- 1Philippine Institute of Volcanology and Seismology-Department of Science and Technology (PHIVOLCS-DOST), Quezon City, Philippines
- 2Tokyo Metropolitan College of Industrial Technology, Tokyo, Japan
- 3Tokyo Institute of Technology, Tokyo, Japan
Accelerated creep, primarily through vertical displacement, has been occurring along 15 en-echelon faults belonging to a 15 km-long and 1.5 km-wide, N-S-trending dilational jog of the active West Valley Fault (or West Marikina Valley Fault), on the southeastern part of Metro Manila, Philippines. The much-larger-than-known tectonic slip rates had been the only reliable evidence in support of excessive groundwater withdrawal as the trigger of creep in the 1990s. Recently available groundwater extraction data (1977–2019) could more directly and consistently link groundwater withdrawal to accelerated creep in the 1990s. Twenty years (1999–2019) of precise displacement measurements could also reveal significant spatial and temporal links between slip rate changes and patterns of groundwater extraction. Our analysis shows that greater rates of vertical displacement are related to the timing of faster extraction rates. Variations in slip rates between the northern and southern measurement sites are primarily due to regional differences in groundwater extraction, which are influenced by differences in the implementation of water extraction reduction regulations. Proximity to the key source of groundwater recharge (Laguna Lake) is also an influencing factor. Although there are many unknowns inherent to this type of study, continued depressurization could induce static stress changes that could modulate the timescale of earthquake occurrence due to the natural course of stress loading driven by regional tectonics. The current and potential effects of continued depressurization in the areas with high slip rates and surrounding regions are paramount considerations in crafting and implementing tighter and extended groundwater extraction regulations.
Introduction
Creep has been occurring along 15 NE-SW-trending faults arranged en-echelon within a 15 km-long and 1.5 km-wide segment of the West Valley Fault (WVF). This segment corresponds to the dilational gap between the right-stepping segments of the WVF, which is an active dextral strike-slip fault that cuts through the eastern portion of Metro Manila in Luzon Island, Philippines (Figure 1A; Punongbayan and Rimando, 1996; Rimando, 2002; Rimando and Knuepfer, 2006). Previous researchers (Punongbayan and Rimando, 1996; Rimando et al., 2000; Rimando, 2002) identified groundwater withdrawal as the more likely trigger of the accelerated creep primarily because of the high rates of slip recorded in the 1990s, which are more than the known tectonic creep rates (Ambraseys, 1970; Savage et al., 1979; Burford and Harsh, 1980; Yu and Liu, 1989; Lienkaemper et al., 1991; Kelson et al., 1996; Stenner et al., 2000). Similar structurally controlled creeping structures associated with groundwater withdrawal had been documented in Xian City (Kaizuka et al., 1992; Matsuda et al.,1986) in the Weihe Basin of China. Compared to other tectonic and nontectonic creeping ruptures, the Sucat–Biñan creeping zone is unique in terms of pattern, style, and creep rate.
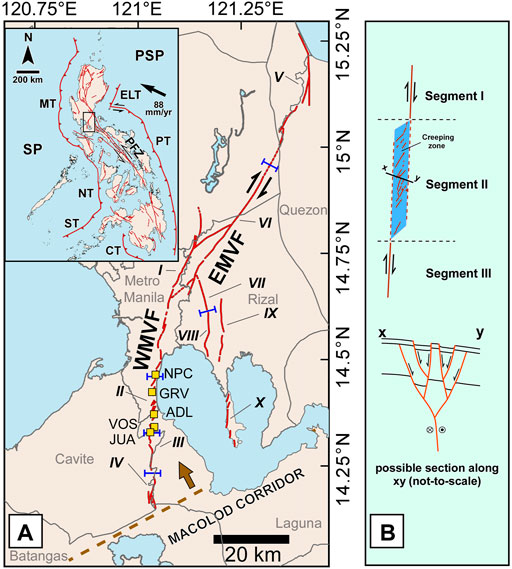
FIGURE 1. (A) Regional setting of the study area showing the major structures (inset). SP—Sunda Plate, PSP—Philippine Sea Plate, MT—Manila Trench, NT—Negros Trench, ST—Sulu Trench, CT—Cotabato Trench, ELT—East Luzon Trough, PT—Philippine Trench, and PFZ—Philippine Fault Zone (http://faultfinder.phivolcs.dost.gov.ph/). The creeping segment lies along the West Valley Fault (WVF) of the Marikina Valley Fault System (MVFS) (bounded by a black rectangle in Figure 1A). Survey sites are shown (yellow boxes labeled as NPC, GRV, ADL, VOS, and JUA, which are abbreviations for the National Power Corporation, Gruenville Subdivision, Adelina I Subdivision, Villa Olympia Subdivision, and Doña Juana Subdivision, respectively). Segments of the MVFS are indicated by roman numerals; the boundaries of these segments are indicated by blue bars. The northern limit of the Macolod Corridor (MC) is indicated by brown dashed lines, and the direction of extension of the MC is indicated by the brown arrow. (B) Schematic diagram of segment II and adjacent segments I and III (top). The dilational jog between echelon segments I and II favor the development of a negative flower structure (bottom).
Although groundwater withdrawal was cited as the more likely trigger for creep, this was inconsistent with the timing of the groundwater level and vertical slip rate declines in the 1990s (Rimando, 2002). Significant groundwater level declines in the 1990s also postdate the supposed pre-1990s creep initiation. Part of the reason could be the limited number of sites from which groundwater levels were interpolated and the long monitoring intervals. We determine possible spatio-temporal links between vertical slip rates and groundwater extraction from a variety of aquifer depths over a longer period of time, by comparing the 1999 to 2019 displacements and slip rates along with those from the 1990s with groundwater extraction from 1977 to 2019. The 1999–2019 slip rates are estimated from creep displacements obtained from several sites within the creeping zone (labeled NPC, GRV, ADL, VOS, and JUA, which are abbreviations for the National Power Corporation, Gruenville Subdivision, Adelina I Subdivision, Villa Olympia Subdivision, and Doña Juana Subdivision, respectively, in Figures 1A; Figure 2) from 1999 to 2019. Other parameters that influence temporal and spatial variations in slip rates (i.e., water regulatory interventions and proximity to the source of recharge) are included in the analysis.
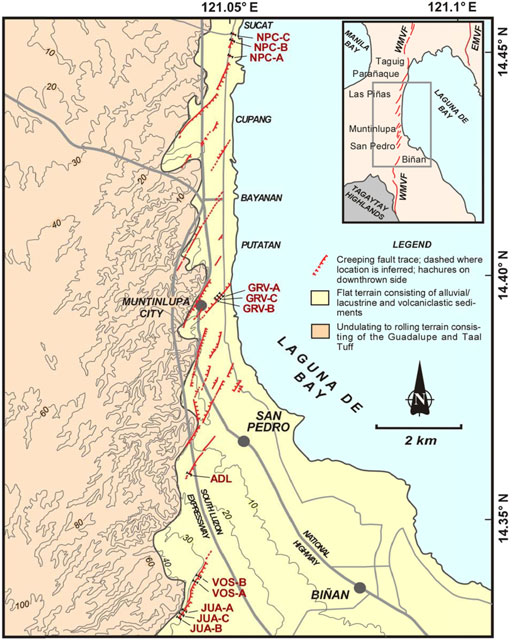
FIGURE 2. More detailed map of the WVF creeping zone showing the location of the survey sites and the 12 survey lines (NPC-A, NPC-B, NPC-C, GRV-A, GRV-B, GRV-C, ADL, VOS-A, VOS-B, JUA-A, JUA-B, and JUA-C) that have been established at different times. As of this study, four (4) of these survey lines (NPC-A, NPC-B, NPC-C, and GRV-A) no longer operate. Simplified lithologies of the study area are also shown. Groundwater reservoirs in the study area include the Guadalupe Formation, Taal Tuff, and the more recent sediments adjacent to and underneath the lake.
Knowledge of any spatial and temporal link between accelerated creep and the amount and rate of groundwater extraction is critical from a seismic hazard standpoint. Many fault movements, believed to be induced or triggered by groundwater extraction and other human activities, have resulted in seismicity (Foulger et al., 2018; Wilson et al., 2017). Many theories explain the mechanism of fault failure and earthquake generation by fluid extraction in the numerous documented cases worldwide (Foulger et al., 2018; Wu, 2021). Earthquakes may also result from creep (Pennington et al., 1986) and is related to the development of resistors to slip. These features are akin to barriers and asperities (Scholz and Engelder, 1976; Aki, 1979; Lay et al., 1982) which may be expressed as step-overs and bends along the surface trace of a fault. The results of this study could be useful guides in crafting and implementing groundwater extraction regulations to avoid further damage from accelerated aseismic fault creep and potential induced seismicity within the creeping zone and along the adjacent segments of the West Valley Fault.
Geological and structural background
The creeping zone in the southeastern part of Metro Manila corresponds to segment II of the West Valley Fault (WVF; Rimando, 2002; Rimando and Knuepfer, 2006), which along with the East Valley Fault (EVF), comprises the two major active segments of the Valley Fault System (VFS; also called the Marikina Valley Fault System or MVFS; Arcilla et al., 1989; Rimando, 2002; Rimando and Knuepfer, 2006). North of the creeping segment is the WVF’s 45 km segment I (Figure 1), which has the potential of generating a MW 7 earthquake based on length (Rimando, 2002; Rimando and Knuepfer 2006) and paleoseismicity (Nelson et al., 2000) or MW 7.7 based on the most recent, single-event scarp offsets (Rimando, 2002; Rimando and Knuepfer, 2006). According to Nelson et al. (2000), the return period of earthquakes along this segment is 400–600 years. The zone of creeping echelon segments fills the dilational gap between the right-stepping segments of the WVF (segments I and III), which favors the formation of a negative flower structure (Figure 1B).
The VFS, along with the PFZ on the east, belongs to a system of faults and subduction zones that accommodate part of the deformation due to the northwestward drift of the Philippine Sea Plate (PSP) toward the Sunda Plate (Figure 1). The MVFS branches southward from the PFZ and appears to terminate near the rifting front of the Macolod Corridor, an extensional structure which defines a rift.
An interlayered cover of Holocene fluvial deposits and lacustrine sediments ranging in texture from clay to gravel (Mines and Geosciences Bureau, 2010) and as thick as 100 m adjacent to and under Laguna Lake comprises the unconfined aquifer in the zone of creeping faults (CEST, 2004). The Pleistocene Guadalupe Formation underlies the unconfined aquifer in the zone of creeping faults (Figure 2), Laguna Lake, and in many parts of Metro Manila, (Clemente et al., 2001). The Guadalupe Formation is composed of Early to Late Pleistocene conglomerate, silty mudstone, tuffaceous sandstone, and well-bedded tuff units that are most likely derived from a nearby volcanic center (Zanoria, 1988). A semi-permeable layer, which in some places is as thick as 45 m, separates the unconfined and the confined aquifer (Guadalupe Formation). Basement rocks that are exposed north and east of the Marikina Valley belong to the Angat ophiolite of possible Cretaceous age (Arcilla et al., 1989) and Upper Cretaceous pillow basalts that are genetically unrelated to the ophiolites. Younger units also include the other tertiary sedimentary units (Laguna Formation and the Taal Tuff) and igneous intrusions. Recharge to the groundwater system comes from rainfall and induced flow from Laguna Lake (Clemente et al., 2001).
Methodology
Periodic deformation surveys for determining displacements and short-term slip rates commenced in September 1999. Since slip is almost purely vertical, we employed precise leveling using an electronic digital level (TOPCON DL-103AF), a bar-code leveling staff, and several fixed benchmarks on stable and solid ground across selected fault creep scarps (Figure 3). All of the survey lines are located along the road. After the first detailed deformation survey was conducted in 1999, these sites were periodically resurveyed (initially every quarter, then twice a year later) to allow monitoring and estimation of the short-term slip rates. As of September 2018, there were 10 lines being surveyed periodically. As of this study, with the closure of the northernmost site (NPC) and the addition of another line at the southernmost site (JUA-C), there are eight lines (GRV-B and C, ADL, VOS-A and B, and JUA-A, B, and C; Figure 2) being surveyed periodically.
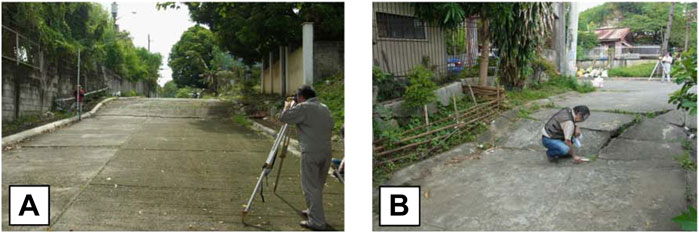
FIGURE 3. Precise measurement of displacement across the creeping trace of the West Marikina Valley Fault (WMVF) at the southernmost survey sites at (A) Villa Olympia Subdivision (VOS) and (B) Juana Subdivision (JUA-B). Measurement of displacement is done twice a year and uses a digital-level and dual-face telescopic leveling staff.
Precise leveling had been applied in the precise and accurate determination of displacements and slip rates of fault deformation worldwide (Sylvester, 1982; Murase et al., 2013; Pousse Beltran et al., 2016; Tiryakioğlu et al., 2019). The use of precise leveling to measure vertical displacements offers advantages over other techniques. Precise leveling is an accurate and precise method that is most appropriate for measuring near-field deformation. Deformation due to groundwater withdrawal affects Metro Manila and the surrounding regions but nowhere else outside of the study area (creeping segment of the VFS) is displacement concentrated along faults. Although the measurements are limited to specific points with no spatial continuity, a large number of survey lines distributed over the entire length of the creeping segment can offset this limitation. New techniques such as InSAR allow wider coverage of the spatial variations in deformation. In spite of its accuracy for far-field fault displacement, InSAR is unreliable in the near-field as it is vulnerable to large displacements and complex textures (e.g., vegetation) found within the near-field. In addition, InSAR will only cover the past <10 years since the Sentinel 1 and B images became freely available to the public only recently, and thus fails to capture the important trends reflected by our high-precision, near-field measurements. Our study avoids all the issues with coherence and the need for corrections (e.g., tropospheric and ionospheric), which may introduce higher uncertainty in creep rate estimates when compared with our field-based approach.
We employed time series analysis by temporal alignment of displacement and ground extraction plots to determine the timing correlation between significant changes in displacement and surges in groundwater extraction. The results may be merely suggestive of a cause and effect relationship but may be combined with other line(s) of evidence to come up with reasonable inferences. Though this approach does not require highly accurate displacements, the measurement results using the TOPCON DL-103AF are extremely accurate (the standard deviation is up to 2.5 mm for 1 km). The non-instrumental errors are difficult to quantify but the procedures employed ensured that these remain low. In contrast to the long (>1 km) survey lines employed by previous applications of the method, our survey lines across the fault creep scarps are short (Supplementary Figure S1). Maintaining short distances between benchmarks minimizes errors from refraction and collimation. The long interval between resurveys (= or > 2x/year) ensures that a large enough displacement has occurred. The error becomes proportionately less with larger displacement changes after many years of resurveys. Also, surveys are conducted rapidly to minimize scale errors due to staff expansion and eccentricity of staff inclination. Relative vertical heights of benchmarks were measured three times to minimize human and other non-instrumental errors. Displacement is estimated using a simple model that takes the difference in the average heights of benchmarks from each side of the fault. The displacement plot tracks changes in height relative to the first measurement period. Measurements across scarps in the early 1990s used a less precise hand level and graduated rod, but displacements were significantly larger, sight distances were shorter, and measurement intervals were longer.
Groundwater extraction for the cities and towns around the creeping zone was obtained from the National Water Resources Board (NWRB). The annual groundwater extraction within the 2, 3, 4, and 5 km buffer zones centered around the displacement survey sites are determined which are then compared with the displacements and slip rates from each survey site. This is akin to the GIS weights of evidence modeling procedure using Bayesian statistics. Due to their close proximity, a combined groundwater extraction plot (JUA-VOS) represents the two southernmost survey sites (JUA and VOS). The buffer zones are centered midway between these sites.
Other parameters (i.e., groundwater extraction regulations and proximity to the source of recharge) were also considered in the analysis of displacement and slip rate variations.
Results of 1999–2019 deformation surveys
The results of displacement measurements using a digital leveling instrument since September 1999 are summarized in Figure 4A. Displacement values for each of the survey lines are shown in Supplementary Table S1. Table 1 also shows the slip rates estimated from the displacements. Since 1999, only one survey line, VOS, showed continuous significant movement (Figure 4A). On the other hand, only NPC-A showed little or no displacement since the survey started in 1999 (Figure 4A). NPC-A showed no creep dislocation since the survey started in 1999 even though echelon cracks on the pavements are still visible (Figure 4A). The two other NPC survey lines (NPC-B and NBC-C), however, displayed continuous creep dislocation rates of 1.23 cm/yr until 2006 (Table 1). After 2006, NPC-B exhibited almost no displacement changes while NPC-C showed minute changes until the last survey in September 2018.
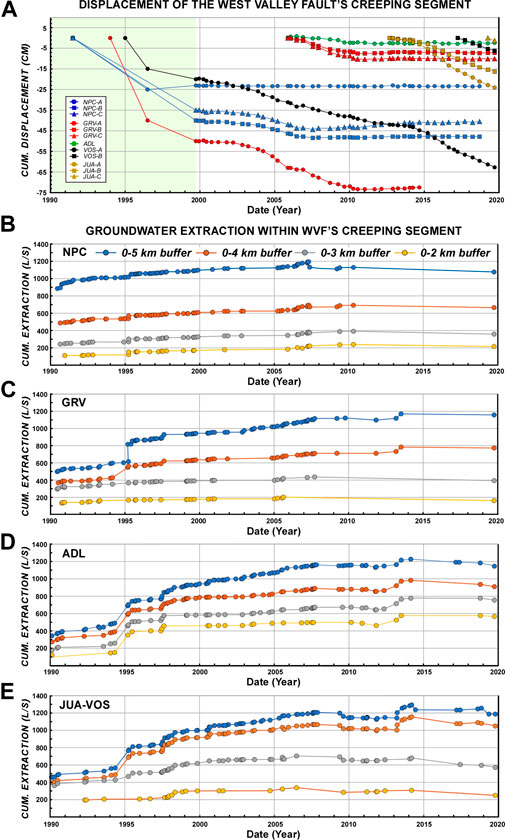
FIGURE 4. (A) Combined plot of differential displacement from the 1990s (left part of the plot) and from 1999 to 2019 (right part of the plot) for the 12 survey lines across creeping segments in five sites (NPC, GRV, ADL, VOS, and JUA; see Figures 1, 2 for locations). Value for each resurvey is the difference from the displacement measured in a preceding survey. The first resurvey conducted in December 1999 was preceded by the September 1999 survey. Values below zero indicate that the east side of the fault is moving down. Also shown are plots of cumulative groundwater extraction (in liters per second or lps) within the 2, 3, 4, and 5 km buffer zones centered around NPC (B), GRV (C), ADL (D), and JUA-VOS (E). (Note: multiple data points within each year included. Cumulative groundwater extraction values at any given time for each buffer zone are estimated by subtracting values from wells whose permits were canceled at the time indicated).
All three GRV survey lines (GRV-A, GRV-B, and GRV-C) had relatively significant displacements only until March 2010 (Figure 4A). Clear acceleration of creep is evident at GRV-A from 2004 to 2009 and, briefly, at GRV-B and GRV-C from 2007 to 2008 when the slip rate reached 4.55 cm/yr (Figure 4A; Table 1). After 2010, little or no change in displacement was measured in all GRV sites (Figure 4A). For a brief period, almost no displacement changes were measured in GRV-A, GRV-B, and GRV-C from late 2005 to late 2006 (Figure 4A). Like the GRV lines, ADL (first established in September 2006) had continuous creep dislocation until June 2010, after which little or no movement was measured (Figure 4A).
VOS and JUA, the two southernmost deformation survey sites, yielded temporal displacement patterns and rates that are different from those of the northern sites (NPC, GRV, and ADL) (Figure 4A). Between 1999 and the most recent survey conducted in September 2019, VOS-A exhibited continuous creep dislocation. A brief period of near flattening from 2012 to 2013 was recorded at VOS-A (Figure 4A). VOS-B, which was first surveyed in March 2017, also showed continuous creep dislocation (Figure 4A). A significant shift in the slip rate occurred at VOS-A since 2014, as creep accelerated to 3.5 cm/yr from an average rate of 1.60 cm/yr from 1999 to 2014 (Figure 4A; Table 1). VOS-B had comparable slip rates to those of VOS-A. VOS-B exhibited accelerated creep rates similar to VOS-A (Figure 4A). Until the most recent survey conducted in September 2019, JUA-A and JUA-B (since September 2012) and JUA-C (since March 2019) displayed continuous creep dislocation (Figure 4A). A significant shift at JUA-A and JUA-B also occurred starting in 2014, as the creep rate accelerated (3.5 cm/yr for JUA-B; 4.17 cm/yr for JUA-A) (Figure 4A; Table 1). The slip rate at JUA-C (3.5 cm/yr; established in March 2019) is consistent with the observed accelerated creep rates at JUA-A and JUA-B (Figure 4A; Table 1).
Figure 4A (shaded left portion of the plot) also shows the displacement measurements at NPC (A, B, and C), GRV-A, and VOS-A, while Table 1 summarizes the slip rates based on the pre-1999 estimates made by Rimando (2002) for comparison with the slip rates obtained from our precise measurements since 1999. Pre-1999 slip rate estimates are much higher. Based on the 1996 measurement of displacement across selected sites, the minimum rate of vertical slip ranged from 0.8–10 cm/yr and the maximum rate is 4.55–10 cm/yr on individual traces. Locally, some slip rates as high as 20 cm/yr were recorded. The average maximum vertical slip rate was 9.5 cm/yr while the mean minimum vertical slip rate was 7.25 cm/yr. Minimum slip rates ranged from 0.4–4.45 cm/yr while maximum slip rates ranged from 1.45–5.8 cm/yr. Localized high slip rates were on the order of 10 cm/yr.
Discussion
As creep is believed to be triggered by groundwater extraction, variations in displacement and slip rates are more likely linked to patterns of ground extraction. To verify this, groundwater extraction from wells around the survey sites for the 1977–2019 period is compared with the displacement and slip rate data. The cumulative amounts and rates of groundwater extraction from the wells around all survey sites (ADL, GRV, JUA-VOS, and NPC) from the late 70s until August 2019 are shown in detail in Figures 4B–E. The lines represent groundwater extraction within the 2 km, 3 km, 4 km, and 5 km buffer zones centered around each survey site (Figure 5). It includes plots of multiple data points within a year for each of the survey sites at NPC (Figure 4B), GRV (Figure 4D), ADL (Figure 4D), and JUA-VOS (Figure 4E). JUA and VOS were treated as one site due to their proximity. The midpoint between JUA and VOS was taken as the center of buffer zones for JUA-VOS. Supplementary Table S2 shows only the annual total groundwater extraction for each site’s buffer zones. The influences of groundwater extraction regulations and proximity to Laguna Lake (as a source of recharge) are also included in the discussion of displacement and strike-slip variations.
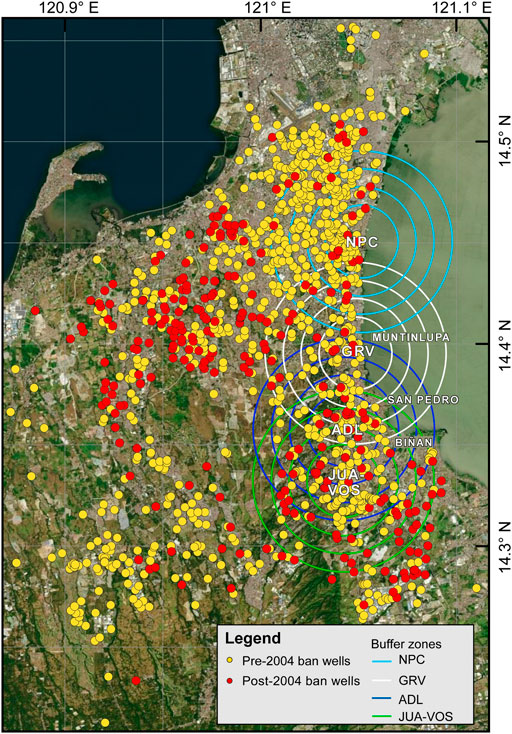
FIGURE 5. Location of wells in the vicinity of the survey sites. Around the survey sites are 2, 3, 4, and 5 km buffer zones drawn for purposes of determining the correlation of displacement and groundwater extraction from wells around the sites. The wells shown include all those with NWRB licenses granted from 1977 to 2019 (August). Solid yellow dots are wells with operation licenses granted before the ban on new wells (through NWRB Resolution No. 001–0904) on 22 September 2004. Solid red dots are wells with operation licenses granted after this date. Due to the close proximity of VOS and JUA, common buffer zones centered between the two sites were drawn. The satellite image of the survey sites is from Microsoft Bing Maps (https://www.bing.com/maps).
Accounting for the high slip rates in the 1990s
The poor temporal and spatial correlation between groundwater levels and slip rates in the 1990s (Rimando, 2002) does not accurately reflect the role of groundwater in triggering fault creep. In the first place, groundwater level maps were not generated frequently enough to reflect short-term changes in groundwater extraction. It can be shown that in the 1990s, when fault creep was first documented, displacements and slip rates are related to the rates of pumping groundwater. The 1996 slip estimates for NPC-A, GRV-A, and VOS-A are quite high compared with those of the late 1990s (Table 1; Punongbayan and Rimando, 1996; Rimando, 2002). It was about this time when numerous cases of damage by vertical displacement to roads, residential units, commercial and industrial buildings, and public and private structures were reported (Punongbayan and Rimando, 1996; Rimando, 2002). This period of high slip rate is preceded by the abrupt and significant increase (by more than 200 lps) in the amount of groundwater extraction recorded near ADL in 1995 (Figure 4D). The exact lag between the start of the surge in extraction and the significant change in displacement cannot be determined, as no periodic measurement or continuous displacement monitoring was being conducted. However, there is an interval of approximately 1 ½ years between the time of the abrupt increase in extraction (February 1995) and the time of the earliest displacement measurement following the surge. The abrupt increase in extraction is recorded within 2-, 3-, 4-, and 5-k distance around ADL but only within the outer zones around GRV (Figure 4C); Supplementary Table S2) and JUA-VOS (Figure 4E; Supplementary Table S2), which indicates that the cause of the 1995 extraction spike is within the innermost (i.e., 2 km) zone around ADL. The abrupt change near ADL, which influenced slip in other sites, is attributed to the emergence of new large-capacity pumping activity close to ADL, mainly by industrial and local government unit (LGU) operators. The 1995 surge was followed by a more minor increase in 1997–1998 around ADL (Figure 4D) and JUA-VOS (Figure 4E).
Groundwater extraction regulations and slip rate changes
Since 1999 (until 2019), there had been no significant increases in creep rates in the northern part of the creeping zone while the southernmost deformation survey sites show slip rates that are comparable to those in the late 1990s (Table 1). Since 1999, there had been little or no change in the slip rate at NPC-A. A flatlining in the displacement curve for NPC (B and C) since 2007 and for GRV (A, B, and C) (Figure 4) since 2010 is also evident. Likewise, a flatlining is displayed in 2006 to late 2007 at GRV-A, -B, and -C. During these periods, groundwater extraction did not vary significantly around all the sites. The lack of significant changes in extraction around NPC and GRV had been going on since the mid-1990s (Figures 4B,C; Supplementary Table S2). In contrast, both the areas within 4 km around JUA-VOS (and ADL) in the southern part of the creeping zone increased by as much as 280 lps from 1995 to 2010 (Figure 4E; Supplementary Table S2). Also, there was little or no increase in groundwater extraction at NPC and GRV after 2010. The flatlining of slip rates at the northern sites can be related to government regulation of groundwater extraction. In 2004, the National Water Resources Board issued NWRB Resolution No. 001–0904 (National Water Resources Board, 2004) to check the overextraction of groundwater in many parts of Metro Manila which includes Muntinlupa City and the cities near the northern sites (Taguig, Las Piñas, and Parañaque; refer to inset map in Figure 2 for location). Cities and towns near and around ADL, VOS, and JUA (e.g., San Pedro, Biñan, and Sta. Rosa; refer to inset map in Figure 2 for location) do not belong to Metro Manila. It was later amended a few times (e.g., NWRB Resolution No. 004–0507 dated 16 May 2007, NWRB Resolution No. 03–0607 dated 22 October 2008, and NWRB Resolution No. 020–1209 dated 10 December 2009) to reiterate the restrictions. These resolutions resulted in the suspension of granting new permits, nonrenewal of permits, and the cancellation of permits in these areas (Supplementary Table S3). It was reported that NWRB shut down a total of 1,008 illegal or abandoned deep wells from 2008 to 2013 in and outside Metro Manila (Department of Environment and Natural Resources, 2015).
While there was little or no change in extraction after 2010 near NPC (Figure 4B) and GRV (Figure 4C), extraction around ADL (Figure 4D) and JUA-VOS (Figure 4E) had been increasing substantially since 1995. Displacement at VOS continued increasing at a relatively steady rate from 1999 until 2014 when displacements increased at faster rates along with those at JUA-A and JUA-B (Figure 4A). VOS-B and JUA-C reflected these trends since monitoring started in 2017 and 2012, respectively. These increases were preceded by an ∼120 lps jump in extraction in 2013 within the outer JUA-VOS buffer zones (Figure 4E; Supplementary Table S2). This jump in extraction was also recorded within the outermost GRV buffer zones (Figure 4C) and within all ADL buffer zones, which means that the cause of the surge in the pumping rate occurred within the innermost buffer zone of ADL. This time, only one big industrial establishment was responsible for the surge. The start of the surge in extraction around ADL, JUA-VOS, and GRV (March 2013) preceded, by 1 year, the start of a significant increase in displacements at JUA and VOS sites. Though the major cause of extraction surges in 2013 is much closer to ADL than JUA-VOS, ADL showed almost no changes in displacement and slip rate after 2013 (Figure 4A). This is probably due to the larger number of new wells around JUA-VOS (Figure 5) on top of the effect of the 2013 extraction surge.
Creep appears to have regained the level of the slip rate that VOS-A had in the late 1990s, while creep had long stopped (for at least 4 years) in the northern deformation survey sites (NPC, GRV, and ADL) (Figure 4A; Table 1). As discussed in the following section, the slip rate pattern at ADL is more similar to that of GRV and NPC despite being very close to wells having high rates of extraction. The renewed acceleration of slip at VOS and JUA (Figure 4A) in 2014 is consistent with a southward shift in groundwater extraction. As shown in Figure 5; Table 2, and Supplementary Table S3, post-2004 well numbers and capacities in the vicinity of the southern sites (San Pedro and Binan) are far greater than those in the vicinity of NPC and ADL (Muntinlupa, Paranaque, Las Pinas, and Taguig). These strongly suggest that the 2004 NWRB ban and its succeeding amended versions influenced the southward shift and affected the groundwater extraction balance in favor of the southern portion of the creeping zone.
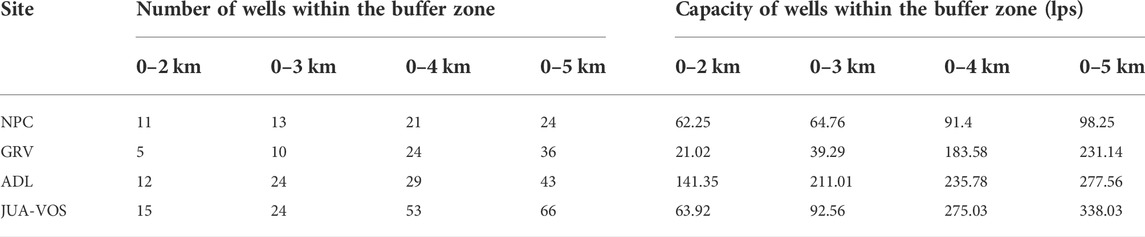
TABLE 2. Post-2004 number and capacity of wells for each buffer zone (cumulative) as of August 2019.
Influence of proximity to the source of recharge
Distance from a key source of recharge (Laguna de Bay) partly explains the variability of the slip rate. Though Laguna Lake contributes only a small portion (11%) of the recharge to the Metro Manila aquifer system (Clemente et al., 2001), a large portion of the rainfall eventually finds its way to the lake. Since Laguna Lake is right beside the VFS creeping zone (Figures 1, 2), it probably constitutes a sizeable portion of the recharge mix in the WVF creeping zone. The flatlining of the slip rate at NPC-B and C (2006) started earlier than at GRV and ADL sites (2010), which may be partly due to the location of NPC right beside Laguna de Bay (Figures 1, 2). Its relative proximity to the lake allows the reservoir underneath NPC to recover/recharge faster. Distance of the sites from the lake becomes progressively greater from NPC in the north to the southernmost survey sites (JUA and VOS) (Figures 1,2). More likely, both the decline in groundwater extraction and the relative ease of recharge account for the earlier flatlining in the slip at NPC (Figure 4A). The subtle decrease in displacement at NPC-C (until its termination in September 2018), which is the closest NPC survey line to the Laguna de Bay shoreline, could indicate some degree of aquifer recovery due to recharge from Laguna de Bay. This could also explain why displacement at ADL remained virtually unchanged since 2010 despite having a similar groundwater extraction pattern around it to that around JUA-VOS. ADL is closer (∼2.3 km away) to Laguna Lake than JUA-VOS is (∼4 km away) to the nearest lake shoreline. This makes JUA-VOS less accessible to recharge from Laguna de Bay, which is more conducive to continued and accelerated creep.
The influence of rainfall, floods, and seasonal changes is too small to be resolved by periodic digital-level surveys. These are later discussed using the results of more near-field continuous monitoring (in preparation).
Prospects of seismic slip
Sustained accelerated fault creep in the southern part of the creeping segment raises an issue about the possible occurrence of seismicity. The WVF’s creeping zone corresponds to the dilational gap between the right-stepping segments I and III (Figure 1B; Rimando, 2002; Rimando and Knuepfer, 2006). Some of the creeping segments had been shown to occur along active pre-existing tectonic traces based on pre-existing scarps (Rimando, 2002) and on creek (Rimando, 2002) and trench (Kinugasa, Personal communication) exposures of fault strands cutting sediments beneath anthropogenic layers. Whether excessive groundwater extraction can lead to seismicity is quite difficult to ascertain and there is no known reliable method for this. As implied by the Mohr–Coulomb failure criterion, fault failure results when the frictional strength and normal (or clamping) stresses are exceeded by shear stress on the fault. Whether fault failure will result directly in aseismic slip or seismicity depends on the frictional properties of faults (Guglielmi et al., 2015). Groundwater extraction causes a reduction in the pore fluid pressure and an increase in effective normal stress (Segall, 1985; Segall, 1992), which inhibits failure, but the compaction of the aquifer and the reduction of the vertical load of water decrease the normal stress, promoting failure (Holzer et al., 1979; Segall, 1989). Groundwater extraction can cause the shear stress along the fault to evolve non-uniformly to locally induce fault rupture, which may propagate beyond the areas of the overextraction and affect tectonically controlled segments. Minor tectonic stress changes and other changes brought about by natural processes are known to deliver the final stress increment that can advance the timing and alter the location of seismicity (Foulger et al., 2018). Likewise, stress perturbations involving seismicity induced by groundwater withdrawal are minuscule compared with stress drops during large earthquakes but are enough to modulate seismicity (Kundu et al., 2015, 2019; Foulger et al., 2018; Tiwari et al., 2021). Several cases of seismicity had been attributed to groundwater extraction or artificial dewatering (González et al., 2012; Amos et al., 2014; Kundu et al., 2015, 2019; Foulger et al., 2018; Wu, 2021).
Pennington et al.’s (1986) model of induced seismicity postulated the possibility of seismicity occurring along a creeping fault. According to the model, continued depressurization strengthens the fault through the development of “barriers” (high-strength, low-stress portions of the fault which are resistant to slip) and “asperities” (highly-stressed barriers), which resist slip. Once the resistance is overcome by the effective stress, sudden failure and earthquake occur. Continued extraction could lead to the development of bigger barriers and asperities, increasing the size of succeeding earthquakes (Pennington et al., 1986). The development of seismicity due to continued depressurization from aseismic slip occurring above the locking depth is contingent on the stress conditions and on the development of barriers and asperities. Equally unknown is the amount of time that is necessary for failure and for each cycle to be completed.
Fault jogs and bends are surface expressions of larger-scale barriers and asperities (Scholz and Engelder, 1976; Aki, 1979; Lay et al., 1982). Both anti-dilational and dilational jogs are known sites of rupture initiation (Bakun et al., 1980; King and Nabelek, 1985; Sibson, 1985; King, 1986). These also form obstacles to short- and long-term slip transfer along faults, but dilational jogs are the preferred sites for rupture arrest (Sibson 1985, 1986) owing to the difficulty of opening such features in the fluid-saturated crust (Sibson 1985). This is shown by a series of recent ruptures along the San Andreas Fault system and other strike-slip faults around the world (Barka et al., 1987; Knuepfer et al., 1987; Sibson, 1987). The WVF creeping zone constitutes a larger-scale barrier which could weaken and fail through continued depressurization. Its failure could induce static stress changes that might cause increased stress in adjoining areas. This could advance the failure of either of the adjacent segments (segments I and III). The 45 km segment I of the WVF has an estimated return period of 400–600 years and has not moved since 1599 (Nelson et al., 2000; Rimando, 2002; Rimando and Knuepfer, 2006). Weakening of the creeping zone could also hasten stress load transfer and facilitate slip transfer during a seismic event involving either of the adjacent segments. The occurrence and timing of any contagion depend on the duration for a barrier to yield and transfer an additional stress load from one segment to another and on the physical state of the target segment of the transfer (Perkins, 1985), which are unknown.
Continued overextraction of groundwater and fault creep does not always directly trigger an earthquake (e.g., Lui et al., 2021) but continued excessive groundwater extraction will exacerbate the damage due to fault creep.
Summary and conclusion
The unusually large amounts of displacements and high rates of slip along the creeping segment of the WVF in the mid-1990s is related to the surge in extraction (by more than 200 lps) which can be attributed to industrial and local water utilities near ADL. Since 1999, slip rates at NPC, GRV, and ADL also respond to changes in the amounts of groundwater extraction, which in turn are influenced by government regulatory interventions. Flatlining in slip rates in these sites occurred after a series of NWRB bans on new groundwater extraction permits and nonrenewal or cancellation of licenses in these areas in 2004 and in the succeeding years of renewed campaigns against groundwater extraction. The increase in the number and capacity of wells in the southern part of the creeping zones, on the other hand, resulted in the continuation/resurgence of accelerated creep at VOS and JUA since 2014. This was preceded by an ∼120 lps increase in extraction within 5 km of JUA and VOS and was caused by excessive pumping near ADL.
The greater distance of VOS and JUA from Laguna de Bay, which makes recharge from the lake more difficult, also partly explains the continued accelerated creep in the south. While groundwater extraction around ADL and JUA-VOS is not significantly different, ADL is much closer to Laguna de Bay.
Fault creep due to excessive groundwater extraction alone constitutes a serious hazard to man-made structures within the creeping zone. Implementation and amendments to groundwater extraction regulations should take note of the accelerated creep in the south. Groundwater extraction should be closely monitored and controlled in the northern portion of the creeping zone to avoid the resurgence of overextraction and accelerated fault creep.
Creep occurs along some known pre-existing active tectonic segments of the WVF. Excessive groundwater extraction has the potential of disrupting the natural course of deformation (within the creeping zone and along the WVF’s active segments I and III) resulting from the northwestward motion of the Philippine Sea Plate and the influence of the motion of the major bounding structures in the region. The effects of the fault creep and, potentially, the modulated occurrence of seismic activity can be avoided by tighter regulation of groundwater extraction, especially in the southern part of the creeping zone where access to recharge is more difficult. Precise displacement surveys should also continue to monitor both slip rate changes within and around the creeping zone and compliance with groundwater extraction regulations.
For a more comprehensive monitoring and characterization of deformation within the creeping zone and surrounding areas, the combined use of remote sensing technology such as InSAR and a denser and wider network of precise leveling lines is recommended for future work. InSAR provides both breadth and measurement accuracy while leveling provides the detail and precision needed for near-field deformation.
Data availability statement
The original contributions presented in the study are included in the article/Supplementary Material; further inquiries can be directed to the corresponding author.
Author contributions
RR: writing of the original draft, review and editing, data acquisition, data analysis, and supervision (Philippine side); KK: review, data acquisition, data analysis, and development of the mathematical model used in estimating displacements; and YK: conceptualization of the research, review, data gathering, and overall supervision of the whole research investigation.
Funding
This research was supported by research funds of the Philippine Institute of Volcanology and Seismology-Department of Science and Technology in accordance with the General Appropriations Act of the Republic of the Philippines, Tokyo Institute of Technology, and Tokyo Metropolitan College of Industrial Technology.
Acknowledgments
The authors would like to thank the management of the National Power Corporation and the homeowner’s associations of the Gruenville, Adelina I, Villa Olympia Phase 6, and Juana Complex II-A and B subdivisions. Mr. Renato Garduque provided invaluable assistance in the conduct of the deformation surveys. The authors would also like to acknowledge the National Water Resources Board (NWRB) of the Philippines for access to data on the wells near the survey sites in Metro Manila and in southern Luzon.
Conflict of interest
The authors declare that the research was conducted in the absence of any commercial or financial relationships that could be construed as a potential conflict of interest.
Publisher’s note
All claims expressed in this article are solely those of the authors and do not necessarily represent those of their affiliated organizations, or those of the publisher, the editors, and the reviewers. Any product that may be evaluated in this article, or claim that may be made by its manufacturer, is not guaranteed or endorsed by the publisher.
Supplementary material
The Supplementary Material for this article can be found online at: https://www.frontiersin.org/articles/10.3389/feart.2022.935161/full#supplementary-material
References
Aki, K. (1979). Characterization of barriers on an earthquake fault. J. Geophys. Res. 84 (11), 6140–6148. doi:10.1029/JB084iB11p06140
Ambraseys, N. N. (1970). Some characteristic features of the Anatolian fault zone. Tectonophysics 9 (2-3), 143–165. doi:10.1016/0040-1951(70)90014-4
Amos, C. B., Audet, P., Hammond, W. C., Bürgmann, R., Johanson, I. A., and Blewitt, G. (2014). Uplift and seismicity driven by groundwater depletion in central California. Nature 509, 483–486. doi:10.1038/nature13275
Arcilla, C. A., Ruelo, H. B., and Umbal, J. (1989). The Angat ophiolite, Luzon, Philippines: Lithology, structure, and problems in age interpretation. Tectonophysics 168 (1-3), 127–135. doi:10.1016/0040-1951(89)90372-7
Bakun, W. H., Stewart, R. M., Bufe, C. G., and Marks, S. M. (1980). Implication of seismicity for failure of a section of the San Andreas fault. Bull. Seismol. Soc. Am. 70 (1), 185–201. doi:10.1785/bssa0700010185
Barka, A. A., Kadinsky-Cade, K., and Toksoz, N. N. (1987). North Anatolian fault geometry and earthquake activity. Seismol. Res. Lett. 58, 31. doi:10.1785/BSSA0700010185
Bureau of Mines and Geosciences, (1981). Geology and mineral Resources of the Philippines, Part I. Manila: Bureau of Mines and Geosciences.
Burford, R. O., and Harsh, P. W. (1980). Slip on the San Andreas fault in central California from alinement array surveys. Bull. Seismol. Soc. Am. 70 (4), 1233–1261. doi:10.1785/BSSA0700041233
Cest, Inc. (2004). Water Resources assessment for prioritized critical areas (Phase 1): Final report. (Metro Manila).
Clemente, R. S., Abracosa, R. P., David, C. C., Inocencio, A. B., and Tabios, G. Q. (2001). Metro Manila and Metro cebu groundwater assessment: Discussion paper series No. 2001-05. Makati: Philippine Institute for Development Studies.
Department of Environment and Natural Resources, (2015). Government to public: Report illegal deep wells. Available at: https://www.officialgazette.gov.ph/2015/09/24/denr-report-illegal-deep-wells/(Accessed April 16, 2022).
Foulger, G. R., Wilson, M. P., Gluyas, J. G., Julian, B. R., and Davies, R. J. (2018). Global review of human-induced earthquakes. Earth. Sci. Rev. 178, 438–514. doi:10.1016/j.earscirev.2017.07.008
Gonzalez, P. J., Tiampo, K. F., Palano, M., Cannavo, F., and Frenandez, J. (2012). The 2011 Lorca earthquake slip distribution controlled by groundwater crustal unloading. Nat. Geosci. 5 (11), 821–825. doi:10.1038/ngeo1610
Guglielmi, Y., Cappa, F., Avouac, J. P., Henry, P., and Elsworth, D. (2015). Seismicity triggered by fluid injection-induced aseismic slip. Science 348, 1224–1226. doi:10.1126/science.aab0476
Holzer, T. L., Davis, S. N., and Lofgren, B. E. (1979). Faulting caused by groundwater extraction in southcentral Arizona. J. Geophys. Res. 84 (B2), 603–612. doi:10.1029/jb084ib02p00603
Kaizuka, S., Matsuda, T., Oike, K., Togo, M., Yamashina, K., Takeuchi, A., et al. (1992). Active faults in the Weihe Basin and ground fissures in xian city, shaanxi province, China [in Japanese]. Bull. Earthq. Res. Inst. Suppl. 7, 1–186.
Kelson, K. I., Simpson, G. D., Lettis, W. R., and Haraden, C. C. (1996). Holocene slip rate and earthquake recurrence of the northern Calaveras fault at Leyden Creek, northern California. J. Geophys. Res. 101 (3), 5961–5975. doi:10.1029/95JB02244
King, G. C. P. (1986). Speculations on the geometry of the initiation and termination processes of earthquake rupture and its relation to morphology and geological structure. Pure Appl. Geophys. 124 (3), 567–585. doi:10.1007/BF00877216
King, G., and Nábělek, J. (1985). Role of fault bends in the initiation and termination of earthquake rupture. Science 228 (4702), 984–987. doi:10.1126/science.228.4702.984
Knuepfer, P. L. K., Bamberger, M. J., Turko, J. M., and Coppersmith, K. J. (1987). Characteristics of the boundaries of historical surface fault ruptures. Seismol. Res. Lett. 58, 31.
Kreitler, C. W. (1977)., 15. Houston, Texas, 203–214. doi:10.1111/j.1745-6584.1977.tb03165.xFault control of subsidence
Kundu, B., Vissa, N. K., Gahalaut, K., Gahalaut, V. K., Panda, D., and Malik, K. (2019). Influence of anthropogenic groundwater pumping on the 2017 November 12 M7.3 Iran-Iraq border earthquake. Geophys. J. Int. 218, 833–839. doi:10.1093/gji/ggz195
Kundu, B., Vissa, N. K., and Gahalaut, V. K. (2015). Influence of anthropogenic groundwater unloading in Indo-Gangetic plains on the 25 April 2015 Mw 7.8 Gorkha, Nepal earthquake. Geophys. Res. Lett. 42, 10607–10613. doi:10.1002/2015GL066616
Lay, T., Kanamori, H., and Ruff, L. (1982). The asperity model and the nature of large subduction zone earthquakes. Earthq. Predict. Res. 1, 3–71.
Lienkaemper, J. J., Borchardt, G., and Lisowski, M. (1991). Historic creep rate and potential for seismic slip along the Hayward fault, California. J. Geophys. Res. 96 (B11), 18261–18283. doi:10.1029/91JB01589
Lui, S. K. Y., Huang, Y., and Young, R. P. (2021). The role of fluid pressure-induced aseismic slip in earthquake cycle modulation. J. Geophys. Res. Solid Earth 126, e2020JB021196. doi:10.1029/2020JB021196
Matsuda, T., Kaizuka, S., Okada, A., and Imaizumi, T. (1986). Creeping ground fissures in the city (of) Xian, Shanxi, China–a review with the brief observation [in Japanese]. J. Act. Fault Stud. 2, 3–10. doi:10.11462/afr1985.1986.2_3
Mines , , and Geosciences Bureau (MGB), (2010). Geology of the Philippines. 2nd ed. (Quezon City: Mines and Geosciences Bureau, Department of Environment and Natural Resources).
Murase, M., Matta, N., Lin, C. H., Chen, W. S., and Koizumi, N. (2013). An episodic creep-slip event detected by precise levelling surveys in the central part of the Longitudinal Valley Fault, eastern Taiwan, in 2011–2012. Tectonophysics 608, 904–913. doi:10.1016/j.tecto.2013.07.027
National Water Resources Board (2008). Amended resolution 03-0607: Amendment to “policy guidelines on the processing and issuance of water permits” and “conditional water permits. Available at: https://nwrb.gov.ph/images/Board_Resolution/Res_03-0607_as_Amended.pdf (Accessed April 16, 2022).
National Water Resources Board (2004). Resolution No. 001-0904: Policy recommendations for Metro Manila critical areas. Available at: https://nwrb.gov.ph/images/Board_Resolution/BR_001-0904.pdf (Accessed November 19, 2021).
National Water Resources Board (2007). Resolution No. 004-0507: Policy guidelines on the abstraction of groundwater for refilling stations. Available at: https://nwrb.gov.ph/images/Board_Resolution/Res_05-0507.pdf (Accessed November 19, 2021).
National Water Resources Board (2009). Resolution No. 020-1209: Non-Acceptance of water permit applications (WPAS) and suspension of processing of pending WPAs in critical areas in Metro Manila and Metro cebu that are under mining conditions. Available at: https://nwrb.gov.ph/images/Board_Resolution/Res_20-1209.pdf (Accessed April 16, 2022).
Nelson, A. R., Personius, S. F., Rimando, R. E., Punongbayan, R. S., Tungol, N., Mirabueno, H., et al. (2000). Multiple large earthquakes in the past 1500 years on a fault in metropolitan Manila, the Philippines. Bull. Seismol. Soc. Am. 90 (1), 73–85. doi:10.1785/0119990002
Pennington, W. D., Davis, S. D., Carlson, S. M., Dupree, J., and Ewing, T. E. (1986). The evolution of seismic barriers and asperities caused by the depressuring of fault planes in oil and gas fields of South Texas. Bull. Seismol. Soc. Am. 76 (4), 939–948.
Perkins, D. M. (1985). “Contagious fault rupture, probabilistic hazard, and contagion observability,” in Directions in paleoseismology: Proceedings of conference XXIX (Albuquerque, New Mexico: United States Geological Survey), 428–439. Available at: https://pubs.usgs.gov/of/1987/0673/report.pdf.
Pousse Beltran, L., Pathier, E., Jouanne, F., Vassallo, R., Reinoza, C., Audemard, F., et al. (2016). Spatial and temporal variations in creep rate along the El Pilar fault at the Caribbean-South American plate boundary (Venezuela), from InSAR. J. Geophys. Res. Solid Earth 121, 8276–8296. doi:10.1002/2016JB013121
Punongbayan, R. S., and Rimando, R. E. (1996). Detailed mapping of active faults in the Fort Bonifacio area and vicinity. Metro Manila, Philippines: Department of Science and Technology.
Rimando, R. E., and Knuepfer, P. L. (2006). Neotectonics of the Marikina Valley fault system (MVFS) and tectonic framework of structures in northern and central Luzon, Philippines. Tectonophysics 415 (1-4), 17–38. doi:10.1016/j.tecto.2005.11.009
Rimando, R. E. (2002). Neotectonic and paleoseismic study of the Marikina Valley fault system, Philippines. [Binghamton (NY)]: State University of New York at Binghamton. [Dissertation].
Rimando, R. E., Panol, M. D., Daligdig, J. A., Lanuza, L., Besana, G. M., Takezono, M., et al. (2000). Aseismic slip of the Marikina fault in Metro Manila, Philippines (abstract). EOS 81, WP147.
Savage, J. C., Prescott, W. H., Lisowski, M., and King, N. (1979). Geodolite measurements of deformation near Hollister, California, 1971–1978. J. Geophys. Res. 84 (13), 7599–7615. doi:10.1029/JB084iB13p07599
Scholz, C., and Engelder, J. T. (1976). The role of asperity indentation and ploughing in rock friction — I. Int. J. Rock Mech. Min. Sci. Geomechanics Abstr. 13, 149–154. doi:10.1016/0148-9062(76)90819-6
Segall, P. (1989). Earthquakes triggered by fluid extraction. Geology, 17, 942–946. doi:10.1130/0091-7613(1989)017%3C0942
Segall, P. (1992). Induced stresses due to fluid extraction from axisymmetric reservoirs. Pure Appl. Geophys. 139, 535–560. doi:10.1007/bf00879950
Segall, P. (1985). Stress and subsidence resulting from subsurface fluid withdrawal in the epicentral region of the 1983 Coalinga earthquake. J. Geophys. Res. 90, 6801–6816. doi:10.1029/jb090ib08p06801
Sibson, R. H. (1986). “Rupture interaction with fault jogs,” in Earthquake source mechanics. Maurice ewing ser. 6. Editors S. Das, J. Boatwright, and C. H. Scholz (American Geophysical Union Mon), 37, 157–168. doi:10.1029/GM037p0157
Sibson, R. N. (1987). Earthquake rupturing as a hydrothermal mineralizing agent. Geology, 15, 701–704. doi:10.1130/0091-7613(1987)15%3C701
Sibson, R. N. (1985). “Effects of fault heterogeneity on rupture propagation,” in Directions in paleoseismology: Proceedings of conference XXIX (Albuquerque, New Mexico: United States Geological Survey), 362–373. Available at: https://pubs.usgs.gov/of/1987/0673/report.pdf.
Stenner, H. D., and Ueta, K. (2000). “Looking for evidence of large surface rupturing events on the rapidly creeping Southern Calaveras fault, California,” in Proceeding of the hokudan international symposium and school on active Faulting (Hokudan, Japan: International Lithosphere Program Task Group II-5, Hokudan Town and Hokudan Board of Education), 479–486.
Sylvester, A. G. (1982)., Vol. 5. Munich, Germany: International Association of Geodesy, 162–174. No 258: Network Analysis Models).Precise leveling across active faults in CaliforniaProc. Int. Symposium Geodetic Netw. Comput.
Tiryakioğlu, I., Yigit, C. O., Ozkaymak, C., Baybura, T., Yilmaz, M., Ugur, M. A., et al. (2019). Active surface deformations detected by precise levelling surveys in the Afyon-Akşehir Graben, Western Anatolia, Turkey. Geofizika 36 (1), 33–52. doi:10.15233/gfz.2019.36.4
Tiwari, D. K., Jha, B., Kundu, B., Gahalaut, V. K., and Vissa, N. K. (2021). Groundwater extraction-induced seismicity around Delhi region, India. Sci. Rep. 11, 10097. doi:10.1038/s41598-021-89527-3
Wilson, M. P., Foulger, G. R., Gluyas, J. G., Davies, R. J., and Julian, B. R. (2017). HiQuake: The human‐induced earthquake database. Seismol. Res. Lett. 88 (6), 1560–1565. doi:10.1785/0220170112
Wu, W. (2021). A review of unloading-induced fault instability. Undergr. Space 6, 528–538. doi:10.1016/j.undsp.2020.11.001
Yu, S. B., and Liu, C. C. (1989). Fault creep on the central segment of the Longitudinal Valley fault, eastern Taiwan. Proc. Geol. Soc. China 32, 209–231.
Keywords: aseismic slip, West Marikina Valley Fault, dilational jog, groundwater withdrawal, modulated seismicity.
Citation: Rimando RE, Kurita K and Kinugasa Y (2022) Spatial and temporal variation of aseismic creep along the dilational jog of the West Valley Fault, Philippines: Hazard implications. Front. Earth Sci. 10:935161. doi: 10.3389/feart.2022.935161
Received: 03 May 2022; Accepted: 04 October 2022;
Published: 25 October 2022.
Edited by:
Brijesh K. Bansal, Indian Institute of Technology Delhi, IndiaReviewed by:
João Fontiela, University of Evora, PortugalBhaskar Kundu, National Institute of Technology Rourkela, India
Pallabee Choudhury, Institute of Seismological Research, India
Copyright © 2022 Rimando, Kurita and Kinugasa. This is an open-access article distributed under the terms of the Creative Commons Attribution License (CC BY). The use, distribution or reproduction in other forums is permitted, provided the original author(s) and the copyright owner(s) are credited and that the original publication in this journal is cited, in accordance with accepted academic practice. No use, distribution or reproduction is permitted which does not comply with these terms.
*Correspondence: Rolly E. Rimando, cm9sbHkucmltYW5kb0BwaGl2b2xjcy5kb3N0Lmdvdi5waA==