (the optimum temperature for growth and reproduction is less than 4°C). When the temperature drops to 4°C, both the number of algae and the EPS will however usually decrease (Domozych, 2007; Kiemle et al., 2007), and the production of EPS will also decrease, thus reducing the effect on flocculation (Wilén et al., 2000).
There are warm water algae (the optimum temperature for growth and reproduction is about 20°C) in summer, which can complete their life cycle in a short time. The highest value of photosynthesis is between 20 and 25°C, when the algae growth reaches the maximum value (Colijn and van Buurt, 1975; Blanchard et al., 1996), and algae then produce EPS with a high viscosity (Lupi et al., 1991).
Most of freshwater microalgae appear in spring and autumn. Some cyanobacteria only appear when the water temperature is high in summer. Light is the decisive factor to determine the vertical distribution of algae. The light absorption capacity of a water body is very strong, so the light intensity at 10 m depth is only 10% of that of water surface, and the light intensity at 100 m deep is only 1% that of water surface. Moreover, because seawater can easily absorb long-wave light, it also causes a spectral difference in light at various water depths. Different algae have different requirements for light intensity and spectrum. Green algae generally live at the surface of water, while red algae and brown algae can use short wave light such as green, yellow and orange to live in deep water (Reynolds, 2006; Wood, 2014; Castro and Huber, 2016).
5.3.5 Effect of water chemistry
The chemical properties of the water body are also important factors for the appearance and species composition of algae (Pearson et al., 1987; Zou et al., 2011). For example, Cyanobacteria and Gymnophyta can live in eutrophic water and often form blooms; Bacillariophyta and Chrysophyta often exist in lakes with poor nutrition in mountainous areas; Chlorophyta and Cryptophyta often occur in small ponds. In addition, the interaction between algae living in the same water area also plays an important role in their emergence and growth, as some algae can inhibit the growth of other algae by secretions.
The pH value of water has a direct impact on the physiological activities of bacteria and microorganisms on the surface charge of mineral sediment. pH can therefore significantly affect (bio)flocculation by changing the EPS activity (Yokoi et al., 1995; Yokoi, 2002). With the increase of pH in a water body, the functional groups on the mineral sediment (and EPS) such as carboxyl and amino groups will dissociate or not leading to an increase or decrease in surface charge. Different bacterial species have different adaptability to pH and therefore, the effect of pH on sediment flocculation needs further study.
5.3.6 Other compositions
Other components of flocs are heavy metals, Humic substances (HS), pore water, and xenobiotic particulate matter (e.g., Microplastics (MPs), Engineered nanoparticles (ENPs), oil droplets). These elements have a unique function in floc formation (Droppo, 2001; Maggi, 2013; Ho et al., 2022). For instance, metal-rich precipitates are often found in flocs with a heterogeneous composition (Jonas and Millward, 2010). HS have strong negative charges, and, when adsorbed on suspended particles, will increase their negative charge. This leads to an enhanced stability of sediment particles (Bob and Walker, 2001; Fettweis and Lee, 2017). Pore water is present in the pore space in the floc matrices when particles aggregate to form large flocs (Droppo, 2001) which affects floc structure and density (Sherman, 1953; Logan and Hunt, 1987). MPs can become associated with SPM and become incorporated into floc matrices (Michels et al., 2018) and provide habitats and substrates for microorganisms, which can create biofilms and scavenge various dissolved and particulate matter (Petrova and Sauer, 2012; Taylor et al., 2016). Oil droplets can easily attach to sediment particles, becoming a part of flocs and affect transport behavior (Droppo et al., 2016). With the intensification of human activities, more and more heterogeneous substances can be absorbed to part of flocs, which makes the study of floc composition and its degradation over time a worthwhile study.
6 Concluding remarks
In conclusion, flocculation of cohesive sediment mainly includes two processes: aggregation and decay [break-up by biodegradation or shear—whereby shear can also lead to a densification of flocs (without breakage)]. In these two processes, physical, chemical and biological factors will have a role and also interact with each other. These factors can either promote aggregation or decay. For example, turbulent shear stress and suspended sediment concentration can promote particle collision frequency and facilitate flocculation, but a very high shear will limit flocs growth. The effect of salinity (cation concentration) is important as well, as it enables in most cases to bind (negatively charged) sediment to (negatively charged) organic matter. Organic matter (such as EPS) will usually enhance the stability of sediment particles, but can also degrade over time and lead to decay (break-up) of flocs.
Although many studies have been performed in the field of flocculation, floc characteristics and factors affecting sediment flocculation, there are relatively few studies on biological effects on sediment flocculation. Many authors have begun to pay attention to the study of biological factors in recent years, however, in most studies, the effects of organic matter and microbial communities are not differentiated, whereas the effects of these two factors should be distinguished in the context of sediment flocculation. Even though it is the microbial community that produces polymeric organic matter (such as EPS), two types of flocculation can be found: EPS acts on the surface properties of sediment while living microalgae aggregate themselves to form large flocs first, and then catch sediment particles within their network. A summary of the different processes involved in flocculation, and their link to the parameters required for sediment transport models are given in Figure 10.
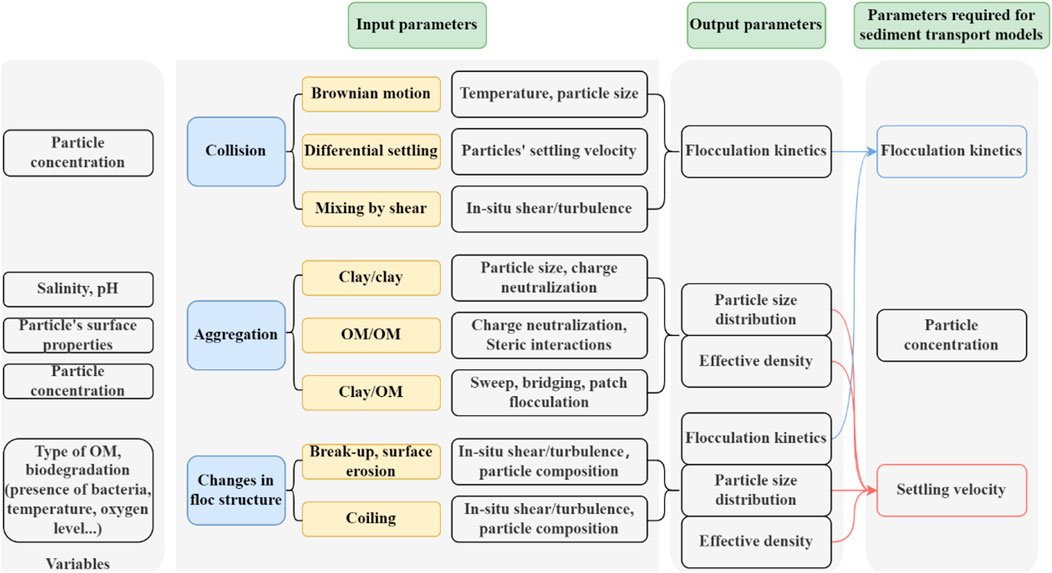
FIGURE 10. Parameters of influence and their interactions during the flocculation process; In order to model sediment transport, the (numerical) models have as input a few classes of particles defined by concentration and settling velocity. The changes in number of particles in a class and their settling velocity are related to flocculation kinetics.
Based on the discussions in the present article, the following points need to be studied in the future regarding the influence of organic matter on flocculation processes:
In terms of flocculation mechanisms, research has demonstrated that organic matter influences mineral sediment flocculation and flocs. Organic matter can promote flocculation but also inhibit the flocculation process. These different effects are often related to different species of algae or different nature of microbial secretions, but a clear overview is still lacking. Most studies have focused on the exopolymers produced by microbial secretions, but overlooked the heterogeneous composition of flocs. It has been shown that algae and microorganisms themselves can form flocs and participate in the sediment flocculation process, and hence more research is needed to understand the dynamic link between microorganisms, exopolymers and mineral sediment. Finally, most of the research on the mechanisms of flocculation is still qualitative, and although some studies have been able to quantify the effects of organic matter, a fully parameterized and calibrated model is still lacking. A promising tool might be the model based on logistic growth that has recently been developed and shown to be working for laboratory studies on algae and EPS induced aggregation of mineral sediment.
In terms of floc properties, most of the current research has focused on floc size variation and less on properties such as floc composition, effective density and structural property variation. The study of these properties is necessary to understand the role of organic matter on flocculation and the time behavior of flocs (change in structure, degradation, etc.).
In terms of flocculation influencing factors, there is a large amount of research on the physical factors influencing sediment flocculation, such as the grain size distribution of sediment particles, hydrodynamics, etc., but there is still relatively little research on the combined physical and biochemical factors. Unlike parameters such as hydrodynamics, sand content and salinity, biochemical parameters, such as microalgae type and concentration are more difficult to control and quantify. Therefore their study, especially in laboratory experiments, require a multidisciplinary approach and the development of new equipment and techniques.
Finally, the large-scale transport of flocs in the overall water ecosystem are still poorly studied. Most quantitative studies are done in the laboratory but there is a need to connect these studies to numerical large-scale models. The flocculation model parametrization can be done in the lab and its implementation in large-scale transport model will help to better study the effect of microscopic, small-scale processes on large-scale sediment transport. To this end, it is also important to further develop in-situ monitoring tools, so as to integrate the monitoring of organic matter in the water column.
Ecological issues are becoming increasingly important in present days societies. Sediment transport cannot anymore be seen as a purely physical process and the role of biology should be accounted for, as organic matter changes the properties of mineral sediment through flocculation. Importantly, through the interactions between mineral sediment, organic and xenobiotic particulate matter, the transport of microorganisms, nutrients and pollutants is a dynamic process in the water column that ought to be better studied. This can only be achieved through a disciplinary collaboration between researchers of the different relevant fields of expertise.
Author contributions
ZD: Writing—Original Draft, Conceptualization, Data Curation, Visualization; DH: Supervision, Writing—Review and Editing; QH: Supervision, Writing—Review and Editing; CC: Supervision, Writing—Review and Editing.
Acknowledgments
The study was carried out within the framework of the MUDNET academic network: https://www.tudelft.nl/mudnet/.
Conflict of interest
The authors declare that the research was conducted in the absence of any commercial or financial relationships that could be construed as a potential conflict of interest.
Publisher’s note
All claims expressed in this article are solely those of the authors and do not necessarily represent those of their affiliated organizations, or those of the publisher, the editors and the reviewers. Any product that may be evaluated in this article, or claim that may be made by its manufacturer, is not guaranteed or endorsed by the publisher.
References
Adachi, Y., Kobayashi, A., and Kobayashi, M. (2012). Structure of colloidal flocs in relation to the dynamic properties of unstable suspension. Int. J. Polym. Sci. 2012, 1–14. doi:10.1155/2012/574878
Agrawal, Y. C., and Pottsmith, H. C. (2000). Instruments for particle size and settling velocity observations in sediment transport. Mar. Geol. 168, 89–114. doi:10.1016/S0025-3227(00)00044-X
Aly, S. M., and Letey, J. (1988). Polymer and water quality effects on flocculation of montmorillonite. Soil Sci. Soc. Am. J. 52, 1453–1458. doi:10.2136/sssaj1988.03615995005200050047x
Avnimelech, Y., Troeger, B. W., and Reed, L. W. (1982). Mutual flocculation of algae and clay: Evidence and implications. Science 216, 63–65. doi:10.1126/science.216.4541.63
Azetsu-Scott, K., and Passow, U. (2004). Ascending marine particles: Significance of transparent exopolymer particles (TEP) in the upper ocean. Limnol. Oceanogr. 49, 741–748. doi:10.4319/lo.2004.49.3.0741
Bainbridge, Z. T., Wolanski, E., Álvarez-Romero, J. G., Lewis, S. E., and Brodie, J. E. (2012). Fine sediment and nutrient dynamics related to particle size and floc formation in a Burdekin River flood plume, Australia. Mar. Pollut. Bull. 65, 236–248. doi:10.1016/j.marpolbul.2012.01.043
Bar-Or, Y., and Shilo, M. (1988a). “Cyanobacterial flocculants,” in Methods in enzymology (Amsterdam ; New York: Elsevier), 616–622. doi:10.1016/0076-6879(88)67071-6
Bar-Or, Y., and Shilo, M. (1988b). The role of cell-bound flocculants in coflocculation of benthic cyanobacteria with clay particles. FEMS Microbiol. Lett. 53, 169–174. doi:10.1111/j.1574-6968.1988.tb02661.x
F. Bergaya, and G. Lagaly (Editors) (2013). Handbook of clay science. Second edition (Amsterdam: Elsevier).
Biggs, S., Habgood, M., Jameson, G. J., and Yan, Y. (2000). Aggregate structures formed via a bridging flocculation mechanism. Chem. Eng. J. 80, 13–22. doi:10.1016/S1383-5866(00)00072-1
Blanchard, G., Guarini, J., Richard, P., Gros, P., and Mornet, F. (1996). Quantifying the short-term temperature effect on light-saturated photosynthesis of intertidal microphytobenthos. Mar. Ecol. Prog. Ser. 134, 309–313. doi:10.3354/meps134309
Bob, M., and Walker, H. W. (2001). Enhanced adsorption of natural organic matter on calcium carbonate particles through surface charge modification. Colloids Surfaces A Physicochem. Eng. Aspects 191, 17–25. doi:10.1016/S0927-7757(01)00760-9
Bolto, B., and Gregory, J. (2007). Organic polyelectrolytes in water treatment. Water Res. 41, 2301–2324. doi:10.1016/j.watres.2007.03.012
Bowers, D. G., Binding, C. E., and Ellis, K. M. (2007). Satellite remote sensing of the geographical distribution of suspended particle size in an energetic shelf sea. Estuar. Coast. Shelf Sci. 73, 457–466. doi:10.1016/j.ecss.2007.02.005
Brinkmann, B. W., Vonk, J. A., Beusekom, S. A. M., Ibanez, M., Lucas Pardo, M. A., Noordhuis, R., et al. (2019). Benthic hotspots in the pelagic zone: Light and phosphate availability alter aggregates of microalgae and suspended particles in a shallow turbid lake. Limnol. Oceanogr. 64, 585–596. doi:10.1002/lno.11062
Burban, P.-Y., Lick, W., and Lick, J. (1989). The flocculation of fine-grained sediments in estuarine waters. J. Geophys. Res. 94, 8323. doi:10.1029/JC094iC06p08323
Castro, P., and Huber, M. E. (2016). Marine biology. Tenth edition. New York, NY: McGraw-Hill Education.
Chai, C., Yu, Z., Song, X., and Cao, X. (2006). The status and characteristics of eutrophication in the yangtze river (changjiang) estuary and the adjacent east China sea, China. Hydrobiologia 563, 313–328. doi:10.1007/s10750-006-0021-7
Chassagne, C., and Ibanez, M. (2012). Electrophoretic mobility of latex nanospheres in electrolytes: Experimental challenges. Pure Appl. Chem. 85, 41–51. doi:10.1351/PAC-CON-12-02-12
Chassagne, C., Mietta, F., and Winterwerp, J. C. (2009). Electrokinetic study of kaolinite suspensions. J. Colloid Interface Sci. 336, 352–359. doi:10.1016/j.jcis.2009.02.052
Chassagne, C., Safar, Z., Deng, Z., He, Q., and Manning, A. (2021). Flocculation in estuaries: Modeling, laboratory and in-situ studies sediment transport - recent advances (IntechOpen). Available at: https://www.intechopen.com/online-first/flocculation-in-estuaries-modeling-laboratory-and-in-situ-studies (Accessed April 8, 2022).
Chassagne, C., and Safar, Z. (2020). Modelling flocculation: Towards an integration in large-scale sediment transport models. Mar. Geol. 430, 106361. doi:10.1016/j.margeo.2020.106361
Chen, S., Eisma, D., and Kalf, J. (1994). In situ distribution of suspended matter during the tidal cycle in the elbe estuary. Neth. J. Sea Res. 32, 37–48. doi:10.1016/0077-7579(94)90026-4
Colijn, F., and van Buurt, G. (1975). Influence of light and temperature on the photosynthetic rate of marine benthic diatoms. Mar. Biol. 31, 209–214. doi:10.1007/BF00387148
Comte, S., Guibaud, G., and Baudu, M. (2006). Biosorption properties of extracellular polymeric substances (EPS) resulting from activated sludge according to their type: Soluble or bound. Process Biochem. 41, 815–823. doi:10.1016/j.procbio.2005.10.014
Costerton, J. W., Cheng, K., Geesey, G. G., Ladd, T. I., Nickel, J. C., Dasgupta, M., et al. (1987). Bacterial biofilms in nature and disease. Annu. Rev. Microbiol. 41, 435–464. doi:10.1146/annurev.mi.41.100187.002251
Craig, M. J., Baas, J. H., Amos, K. J., Strachan, L. J., Manning, A. J., Paterson, D. M., et al. (2020). Biomediation of submarine sediment gravity flow dynamics. Geology 48, 72–76. doi:10.1130/G46837.1
de Lucas Pardo, M. (2014). Effect of biota on fine sediment transport processes: A study of lake markermeer.
Deng, Z., He, Q., Chassagne, C., and Wang, Z. B. (2021). Seasonal variation of floc population influenced by the presence of algae in the Changjiang (Yangtze river) estuary. Mar. Geol. 440, 106600. doi:10.1016/j.margeo.2021.106600
Deng, Z., He, Q., Safar, Z., and Chassagne, C. (2019). The role of algae in fine sediment flocculation: In-situ and laboratory measurements. Mar. Geol. 413, 71–84. doi:10.1016/j.margeo.2019.02.003
Domozych, D. S. (2007). Exopolymer production by the green alga penium margaritaceum: Implications for biofilm residency. Int. J. Plant Sci. 168, 763–774. doi:10.1086/513606
Dronkers, J., van Leussen, W., Puls, W., Kuehl, H., and Heymann, K. (1988). “Settling velocity of mud flocs: Results of field measurements in the elbe and the weser estuary,” in Physical processes in estuaries. Editors J. Dronkers, and W. van Leussen (Berlin Heidelberg: Springer), 404–424. doi:10.1007/978-3-642-73691-9_20
Droppo, I. G. (2009). Biofilm structure and bed stability of five contrasting freshwater sediments. Mar. Freshw. Res. 60, 690. doi:10.1071/MF08019
Droppo, I. G., D’Andrea, L., Krishnappan, B. G., Jaskot, C., Trapp, B., Basuvaraj, M., et al. (2015). Fine-sediment dynamics: Towards an improved understanding of sediment erosion and transport. J. Soils Sediments 15, 467–479. doi:10.1007/s11368-014-1004-3
Droppo, I. G., Krishnappan, B. G., and Lawrence, J. R. (2016). Microbial interactions with naturally occurring hydrophobic sediments: Influence on sediment and associated contaminant mobility. Water Res. 92, 121–130. doi:10.1016/j.watres.2016.01.034
Droppo, I. G., Leppard, G. G., Flannigan, D. T., and Liss, S. N. (1997). The freshwater floc: A functional relationship of water and organic and inorganic floc constituents affecting suspended sediment properties. Water Air Soil Pollut. 99, 43–53. doi:10.1007/BF02406843
Droppo, I. G., and Ongley, E. D. (1994). Flocculation of suspended sediment in rivers of southeastern Canada. Water Res. 28, 1799–1809. doi:10.1016/0043-1354(94)90253-4
Droppo, I. G., and Ongley, E. D. (1992). The state of suspended sediment in the freshwater fluvial environment: A method of analysis. Water Res. 26, 65–72. doi:10.1016/0043-1354(92)90112-H
Droppo, I. G. (2001). Rethinking what constitutes suspended sediment. Hydrol. Process. 15, 1551–1564. doi:10.1002/hyp.228
Droppo, I. G., Walling, D. E., and Ongley, E. D. (2000). The Influence of floc size, density and porosity on sediment and contaminant transport. Wallingford: IAHS Publication, 141–147.
Dyer, K. R., and Manning, A. J. (1999). Observation of the size, settling velocity and effective density of flocs, and their fractal dimensions. J. Sea Res. 41, 87–95. doi:10.1016/S1385-1101(98)00036-7
Dyer, K. R. (1989). Sediment processes in estuaries: Future research requirements. J. Geophys. Res. 94, 14327. doi:10.1029/JC094iC10p14327
Eisma, D. (1986). Flocculation and de-flocculation of suspended matter in estuaries. Neth. J. Sea Res. 20, 183–199. doi:10.1016/0077-7579(86)90041-4
Eisma, D., and Irion, G. (1993). “Suspended matter and sediment transport,” in Pollution of the north sea. Editors W. Salomons, B. L. Bayne, E. K. Duursma, and U. Förstner (Berlin, Heidelberg: Springer), 20–35. Available at: http://www.springerlink.com/index/10.1007/978-3-642-73709-1_2 (Accessed June 5, 2018).
Eisma, D., and Li, A. (1993). Changes in suspended-matter floc size during the tidal cycle in the dollard estuary. Neth. J. Sea Res. 31, 107–117. doi:10.1016/0077-757w9(93)90001-9
Eisma, D. (1991). Particle size of suspended matter in estuaries. Geo-Marine Lett. 11, 147–153. doi:10.1007/BF02431001
R. D. Evans, J. Wisniewski, and J. R. Wisniewski (Editors) (1997). The interactions between sediments and water (DordrechtNetherlands: Springer). doi:10.1007/978-94-011-5552-6
Fall, K. A., Friedrichs, C. T., Massey, G. M., Bowers, D. G., and Smith, S. J. (2021). The importance of organic content to fractal floc properties in estuarine surface waters: Insights from video, LISST, and pump sampling. J. Geophys. Res. Oceans 126. doi:10.1029/2020JC016787
Fennessy, M. J., Dyer, K. R., and Huntley, D. A. (1994). Inssev: An instrument to measure the size and settling velocity of flocs in situ. Mar. Geol. 117, 107–117. doi:10.1016/0025-3227(94)90009-4
Fettweis, M., and Baeye, M. (2015). Seasonal variation in concentration, size, and settling velocity of muddy marine flocs in the benthic boundary layer. J. Geophys. Res. Oceans 120, 5648–5667. doi:10.1002/2014JC010644
Fettweis, M., and Lee, B. J. (2017). Spatial and seasonal variation of biomineral suspended particulate matter properties in high-turbid nearshore and low-turbid offshore zones. Water 9, 694. doi:10.3390/w9090694
Filippa, L., Freire, L., Trento, A., Álvarez, A. M., Gallo, M., and Vinzón, S. (2011). Laboratory evaluation of two LISST-25X using river sediments. Sediment. Geol. 238, 268–276. doi:10.1016/j.sedgeo.2011.04.017
Foree, E. G., and McCarty, P. L. (1970). Anaerobic decomposition of algae. Environ. Sci. Technol. 4, 842–849. doi:10.1021/es60045a005
Fox, J. M., Hill, P. S., Milligan, T. G., and Boldrin, A. (2004). Flocculation and sedimentation on the po river delta. Mar. Geol. 203, 95–107. doi:10.1016/S0025-3227(03)00332-3
Fugate, D. C., and Friedrichs, C. T. (2003). Controls on suspended aggregate size in partially mixed estuaries. Estuar. Coast. Shelf Sci. 58, 389–404. doi:10.1016/S0272-7714(03)00107-0
Geyer, W. R., Hill, P. S., and Kineke, G. C. (2004). The transport, transformation and dispersal of sediment by buoyant coastal flows. Cont. Shelf Res. 24, 927–949. doi:10.1016/j.csr.2004.02.006
Gibbs, R. J. (1985). Estuarine flocs: Their size, settling velocity and density. J. Geophys. Res. 90, 3249. doi:10.1029/jc090ic02p03249
Goldberg, S. (1991). Flocculation of illite/kaolinite and illite/montmorillonite mixtures as affected by sodium adsorption ratio and pH. Clays Clay Minerals 39, 375–380. doi:10.1346/CCMN.1991.0390406
Gratiot, N., and Manning, A. J. (2004). An experimental investigation of floc characteristics in a diffusive turbulent flow. J. Coast. Res. 41, 105–113.
Gregory, J., and Barany, S. (2011). Adsorption and flocculation by polymers and polymer mixtures. Adv. Colloid Interface Sci. 169, 1–12. doi:10.1016/j.cis.2011.06.004
Gregory, J. (2005). Particles in water: Properties and processes. 0 ed. Boca Raton: CRC Press. doi:10.1201/9780203508459
Grossart, H.-P., Kiorboe, T., Tang, K., and Ploug, H. (2003). Bacterial colonization of particles growth and interactions. Appl. Environ. Microbiol. 69, 3500–3509. doi:10.1128/AEM.69.6.3500-3509.2003
Guan, X., Chen, Y., and Du, X. (1996). Experimental study on mechanism of flocculation in yangtze estuary (in Chinese). J. Hydraulic Eng. 1, 70–80.
Guenther, M., and Bozelli, R. (2004). Factors influencing algae–clay aggregation. Hydrobiologia 523, 217–223. doi:10.1023/B:HYDR.0000033127.05034.32
Guo, C. (2018). Cohesive sediment flocculation and settling processes and the controlling mechanisms.
Guo, C., He, Q., Guo, L., and Winterwerp, J. C. (2017). A study of in-situ sediment flocculation in the turbidity maxima of the yangtze estuary. Estuar. Coast. Shelf Sci. 191, 1–9. doi:10.1016/j.ecss.2017.04.001
Guo, L., and He, Q. (2011). Freshwater flocculation of suspended sediments in the Yangtze River, China. Ocean. Dyn. 61, 371–386. doi:10.1007/s10236-011-0391-x
Heath, A. R., Bahri, P. A., Fawell, P. D., and Farrow, J. B. (2006). Polymer flocculation of calcite: Population balance model. AIChE J. 52, 1641–1653. doi:10.1002/aic.10749
Hicks, R. F. (1988). Sediment rafting: A novel mechanism for the small-scale dispersal of intertidal estuarine meiofauna. Mar. Ecol. Prog. Ser. 48, 69–80. doi:10.3354/meps048069
Higgins, M. J., and Novak, J. T. (1997). The effect of cations on the settling and dewatering of activated sludges: Laboratory results. Water Environ. Res. 69, 215–224. doi:10.2175/106143097X125371
Ho, Q. N., Fettweis, M., Spencer, K. L., and Lee, B. J. (2022). Flocculation with heterogeneous composition in water environments: A review. Water Res. 213, 118147. doi:10.1016/j.watres.2022.118147
Horemans, D. M. L., Dijkstra, Y. M., Schuttelaars, H. M., Sabbe, K., Vyverman, W., Meire, P., et al. (2021). Seasonal variations in flocculation and erosion affecting the large‐scale suspended sediment distribution in the scheldt estuary: The importance of biotic effects. J. Geophys. Res. Oceans 126. doi:10.1029/2020JC016805
Hu, K., Ding, P., Wang, Z., and Yang, S. (2009). A 2D/3D hydrodynamic and sediment transport model for the yangtze estuary, China. J. Mar. Syst. 77, 114–136. doi:10.1016/j.jmarsys.2008.11.014
Hunt, J. R. (1980). “Prediction of oceanic particle size distributions from coagulation and sedimentation mechanisms,” in Particulates in water advances in chemistry. Editors M. C. Kavanaugh, and J. O. Leckie (WASHINGTON, D. C.: American Chemical Society), 243–257. doi:10.1021/ba-1980-0189.ch011
Hunter, K. A., and Liss, P. S. (1979). The surface charge of suspended particles in estuarine and coastal waters. Nature 282, 823–825. doi:10.1038/282823a0
Ibanez Sanz, M. E. (2018). Flocculation and consolidation of cohesive sediments under the influence of coagulant and flocculant. doi:10.4233/UUID:6E96DB66-1DF0-4ED1-B343-92939D58D864
Jarvis, P., Jefferson, B., Gregory, J., and Parsons, S. A. (2005). A review of floc strength and breakage. Water Res. 39, 3121–3137. doi:10.1016/j.watres.2005.05.022
Jeldres, R. I., Fawell, P. D., and Florio, B. J. (2018). Population balance modelling to describe the particle aggregation process: A review. Powder Technol. 326, 190–207. doi:10.1016/j.powtec.2017.12.033
Jiang, G., Yao, Y., and Tang, Z. (2002). Analysis of influencing factors on fine sediment flocculation in the changjiang estuary. Acta Oceanol. Sin. 21, 385–394.
Jonas, P. J. C., and Millward, G. E. (2010). Metals and nutrients in the severn estuary and bristol channel: Contemporary inputs and distributions. Mar. Pollut. Bull. 61, 52–67. doi:10.1016/j.marpolbul.2009.12.013
Jorand, F., Zartarian, F., Thomas, F., Block, J. C., Bottero, J. Y., Villemin, G., et al. (1995). Chemical and structural (2D) linkage between bacteria within activated sludge flocs. Water Res. 29, 1639–1647. doi:10.1016/0043-1354(94)00350-G
Kiemle, S. N., Domozych, D. S., and Gretz, M. R. (2007). The extracellular polymeric substances of desmids (conjugatophyceae, streptophyta): Chemistry, structural analyses and implications in wetland biofilms. Phycologia 46, 617–627. doi:10.2216/06-97.1
Kiørboe, T., Andersen, K. P., and Dam, H. G. (1990). Coagulation efficiency and aggregate formation in marine phytoplankton. Mar. Biol. 107, 235–245. doi:10.1007/BF01319822
Kosmulski, M., and Dahlsten, P. (2006). High ionic strength electrokinetics of clay minerals. Colloids Surfaces A Physicochem. Eng. Aspects 291, 212–218. doi:10.1016/j.colsurfa.2006.06.037
Kranenburg, C. (1994). The fractal structure of cohesive sediment aggregates. Estuar. Coast. Shelf Sci. 39, 451–460. doi:10.1016/S0272-7714(06)80002-8
Kruyt, H. R., Jonker, G., and Overbeek, J. (1952). “Colloid science,” in Irreversible systems (Amsterdam ; New York: Elsevier Publishing Co), Vol. 1.
Kumar, R. G., Strom, K. B., and Keyvani, A. (2010). Floc properties and settling velocity of San Jacinto estuary mud under variable shear and salinity conditions. Cont. Shelf Res. 30, 2067–2081. doi:10.1016/j.csr.2010.10.006
Lai, H., Fang, H., Huang, L., He, G., and Reible, D. (2018). A review on sediment bioflocculation: Dynamics, influencing factors and modeling. Sci. Total Environ. 642, 1184–1200. doi:10.1016/j.scitotenv.2018.06.101
Langmuir, I. (1918). The adsorption of gases on plane surface of glass, mica and platinum. J. Am. Chem. Soc. 40, 1361–1403. doi:10.1021/ja02242a004
Lee, B. J., Toorman, E., and Fettweis, M. (2014). Multimodal particle size distributions of fine-grained sediments: Mathematical modeling and field investigation. Ocean. Dyn. 64, 429–441. doi:10.1007/s10236-014-0692-y
Lee, B. J., Toorman, E., Molz, F. J., and Wang, J. (2011). A two-class population balance equation yielding bimodal flocculation of marine or estuarine sediments. Water Res. 45, 2131–2145. doi:10.1016/j.watres.2010.12.028
Leppard, G. G. (1992). Size, morphology and composition of particulates in aquatic ecosystems: Solving speciation problems by correlative electron microscopy. Analyst 117, 595. doi:10.1039/an9921700595
Li, D.-H., and Ganczarczyk, J. J. (1990). Structure of activated sludge floes. Biotechnol. Bioeng. 35, 57–65. doi:10.1002/bit.260350109
Li, H.-M., Tang, H.-J., Shi, X.-Y., Zhang, C.-S., and Wang, X.-L. (2014). Increased nutrient loads from the changjiang (yangtze) river have led to increased harmful algal blooms. Harmful Algae 39, 92–101. doi:10.1016/j.hal.2014.07.002
Lick, W., Huang, H., and Jepsen, R. (1993). Flocculation of fine-grained sediments due to differential settling. J. Geophys. Res. 98, 10279. doi:10.1029/93jc00519
Lick, W., and Lick, J. (1988). Aggregation and disaggregation of fine-grained lake sediments. J. Gt. Lakes. Res. 14, 514–523. doi:10.1016/S0380-1330(88)71583-X
Liu, Q., Li, J., Dai, Z., and Li, D. (2007). Flocculation process of fine-grained sediments by the combined effect of salinity and humus in the changjiang estuary. ACTA Oceanol. SINICA-ENGLISH Ed. 26, 140.
Logan, B. E., and Hunt, J. R. (1987). Advantages to microbes of growth in permeable aggregates in marine systems 1: Growth in permeable aggregates. Limnol. Oceanogr. 32, 1034–1048. doi:10.4319/lo.1987.32.5.1034
Lupi, F. M., Fernandes, H. M. L., Sá-Correia, I., and Novais, J. M. (1991). Temperature profiles of cellular growth and exopolysaccharide synthesis by Botryococus braunii Kütz. UC 58. J. Appl. Phycol. 3, 35–42. doi:10.1007/BF00003917
Maggi, F. (2013). The settling velocity of mineral, biomineral, and biological particles and aggregates in water. J. Geophys. Res. Oceans 118, 2118–2132. doi:10.1002/jgrc.20086
Manning, A., Baugh, J., Soulsby, R., Spearman, J., and Whitehouse, R. (2011). Cohesive sediment flocculation and the application to settling flux modelling. Sediment Transport, 91–116.
Manning, A. J., Baugh, J. V., Spearman, J. R., and Whitehouse, R. J. S. (2010a). Flocculation settling characteristics of mud: Sand mixtures. Ocean. Dyn. 60, 237–253. doi:10.1007/s10236-009-0251-0
Manning, A. J., and Dyer, K. R. (1999). A laboratory examination of floc characteristics with regard to turbulent shearing. Mar. Geol. 160, 147–170. doi:10.1016/s0025-3227(99)00013-4
Manning, A. J., Friend, P. L., Prowse, N., and Amos, C. L. (2007). Estuarine mud flocculation properties determined using an annular mini-flume and the LabSFLOC system. Cont. Shelf Res. 27, 1080–1095. doi:10.1016/j.csr.2006.04.011
Manning, A. J., Langston, W. J., and Jonas, P. J. C. (2010b). A review of sediment dynamics in the severn estuary: Influence of flocculation. Mar. Pollut. Bull. 61, 37–51. doi:10.1016/j.marpolbul.2009.12.012
Markussen, T. N., and Andersen, T. J. (2014). Flocculation and floc break-up related to tidally induced turbulent shear in a low-turbidity, microtidal estuary. J. Sea Res. 89, 1–11. doi:10.1016/j.seares.2014.02.001
McAnally, W. H., and Mehta, A. J. (2001). Coastal and estuarine fine sediment processes. Amsterdam ; New York: Elsevier.
McCave, I. N. (1984). Size spectra and aggregation of suspended particles in the deep ocean. Deep Sea Res. Part A. Oceanogr. Res. Pap. 31, 329–352. doi:10.1016/0198-0149(84)90088-8
Mehta, A. J., and Partheniades, E. (1975). An investigation of the depositional properties of flocculated fine sediments. J. Hydraulic Res. 13, 361–381. doi:10.1080/00221687509499694
Mhashhash, A., Bockelmann-Evans, B., and Pan, S. (2018). Effect of hydrodynamics factors on sediment flocculation processes in estuaries. J. Soils Sediments 18, 3094–3103. doi:10.1007/s11368-017-1837-7
Michels, J., Stippkugel, A., Lenz, M., Wirtz, K., and Engel, A. (2018). Rapid aggregation of biofilm-covered microplastics with marine biogenic particles. Proc. R. Soc. B 285, 20181203. doi:10.1098/rspb.2018.1203
Mietta, F., Chassagne, C., Manning, A. J., and Winterwerp, J. C. (2009a). Influence of shear rate, organic matter content, pH and salinity on mud flocculation. Ocean. Dyn. 59, 751–763. doi:10.1007/s10236-009-0231-4
Mietta, F., Chassagne, C., and Winterwerp, J. C. (2009b). Shear-induced flocculation of a suspension of kaolinite as function of pH and salt concentration. J. Colloid Interface Sci. 336, 134–141. doi:10.1016/j.jcis.2009.03.044
Mikkelsen, L. (2002a). The shear sensitivity of activated sludge: An evaluation of the possibility for a standardised floc strength test. Water Res. 36, 2931–2940. doi:10.1016/S0043-1354(01)00518-8
Mikkelsen, O. A. (2002b). Examples of spatial and temporal variations of some fine-grained suspended particle characteristics in two Danish coastal water bodies. Oceanol. Acta 25, 39–49. doi:10.1016/s0399-1784(01)01175-6
Mikkelsen, O., and Pejrup, M. (2001). The use of a LISST-100 laser particle sizer for in-situ estimates of floc size, density and settling velocity. Geo-Marine Lett. 20, 187–195. doi:10.1007/s003670100064
Mikutta, R., Mikutta, C., Kalbitz, K., Scheel, T., Kaiser, K., and Jahn, R. (2007). Biodegradation of forest floor organic matter bound to minerals via different binding mechanisms. Geochimica Cosmochimica Acta 71, 2569–2590. doi:10.1016/j.gca.2007.03.002
Nabzar, L., Pefferkorn, E., and Varoqui, R. (1988). Stability of polymer—clay suspensions. The polyacrylamide—sodium kaolinite system. Colloids Surfaces 30, 345–353. doi:10.1016/0166-6622(88)80217-8
Otsubo, Y. (1992). Effect of particle size on the bridging structure and elastic properties of flocculated suspensions. J. Colloid Interface Sci. 153, 584–586. doi:10.1016/0021-9797(92)90350-U
Park, C., Fang, Y., Murthy, S. N., and Novak, J. T. (2010). Effects of floc aluminum on activated sludge characteristics and removal of 17-α-ethinylestradiol in wastewater systems. Water Res. 44, 1335–1340. doi:10.1016/j.watres.2009.11.002
Partheniades, E. (1991). “Effect of bed shear stresses on the deposition and strength of deposited cohesive muds,” in Microstructure of fine-grained sediments: From mud to shale. R. H. Bennett, W. R. Bryant, M. H. Hulbert, W. A. Chiou, R. W. Faas, J. Kasprowiczet al. (New York, NY: Springer New York), 175–183. Available at:. doi:10.1007/978-1-4612-4428-8₁8
Partheniades, E. (1993). “Turbulence, flocculation and cohesive sediment dynamics,” in Coastal and Estuarine Studies. Editors A. J. Mehta (Washington, D. C.: American Geophysical Union), 40–59. doi:10.1029/CE042p0040
Passow, U., and De La Rocha, C. L. (2006). Accumulation of mineral ballast on organic aggregates: Mineral ballast and organic aggregates. Glob. Biogeochem. Cycles 20. doi:10.1029/2005GB002579
Passow, U. (2002). Transparent exopolymer particles (TEP) in aquatic environments. Prog. Oceanogr. 55, 287–333. doi:10.1016/S0079-6611(02)00138-6
Pearson, H. W., Mara, D. D., Mills, S. W., and Smallman, D. J. (1987). Physico-chemical parameters influencing faecal bacterial survival in waste stabilization ponds. Water Sci. Technol. 19, 145–152. doi:10.2166/wst.1987.0139
Petrova, O. E., and Sauer, K. (2012). Sticky situations: Key components that control bacterial surface attachment. J. Bacteriol. 194, 2413–2425. doi:10.1128/JB.00003-12
Plude, J. L., Parker, D. L., Schommer, O. J., Timmerman, R. J., Hagstrom, S. A., Joers, J. M., et al. (1991). Chemical characterization of polysaccharide from the slime layer of the cyanobacterium microcystis flos-aquae C3-40. Appl. Environ. Microbiol. 57, 1696–1700. doi:10.1128/AEM.57.6.1696-1700.1991
Porter, K. G. (1973). Selective grazing and differential digestion of algae by zooplankton. Nature 244, 179–180. doi:10.1038/244179a0
Rand, B., and Melton, I. E. (1977). Particle interactions in aqueous kaolinite suspensions. J. Colloid Interface Sci. 60, 308–320. doi:10.1016/0021-9797(77)90290-9
Riley, G. A. (1963). Organic aggregates in seawater and the dynamics of their formation and utilization. Limnol. Oceanogr. 8, 372–381. doi:10.4319/lo.1963.8.4.0372
Rosen, M. J. (2004). Surfactants and interfacial phenomena. Hoboken, NJ, USA: John Wiley & Sons. doi:10.1002/0471670561
Safar, Z., Chassagne, C., Rijnsburger, S., Sanz, M. I., Manning, A. J., Souza, A. J., et al. (2022). Characterization and classification of estuarine suspended particles based on their inorganic/organic matter composition. Front. Mar. Sci. 9, 896163. doi:10.3389/fmars.2022.896163
Safar, Z. (2022). Suspended particulate matter formation and accumulation in the delta: From monitoring to modelling. doi:10.4233/UUID:17D027D9-9667-4AFA-89B9-AACD557A41AC
Sahin, C. (2014). Investigation of the variability of floc sizes on the Louisiana Shelf using acoustic estimates of cohesive sediment properties. Mar. Geol. 353, 55–64. doi:10.1016/j.margeo.2014.03.022
Serra, T., and Casamitjana, X. (1998). Structure of the aggregates during the process of aggregation and breakup under a shear flow. J. Colloid Interface Sci. 206, 505–511. doi:10.1006/jcis.1998.5714
Serra, T., Colomer, J., and Logan, B. E. (2008). Efficiency of different shear devices on flocculation. Water Res. 42, 1113–1121. doi:10.1016/j.watres.2007.08.027
Shakeel, A., Safar, Z., Ibanez, M., van Paassen, L., and Chassagne, C. (2020). Flocculation of clay suspensions by anionic and cationic polyelectrolytes: A systematic analysis. Minerals 10, 999. doi:10.3390/min10110999
Shao, Y., Yan, Y., and Maa, J. P.-Y. (2011). In situ measurements of settling velocity near baimao shoal in changjiang estuary. J. Hydraul. Eng. 137, 372–380. doi:10.1061/(ASCE)HY.1943-7900.0000312
Sheng, G.-P., Yu, H.-Q., and Li, X.-Y. (2010). Extracellular polymeric substances (EPS) of microbial aggregates in biological wastewater treatment systems: A review. Biotechnol. Adv. 28, 882–894. doi:10.1016/j.biotechadv.2010.08.001
Sherman, I. (1953). Flocculent structure of sediment suspended in Lake Mead. Trans. AGU. 34, 394. doi:10.1029/TR034i003p00394
Simon, M., Grossart, H.-P., Schweitzer, B., and Ploug, H. (2002). Microbial ecology of organic aggregates in aquatic ecosystems. Aquat. Microb. Ecol. 28, 175–211. doi:10.3354/ame028175
Sobeck, D. C., and Higgins, M. J. (2002). Examination of three theories for mechanisms of cation-induced bioflocculation. Water Res. 36, 527–538. doi:10.1016/S0043-1354(01)00254-8
Son, M., and Hsu, T.-J. (2009). The effect of variable yield strength and variable fractal dimension on flocculation of cohesive sediment. Water Res. 43, 3582–3592. doi:10.1016/j.watres.2009.05.016
Sondi, I., Bišćan, J., and Pravdić, V. (1996). Electrokinetics of pure clay minerals revisited. J. Colloid Interface Sci. 178, 514–522. doi:10.1006/jcis.1996.0146
Spicer, P. T., and Pratsinis, S. E. (1996). Coagulation and fragmentation: Universal steady-state particle-size distribution. AIChE J. 42, 1612–1620. doi:10.1002/aic.690420612
Swenson, J., Smalley, M. V., and Hatharasinghe, H. L. M. (1998). Mechanism and strength of polymer bridging flocculation. Phys. Rev. Lett. 81, 5840–5843. doi:10.1103/PhysRevLett.81.5840
Tang, J. (2007). Characteristics of fine cohesive sediment’s flocculation in the Changjiang estuary and its adjacent sea area.
Taylor, M. L., Gwinnett, C., Robinson, L. F., and Woodall, L. C. (2016). Plastic microfibre ingestion by deep-sea organisms. Sci. Rep. 6, 33997. doi:10.1038/srep33997
Thill, A., Moustier, S., Garnier, J.-M., Estournel, C., Naudin, J.-J., and Bottero, J.-Y. (2001). Evolution of particle size and concentration in the Rhône river mixing zone. Cont. Shelf Res. 21, 2127–2140. doi:10.1016/S0278-4343(01)00047-4
Thomas, D. N., Judd, S. J., and Fawcett, N. (1999). Flocculation modelling: A review. Water Res. 33, 1579–1592. doi:10.1016/S0043-1354(98)00392-3
Tsai, C., and Hwang, S. (1995). Flocculation of sediment from the tanshui River Estuary. Mar. Freshw. Res. 46, 383. doi:10.1071/MF9950383
Tsujimoto, Y., Chassagne, C., and Adachi, Y. (2013). Comparison between the electrokinetic properties of kaolinite and montmorillonite suspensions at different volume fractions. J. Colloid Interface Sci. 407, 109–115. doi:10.1016/j.jcis.2013.05.080
Uncles, R. J., Bale, A. J., Stephens, J. A., Frickers, P. E., and Harris, C. (2010). Observations of floc sizes in a muddy estuary. Estuar. Coast. Shelf Sci. 87, 186–196. doi:10.1016/j.ecss.2009.12.018
van der Lee, W. T. B. (2000). Temporal variation of floc size and settling velocity in the dollard estuary. Cont. Shelf Res. 20, 1495–1511. doi:10.1016/S0278-4343(00)00034-0
van Leussen, W. (1988). “Aggregation of particles, settling velocity of mud flocs A review,” in Physical processes in estuaries. Editors J. Dronkers, and W. van Leussen (Berlin, Heidelberg: Springer Berlin Heidelberg), 347–403. doi:10.1007/978-3-642-73691-9_19
van Leussen, W. (1994). Estuarine macroflocs and their role in fine-grained sediment transport. Available at: https://ci.nii.ac.jp/naid/10024267924/(Accessed June 7, 2018).
van Leussen, W. (1997). The Kolmogorov microscale as a limiting value for the floc sizes of suspended fine-grained sediments in estuaries. Cohesive sediments, 45–62.
Verney, R., Lafite, R., Claude Brun-Cottan, J., and Le Hir, P. (2011). Behaviour of a floc population during a tidal cycle: Laboratory experiments and numerical modelling. Cont. Shelf Res. 31, S64–S83. doi:10.1016/j.csr.2010.02.005
Walsby, A. E. (1968). Mucilage secretion and the movements of blue-green algae. Protoplasma 65, 223–238. doi:10.1007/BF01666380
Wan, Y., Wu, H., Roelvink, D., and Gu, F. (2015). Experimental study on fall velocity of fine sediment in the yangtze estuary, China. Ocean. Eng. 103, 180–187. doi:10.1016/j.oceaneng.2015.04.076
Wang, Y. P., Voulgaris, G., Li, Y., Yang, Y., Gao, J., Chen, J., et al. (2013). Sediment resuspension, flocculation, and settling in a macrotidal estuary: Flocculation and settling in estuary. J. Geophys. Res. Oceans 118, 5591–5608. doi:10.1002/jgrc.20340
Wilén, B.-M., Lund Nielsen, J., Keiding, K., and Nielsen, P. H. (2000). Influence of microbial activity on the stability of activated sludge flocs. Colloids Surfaces B Biointerfaces 18, 145–156. doi:10.1016/S0927-7765(99)00138-1
Winterwerp, J. C. (1998). A simple model for turbulence induced flocculation of cohesive sediment. J. Hydraulic Res. 36, 309–326. doi:10.1080/00221689809498621
Winterwerp, J. C., Manning, A. J., Martens, C., de Mulder, T., and Vanlede, J. (2006). A heuristic formula for turbulence-induced flocculation of cohesive sediment. Estuar. Coast. Shelf Sci. 68, 195–207. doi:10.1016/j.ecss.2006.02.003
Winterwerp, J. C. (2002). On the flocculation and settling velocity of estuarine mud. Cont. Shelf Res. 22, 1339–1360. doi:10.1016/S0278-4343(02)00010-9
Winterwerp, J. C., and Van Kesteren, W. G. M. (2004). Introduction to the physics of cohesive sediment dynamics in the marine environment. Burlington: Elsevier Science. Available at: http://www.123library.org/book_details/?id=33664 (Accessed April 4, 2021).
Winterwerp, J., and Kesteren, W. V. (2004). Introduction to the physics of cohesive sediment in the marine environment.
Wood, E. J. F. (2014). Microbiology of oceans and estuaries. Amsterdam: Elsevier Science. Available at: http://qut.eblib.com.au/patron/FullRecord.aspx?p=404785 (Accessed July 29, 2020).
Xia, X. M., Li, Y., Yang, H., Wu, C. Y., Sing, T. H., and Pong, H. K. (2004). Observations on the size and settling velocity distributions of suspended sediment in the pearl river estuary, China. Cont. Shelf Res. 24, 1809–1826. doi:10.1016/j.csr.2004.06.009
Yeung, A. K. C., and Pelton, R. (1996). Micromechanics: A new approach to studying the strength and breakup of flocs. J. Colloid Interface Sci. 184, 579–585. doi:10.1006/jcis.1996.0654
Yokoi, H. (2002). Flocculation properties of pectin in various suspensions. Bioresour. Technol. 84, 287–290. doi:10.1016/S0960-8524(02)00023-8
Yokoi, H., Natsuda, O., Hirose, J., Hayashi, S., and Takasaki, Y. (1995). Characteristics of a biopolymer flocculant produced by Bacillus sp. PY-90. J. Ferment. Bioeng. 79, 378–380. doi:10.1016/0922-338X(95)94000-H
Zhang, J., Zhang, Q., Maa, J. P. Y., and Qiao, G. (2013). Lattice Boltzmann simulation of turbulence-induced flocculation of cohesive sediment. Ocean. Dyn. 63, 1123–1135. doi:10.1007/s10236-013-0646-9
Zhang, Z. (1996). Studies on basic characteristics of fine sediment in changjiang estuary (in Chinese). J. Sediment Res. 1, 67–73.
Zinkevich, V., Bogdarina, I., Kang, H., Hill, M. A. W., Tapper, R., and Beech, I. B. (1996). Characterisation of exopolymers produced by different isolates of marine sulphate-reducing bacteria. Int. Biodeterior. Biodegrad. 37, 163–172. doi:10.1016/S0964-8305(96)00025-X
Keywords: sediment flocculation, bioflocculation, algae, extracellular polymeric substances (EPS), aggregation
Citation: Deng Z, Huang D, He Q and Chassagne C (2022) Review of the action of organic matter on mineral sediment flocculation. Front. Earth Sci. 10:965919. doi: 10.3389/feart.2022.965919
Received: 10 June 2022; Accepted: 06 September 2022;
Published: 26 September 2022.
Edited by:
Omid Haeri-Ardakani, Department of Natural Resources, CanadaReviewed by:
Hadar Elyashiv, Ben-Gurion University of the Negev, IsraelDiana Cuadrado, CONICET Bahía Blanca, Argentina
Copyright © 2022 Deng, Huang, He and Chassagne. This is an open-access article distributed under the terms of the Creative Commons Attribution License (CC BY). The use, distribution or reproduction in other forums is permitted, provided the original author(s) and the copyright owner(s) are credited and that the original publication in this journal is cited, in accordance with accepted academic practice. No use, distribution or reproduction is permitted which does not comply with these terms.
*Correspondence: Dong Huang, Z3Jpd2hAZm94bWFpbC5jb20=