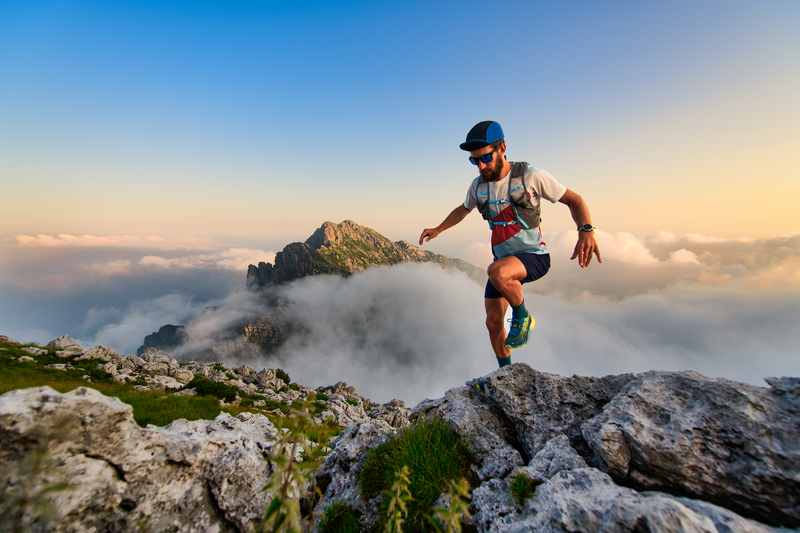
95% of researchers rate our articles as excellent or good
Learn more about the work of our research integrity team to safeguard the quality of each article we publish.
Find out more
ORIGINAL RESEARCH article
Front. Earth Sci. , 02 September 2022
Sec. Volcanology
Volume 10 - 2022 | https://doi.org/10.3389/feart.2022.911129
This article is part of the Research Topic From Magma Generation to Surface Processes in Monogenetic Volcanism View all 8 articles
The Clear Lake Volcanic Field (CLVF) is the northernmost and youngest field in a chain of volcanic fields in the California Coast Range mountains. Effusive and explosive volcanic activity in the field has spanned at least 2.1 million years, with the youngest eruptions comprising a series of maar craters at the edges of, and within, Clear Lake itself. This work documents the first direct ages for many of these maar deposits and builds the stratigraphic basis for interpreting eruptive processes and dynamics of the young eruptions which produced them. Detailed stratigraphy has distinguished maar eruption products from pyroclastic deposits (monolithologic falls and flows, previously mapped together with maars as a single unit) and established a set of six eruption facies from maar deposit lithology, grain size parameters, and depositional structures. Radiocarbon dates from carbon films found on clasts at three outcrops have constrained several of these maar eruptions to ∼8,500–13,500 years BP, coinciding with eruptive periods previously estimated based on lake core tephrachronology. Part of this period also coincides with indigenous inhabitation (<12,000 years BP), which suggests that oral histories of Pomo and other local tribes may contain descriptions of volcanic phenomena experienced by local residents of the CLVF. Collaboration between volcanologists and indigenous historians may add a valuable human dimension to the youngest eruptions of the Clear Lake Volcanic Field, and help inform future volcanic hazard assessment.
The Clear Lake Volcanic Field (CLVF) is the northernmost and youngest field in a chain of volcanic fields in the California Coast Range mountains (Figure 1A) (Hearn et al., 1981; Donnelly-Nolan et al., 1993; Stanley and Blakely, 1995; Hammersley and DePaolo, 2006). These fields were erupted as passage of the Mendocino triple junction and lengthening of the San Andreas Fault system opened magma pathways to the surface; as the nexus of subduction and transform movement marched northward, the age of each volcanic center approximately tracked the position of the triple junction and associated slab window at the time (Figure 2A; Hearn et al., 1981; Liu and Furlong, 1992; Stanley and Rodriguez, 1995). Located within the CLVF, Clear Lake itself is the oldest natural lake in North America and has existed for at least 2.5 million years (Sims, 1976, 1988; Sarna-Wojcicki et al., 1988). The lake and much of the volcanic field itself are located in a transtensional basin, which is responsible for both local and regional fault systems that have provided magma pathways to the surface (Hearn et al., 1981; Sims et al., 1988; Stanley and Rodriguez, 1995).
FIGURE 1. (A) Schematic map of northern California, showing the Coast Range volcanic centers, major regional faults, and approximate position of the Mendocino triple junction over the past 12 million years. CLVF = Clear Lake Volcanic Field, SVF = Sonoma Volcanic Field, BHVF = Berkeley Hills Volcanic Field, QSVF = Quien Sabe Volcanic Field. Modified from McLaughlin and Ohlin (1984); Stanley and Rodriguez (1995). (B) Summary geologic map of the Clear Lake Volcanic Field and surrounding areas, including areal extent of the Clear Lake Volcanics (green), Sonoma Volcanics (blue), Great Valley Sequence sedimentary rocks (peach), ophiolite (brown), and Franciscan Complex (purple). Modified from Rytuba et al. (2009). Terrain from National Map 3D Elevation Program (3DEP), USGS Earth Resources Observation and Science (EROS) Center, GMTED 2010 March 2021.
FIGURE 2. “Young pyroclastic” deposit and outcrop map of the CLVF, focused on the SE end of the Lake. Outcrops sampled for stratigraphy, grain-size, and radiocarbon analyses are noted with colored numbers and black circles with leaders; Soda Bay Group outcrops are shown in red, Riviera West Group in orange, Konocti Bay Group in green, and Lower Lake Group in blue. Known maar craters as mapped by Manson (1989) and Hearn et al. (1995) are marked with black circles, faults are depicted with black lines, and landslide scarps are shown with yellow lines. yp deposits of Hearn et al. (1995) are in purple. The 3.5–8 ka inferred paleoshoreline of Sims et al. (1988) is marked by a white dotted line. Faults are from the USGS Quaternary Fault and Fold Database (U.S. Geological Survey and California Geological Survey, 2022).
Volcanic rocks of the CLVF were erupted around (and through) Clear Lake over a 2.1 Ma period (Figure 2B; Donnelly-Nolan et al., 1993; Hearn et al., 1995; Stimac et al., 2001) over a basement comprised of Coast Range ophiolite, Mesozoic Great Valley Sequence, and Franciscan Complex (Hearn et al., 1981; Donnelly-Nolan et al., 1993). The rocks can be divided into four rough temporal groups: 1.3–2.1 Ma (basalt and basaltic andesite lavas, dacite and rhyolite lava flows and tuffs), 0.8–1.1 Ma (dacite and rhyolite flows and tuffs), 0.30–0.65 Ma (dacite and rhyolite lava flows), and 0.01–0.1 Ma (basaltic andesite scoria cones and maars) (Donnelly-Nolan et al., 1981). This volcanism was fed by a silicic magma source whose magmatic heat still supplies the Geysers geothermal field (Figure 1B) (Hearn et al., 1981; Donnelly-Nolan et al., 1993; Stanley and Blakely, 1995; Mangan et al., 2019), as well as by periodic injections of more mafic magmas from deeper mantle sources (Hearn et al., 1981).
The youngest eruptions of the CLVF comprise a series of maar craters at the edges of, and within, the lake itself (Figure 2). Pyroclastic deposits associated with this activity drape older lava flows and domes around the southeastern half of the lake (Donnelly-Nolan et al., 1993; Hearn et al., 1995). Maars are volcanic craters which form when ascending magma interacts with external water, or water-rich rocks and sediment, in the shallow (<1,000 m) subsurface (Valentine et al., 2011, 2014; Houghton et al., 2015; Zimanowski et al., 2015; Németh and Kósik, 2020). This interaction results in rapid transfer of heat and flash-boiling of the water, fragmentation of magma, and a water-vapor driven explosion aboveground (White and Ross, 2011; Ort et al., 2018). Such phreatomagmatic activity can occur in areas with abundant groundwater and/or surface water such as ponds and lakes (Houghton et al., 2015; Zimanowski et al., 2015). The resulting physical features can range from craters excavated into the current ground surface to tuff rings or tuff cones built above the surface (Lorenz, 1986; Brand and Heiken, 2009; White and Ross, 2011; Graettinger et al., 2014). Processes during maar eruptions can vary from largely steam-driven (lacking a juvenile component in deposits) (Barberi et al., 1992; Pardo et al., 2014; Houghton et al., 2015; Zimanowski et al., 2015), to phreatomagmatic (in which juvenile material makes up a significant component of deposits) (Pardo et al., 2014; Valentine et al., 2014, 2017; Houghton et al., 2015; White and Valentine, 2016), to Strombolian (magma-dominated) (Houghton and Hackett, 1984; Kokelaar, 1986; Gutmann, 2002), and even to Surtseyan (eruptions occurring through bodies of water, often displaying characteristic “rooster-tail” plumes) (Cole et al., 2001; Németh et al., 2006; Murtagh and White, 2013; Gjerløw et al., 2015; Verolino et al., 2019). Maar eruptions may not only produce locally significant tephra-fall deposits (Brand and Heiken, 2009; Valentine et al., 2015; Fierstein and Hildreth, 2017; Ort et al., 2018), ballistics (Self et al., 1980; Mastin and Witter, 2000; Taddeucci et al., 2010; Ort et al., 2018; Graettinger and Bearden, 2021), and ballistic curtains (Melosh, 1986; Graettinger et al., 2015a, 2015b; Valentine et al., 2017), but may also create a variety of dense and dilute pyroclastic density currents (PDCs) (Fisher and Schmincke, 1984; 1998; Cas and Wright, 1988; Lirer and Vinci, 1991; Giaccio et al., 2007; Moore et al., 1966; Fisher and Waters, 1970; Schmincke et al., 1973; Fisher, 1979; Walker, 1984; Moorhouse and White, 2016). Such tephras are found in multiple locations associated with maars in the CLVF, and it is these deposits which form the basis for this study.
This study focuses on three main questions: 1) Where did maar eruptions occur in the CLVF, and how many were there? 2) When did this phreatomagmatic activity happen? and 3) What processes characterized these eruptions? This last question is particularly important at Clear Lake because not only are there many year-round residents of, and recreational visitors to, the lake and its surrounding area (an average daily population of 18,000 people in 2010; Abdollahian et al., 2018), but the region is also heavily developed for wine grapes and other fruit crops (Smith and Broderson, 1989). Residents, infrastructure, agricultural, water, and recreational resources would be at significant risk in the event of explosive phreatomagmatic activity. Additionally, any explosive eruptions in the Clear Lake area have the potential to disrupt major transportation routes and create tephra hazards that affect air traffic in the Bay Area and beyond (Mangan et al., 2019).
In Hearn et al. (1981, 1995) early geologic mappers described a suite of undifferentiated “young pyroclastic” (yp) deposits, including “well-bedded ash, tuffs, and lapilli tephra, mainly consisting of either hydrothermally altered scoria, fresh basaltic andesite or andesite scoria, and fragments of both local lava flow units and Franciscan or Great Valley Sequence bedrock” (Hearn et al., 1995). These deposits were interpreted in some locations as tephra fall deposits connected to visible volcanic vents, and in others as maar deposits (based on the presence of mud-armored lapilli and bomb sags). Their sources were inferred to be “1) vents suggested by arcuate segments of the Clear Lake shoreline, 2) the vent beneath the Little Borax Lake maar, 3) a possible vent north-northwest of Bell Mine; and 4) cinder cones east of Clearlake Oaks” (Hearn et al., 1995). None of the yp deposits were dated directly, but radiocarbon dating of lake sediments provided age constraints on volcanic ash found in lake cores (Hearn et al., 1981, 1995; Sims et al., 1988), suggesting that a significant amount of maar activity may have occurred between 8,000 and 40,000 years BP. Indigenous (Pomo and other) oral histories also contain descriptions which could be interpreted as volcanic phenomena, suggesting that at least some of the explosive activity could have occurred during early human occupation (<12,000 years BP; Mauldin, 1972, Mauldin, 1977; Parker, 2007, Parker, 2008, Parker, 2012; Mauldin, 2018). The relatively young ages of explosive volcanic activity in the CLVF make documenting the eruptive history crucial to accurate hazard assessment and appropriate monitoring for future volcanic unrest and eruptions.
Basic bathymetric surveys of Clear Lake conducted by (Finnegan et al., 1948; Sims et al., 1988; Manson, 1989) suggested the presence of at least 11 volcanic craters, inferred to be maars given that the lake predates activity in the volcanic field, and that all of the craters are located in or very near the lakeshore (Sims, 1976; Sims et al., 1988). Many of these craters are associated with arcuate shorelines, and in one case (Little Borax Lake, now part of the Buckingham Golf Course) even host their own bodies of water (Figure 2). At least seven of these craters intersect known faults as mapped in the USGS Quaternary Faults Database (U.S. Geological Survey and California Geological Survey, 2022). Given the fault-bounded geometry of the paleo-lakeshore of 3.5–8 ka (Figure 2, dotted white line) (Sims et al., 1988), it is reasonable to expect that more maars may be hidden beneath the waters of Clear Lake. An ongoing bathymetric survey (California Natural Resources Agency, 2020) will collect cm-scale measurements and sub-bottom soundings of the current lake floor, and will help shed light on any remaining, hidden craters.
Outcrops were surveyed and sampled over multiple months from 2019 to 2021 during both wet and dry field conditions; wet conditions made it easier to identify the characteristic layering and texture of maar deposits. One of the important tasks of this study was to differentiate between maar deposits and pyroclastic deposits associated with on-shore scoria cones and lava flows. In the field, this was mainly accomplished through componentry; maar deposits contained distinctive vesicular juvenile tephra as well as a variety of local lavas and basement rock, while other pyroclastic deposits were monolithologic and their clasts could be petrologically correlated with nearby lava flows. Samples were taken from each outcrop [labeled “S (#)” in Figure 2] based on visible layering, compositional, and grain size changes grading. In the case of single layers of a particular facies (discussed in the Results section), the layer was usually sampled separately, whereas intervals composed of multiple repeating layers of the same facies were sampled en masse. In the lab, samples were dried in a 40°C oven for at least 2 h and sieved in 1-ϕ increments from -4 to 6ϕ (63–0.063 mm), according to suggested best practices in (Walker, 1971; Buller and McManus, 1973; Lirer and Vinci, 1991; IVHHN, 2005). Sorting parameters (Inman, 1952; Folk and Ward, 1957) were calculated using the GRADISTAT Excel statistics package (Blott and Pye, 2001).
Stratigraphic columns were constructed based on field observations of componentry and depositional structures as well as calculated grain-size-distribution (GSD) analyses. They are divided into four groups based on their geographic location along the southeastern shore of the lake (Figure 2): the Soda Bay Group on the west side of Buckingham Peninsula (S2, S3, S4, S12), the Riviera West Group on the east side of Buckingham Peninsula (S5, S11), the Konocti Bay Group (S8, S13, S14, S15), and the Lower Lake Group (S6). (S7, S9, and S10 were deposits later determined not to be of maar origin, but are shown on the map for numbering clarity. S1 contains pyroclastic deposits related to an overlying scoria cone but was not sampled for stratigraphy). Carbon was recovered from deposits at three locations: the far southern end of the lake (S6, Lower Lake Group), the Konocti Bay area (S13, Konocti Bay Group), and the Riviera West area (S12, Riviera West Group). This material was found both as black, 1–2 mm-thick, continuous layers (S12), and as black films adhering to dispersed clasts (S6 and S13). No clear organic structures were identifiable, but the presence of carbon was confirmed by dissolving the surrounding ash and pyroclasts in HF and then testing the remaining black material for a gas evolution reaction to H2O2. Radiocarbon analyses were conducted by the University of Georgia Center for Applied Isotope Studies; detailed methods are included in the Supplementary Material.
Exposures of maar deposits are anywhere from a few tens of centimeters to multiple meters thick, and their bedding is often inclined and draped over the underlying topography (especially older lava flows). Deposits contain any combination of vesicular to dense, pale-colored tephra; fragments of older CLVF lavas; and other basement bedrock, including the Franciscan Complex and Great Valley Sequence mentioned in Geology of the Clear Lake Volcanic Field. For stratigraphic description purposes, layers where juvenile tephra dominate are referred to as “juvenile,” while those composed mainly of older lavas or basement are referred to as “lithic.” Layers in which neither lithic clasts nor juvenile tephra dominate are described as “mixed.” For size considerations, layers in which particles are coarse-ash-sized or smaller are referred to as “ash” regardless of lithology. Deposits were described as either clast- or matrix-supported, with lithic-dominated units more likely to be clast-supported. Bombs were commonly observed, but no clear bomb sags have been recorded. Depositional structures are rarely observed, and are limited to bedding, pinch-and-swell structures, lenses of larger or smaller clasts, and planar laminations. In many places outcrops are heavily oxidized, both within the deposits and in soil on top of the deposits. In others, there are streaks or lenses of oxidation within individual layers. There are, however, no places where soil development is observed between deposit layers, suggesting that all outcrops represent single eruptive periods, which (in maar eruptions) typically last for hours to months (Ort et al., 2018). For reference, soil production rates in arid or semi-arid climates have been estimated at 30–300 m/My, meaning it could take hundreds to thousands of years for soil layers to develop (Pelletier, 2017).
Juvenile tephra, which are commonly angular to subangular, contain anywhere from 10-40% vesicles and microvesicles of 0.5-3 mm in glassy, tan-to-gray matrices with sparse phenocrysts and microphenocrysts; 1-3mm glomerocrysts of plagioclase and other minerals are occasionally present. Juvenile clasts also contain up to 10% lithic xenoliths, and they are more common in the less vesicular clasts. The surface and vesicles of both juvenile and lithic clasts often carry black films of carbon, discussed further in Radiocarbon ages. The matrices of deposits rich in juvenile clasts are often markedly oxidized, especially near the top of an outcrop, but show no evidence of soil development. A single sample from a glassy bomb at S2 (Soda Bay) yielded an andesitic composition (62% SiO2, 18% Al2O3, 4% FeO, 4.6% Na2O+K2O), consistent with the youngest andesite-to-basaltic andesite terrestrial lava flows and scoria in the CLVF (Hearn et al., 1981; Donnelly-Nolan et al., 1993). A more thorough geochemical investigation of deposit compositions is underway.
Grain-size analysis of the maar deposits and field observations were used to classify individual layers in outcrops by their potential mechanisms of formation (Figures 3A,B). These mechanisms include “Dense PDC,” corresponding to Walker’s “Flow” field (Walker, 1971, 1984) and characterized by very poor sorting, dominantly lapilli or larger grain sizes, and bimodal, or two-peaked, GSD; “Dilute PDC,” corresponding to Walker’s “surge” field and characterized very poor sorting, dominantly ash or smaller grain sizes, and bimodal GSD); “Fall” (poorly-to-moderately-well-sorted, smaller grain sizes, unimodal GSD; and “Ballistic curtain’ (Melosh, 1986; Graettinger et al., 2015b), characterized as massive and poorly sorted, with bimodal grain-size distributions and a wide range of clast sizes. The σϕ parameter is used to determine sorting; σϕ < 2 is well-sorted, while σϕ > 2 is poorly sorted (Inman, 1952; Folk and Ward, 1957).
FIGURE 3. (A) Median clast size and sorting parameters for all sampled deposits categorized by mechanism of formation. Pyroclastic deposit fields of Walker (1971, 1984) are overlain on (A), with ‘surge’ being roughly equivalent to dilute PDCs. (B) shows the cumulative weight percent curves for Clear Lake deposits based on their interpreted mechanism of formation. Note that many of these fields overlap due to both shared grain-size characteristics of the deposits; the presence of volcanic bombs in some layers may also skew data.
In general, this study’s “fall” deposits fit well within Walker’s field for fall deposits (Figure 3A), though their median grain sizes are often skewed into the lapilli (Figure 3B: 2 to -6 ϕ, or 2–64 mm; White and Houghton, 2006) rather than smaller (ash-sized) clasts. Deposits categorized as ‘ballistic curtain’ occupy a similar moderately-to-poorly-sorted space to fall deposits, but their median grain sizes are skewed even farther to -ϕ sizes by the inclusion of blocks and bombs (Figure 3B, >-6ϕ or 64 mm). Deposits classified as ‘Dense PDC’ fall partly within Walker’s pyroclastic flow field, but the fact that many are ash-poor skews some to the larger ϕ sizes and produces a bimodal field of cumulative weight % (Figure 3B). Deposits classified as ‘Dilute PDC’ match very well with Walker’s pyroclastic surge field and tend to be skewed toward larger median grain sizes than Dense PDC deposits (Figure 3A).
Based on clast abundances, depositional structures, and GSD analysis, the following facies designations were assigned and illustrated in Figure 4. Layers noted in photos and facies descriptions correspond to Figures 5–8. (These facies are subject to revision as ongoing stratigraphic mapping, componentry, and textural analyses are conducted):
• Facies 1 (Figure 4A): Lithic-dominated, poorly-sorted, clast-supported, lapilli-to-bomb median clast sizes, containing mostly country rock (Figure 5, S3, layers 011B and 011A). These deposits are often found at the base of outcrops and are composed mainly of unconsolidated, ash-poor, angular clasts of local lava flows and Franciscan Complex lithics; juvenile tephra is sometimes present in sparse amounts (<10%).
• Facies 2 (Figure 4B): Massive, ash-to-bomb median clast sizes, poorly-to very-poorly-sorted, matrix-to-ash-supported, juvenile or mixed lithic and juvenile deposits lacking grading or other structures (Figure 6, S5, layer 026; Figure 7, S13, layer 078; Figure 7, S14, layer 091). These deposits have bimodal grain-size distributions.
• Facies 3 (Figure 4C): Repetitive, reverse- or normal-graded, moderately-to-poorly sorted, mixed lithic and juvenile or juvenile-dominated, ash-to-lapilli median clast sizes, matrix-supported, sometimes with lenses of larger clasts (Figure 5, S2, layer 007; Figure 7, S12, layer 053).
• Facies 4 (Figure 4D): Thin, ash-dominated, moderately-to-poorly sorted, evenly laminated layers (Figure 7, S12, layer 062; Figure 7, S14, layer 088).
• Facies 5 (Figure 4E): Thin, ash-dominated, poorly-to-very-poorly-sorted layers, sometimes underlain by continuous layers of black carbon (Figure 8, S6, layer 038; Figure 7, S12, layer 054).
• Facies 6 (Figure 4F): Massive, poorly sorted, juvenile-dominated layers, either clast- or matrix-supported (Figure 8, S6, layer 037; Figure 7, S15, layer 092). These deposits are similar in sorting and clast sizes to Facies 2 deposits but lack either local lavas or basement rock (lithics).
FIGURE 4. Examples of maar facies. (A) Facies 1, S2, layer 011A. This facies is often totally unconsolidated and eroded into undercuts. (B) Facies 2, S5, layer 026, showing significantly larger clasts than the layers above. (C) Facies 3, S12, layer 053, showing an interval of at least five reverse-graded layers. (D) Facies 4, S12, layer 062; ashy layers with clear laminations. (E) Facies 5, S12, layer 054. Ashy layers lacking laminations, but with irregular bases underlain by thin carbon layers. (F) Facies 6, S6, layer 037. Massive ungraded juvenile lapilli, with brighter clasts having been sliced open during scraping. Field notebook is 11.5 cm × 15.5 cm, hammer is 28 cm tall, scraper is 34.5 cm tall. Image contrast, brightness, and shadows/highlights have been enhanced to emphasize the features of each facies.
FIGURE 5. Stratigraphy of outcrops in the Soda Bay area of the CLVF, west of the Buckingham Peninsula. Average grain size is denoted by the horizontal width of the column (shorter = finer grain size, longer = coarser grain size), and relative clast sizes are also shown in the drawings. Layer widths are also scaled relative to the dominant grain size in the deposit (i.e., smaller grain sizes are narrower, larger are wider). Grain size distributions are shown to the right of each column and are numbered by sample. Facies designations are shown to the left of each column. Locations where carbon was sampled (for example, S12 layer 063) are marked by a “C.” Columns are ordered by their geographic progression from west to east; no correlations or relationships to source vents are implied by this order.
FIGURE 6. Stratigraphy of outcrops in the Riviera West housing development on the east side of the Buckingham Peninsula. See Figure 5 caption for interpretation notes. Columns are ordered according to their geographic progression from west to east; no correlations or relationships to source vents are implied by this order.
FIGURE 7. Stratigraphy of outcrops near Konocti Bay east of Mount Konocti. See Figure 5 caption for interpretation notes. Columns are ordered according to their geographic progression from north to south; no correlations or relationships to source vents are implied by this order.
FIGURE 8. Stratigraphy of an outcrop at the southeast end of Clear Lake, just east of Thurston Lake. See Figure 5 caption for interpretation notes; no correlations or relationships to source vents are implied.
The stratigraphy of 11 outcrops around Clear Lake is described in the following sections, divided into groups based on their locations. The location of each outcrop is labeled and corresponds to Figure 2. Grain-size distributions in 1-ϕ increments are linked to their corresponding layers, and facies designations are noted for each layer analyzed. Some layers were inaccessible for sampling but are represented visually in order to record the stratigraphy of the full outcrop. S7, S9, and S10 are not described here because their maar origin is in question (they appeared to be largely monolithologic and may be associated with other eruptive vents in the Clear Lake Volcanic Field).
The Soda Bay Group (Figure 5), located on the northwest flank of Mount Konocti, is associated with one of the most well-defined maars at Clear Lake, containing at least two craters. Soda Bay is so named because it is a major source of carbon dioxide degassing, which can be observed as bubbles on the surface of the lake (Mauldin, 1991; Bergfeld et al., 2001). The outcrops consist of multiple sets of repetitive reverse- and normal-graded layers, which contain a similar suite of juvenile material in the form of vesicular and dense tephra, and a lithic assortment drawn from both local lava flow layers and the underlying Franciscan Complex metamorphic rocks. Layers 03B to 006 of S2 (Figure 5) contain distinct lenses of oxidized clasts. S2 and S12 are both dominated by Facies 3 deposits (Figure 5, layers 03A to 007, 009 and 010, S2; layers 052, 053, 055, 059, 062, 065, S12), while S3 and S4 contain more varied facies. There are several very poorly-sorted PDC deposits at S2 and S3, and these locations also have lithic-rich bases composed of local lavas (Facies 1; Figure 5, S2 layer 03A, S3 layer 011A). The S2 and S3 outcrops are located in the tephra ring(s) of the Soda Bay maar(s), which makes Soda Bay their most likely eruptive source.
The outcrop at S12, which is located near the edge of the Black Forest landslide on the Buckingham Peninsula (see Figure 2), has the largest continuous sequence of maar deposits, measuring 5+ m in thickness after correction for slope. It preserves examples of most of the maar facies designated in the CLVF, including thick, repetitive reverse-graded layers (Facies 3), massive pyroclastic density current layers (Facies 2), and both laminated (Facies 4) and non-laminated (Facies 5) ash layers. Facies 5 layers (Figure 5, S12, layers 054, 057, 061, 063) are notable for their crenulated bottoms and the distinct layers of black carbon (see Results for more detailed carbon photos). The outcrop appears to end abruptly at the edge of the Black Forest landslide, but it is impossible to tell whether this is because its remainder was removed by the landslide or simply because it pinches out; the landslide itself has been mapped but not dated (Manson, 1989). The most likely source for the deposits at S12, based on proximity, is the Little Borax Lake maar (Figure 2, now hosting the Buckingham Golf Course pond). The location appears to sample directly from the tephra ring surrounding the maar.
On the eastern slopes of Mount Konocti, the Riviera West Group shows the same sort of repetitive reverse-graded layering (Facies 3, Figure 6, S5 and S11) found in the Soda Bay Group. However, the clast sizes in both of these outcrops are skewed heavily toward lapilli and bombs; S11 in particular contains some of the largest bombs (up to 30 cm in diameter) found in this study (Figure 6, S11, layers 048, 049, 051). This is notable because the outcrop is located more than 250 m above the water on the flank of Mount Konocti, suggesting a very high energy of emplacement. While the deposits at S11 are largely unimodal, they are also very poorly sorted. S5 also has a poorly-sorted base with large clasts and vesicular juvenile bombs (Facies 2, S5, layer 026), but subsequent layers are more comparable in thickness and clast size to nearby Soda Bay Group or Konocti Bay Group deposits. The most likely source of eruption for both deposits is the maar mapped immediately to the southeast of Little Borax Lake; however, bathymetry may reveal additional maar craters in this area.
The Konocti Bay Group (Figure 7) is composed of several relatively thick sections of massive Facies two lithic-and-juvenile layers (Figure 7, S13, layer 081; S14, layers 082, 082), interlayered with the typical reverse- and normal-graded repetitive sequences of Facies 3 (S13, layers 074, 075, 080), laminated ash-dominated layers of Facies 5 (S13, layer 073; S14, layers 083 and 085); and the occasional poorly-sorted layers with lithic-dominated lapilli-to-bomb-sized clasts (Facies 1: S13, layer 068; S14, layer 084 and 086). Oxidized lenses are present in layer 078 (S13). Out of all the outcrops, S15 is notable for its massive juvenile-dominated layers (Facies 6, layers 092 and 095). The Konocti Bay Group is important from a volcanic hazards standpoint; some of the deposit locations (S8, S14; Figure 2) are found almost 5 and 3 km distant (respectively) from the Konocti Bay maar, showing that significant tephra fall and possibly pyroclastic density currents reached at least that distance from their originating crater(s).
One outcrop in this group (Figure 7, S8) shows significantly different characteristics compared to other locations in this area (S13, S14, S15), and for this reason its origin as a maar deposit is in question. Composed of one variable-thickness, ash-dominated layer (S8, layer 042, Facies 4) and several layers of matrix-supported, poorly-sorted, lapilli-to-ash-sized pyroclasts (layers 041, 043 and 044), this exposure’s matrix is heavily oxidized and extremely well consolidated. The large ashy layer (042) also fills a trough and tapers from 2 m to one thick at either end of its exposure. Because of extensive oxidation staining and the difficulty of processing the (possibly) welded samples, the lithology and geochemistry of deposits at this sampling location are pending. However, it should be considered whether this deposit is not from one of the maars, but rather an earlier eruption; the dacite of Plum Flat (dpf) (Hearn et al., 1995), which contains pyroclastic density currents sourced from vent eruptions as well as lava dome and lava flow front collapses, is located about 0.5 km to the northeast of this outcrop, and may be a candidate.
The Lower Lake Group (Figure 8) currently is defined by a single outcrop (S6) but is so named because other as-yet unsurveyed but related outcrops exist in the area. The existing stratigraphy is a combination of alternating lithic- (Facies 1) and juvenile-dominated layers (Facies 6), with at least one normally graded sequence (Facies 3, layer 035) containing distinct oxidized lenses. One juvenile-rich layer (033) also contains many clasts coated with carbon films, providing one of the radiocarbon dates discussed in Radiocarbon ages. All of the layers here are poorly-sorted, but only one ash-dominated layer shows a clear bimodal distribution (layer 036, Facies 5). The source location of these deposits is unclear; there is at least one nearby eruptive vent on land directly to the northwest (dt, dacite of Thurston Lake) (Hearn et al., 1995), but it is a dacitic center and not andesitic, as the maar tephra is interpreted to be based on preliminary geochemical analysis. There may also be as-yet hidden maar craters at the southeastern end of Clear Lake, given the heavily scalloped shoreline north of Thurston Lake.
Eight well-calibrated (relative area under 2σ probability distribution >0.7; Reimer et al., 2020) radiocarbon dates were obtained from samples at S6 (Figure 8, Lower Lake Group), S12 (Figure 5, Riviera West Group), and S13 (Figure 7, Konocti Bay Group); the six dates with the greatest area under the 2σ probability distribution are used in Figure 9C, with their corresponding layers noted on the x-axis. The oldest date is 13,250 ±260 cal yr. BP (S6) and the youngest date is 9,030 ±20 cal yr. BP (S13), however most dates fall between about 11,500 and 13,500 cal yr. BP. Full radiocarbon analyses data, including uncalibrated ages and calibration statistics, are reported in the Supplementary Table S1; S12 has two sets of radiocarbon dates because several samples were re-run due to poor calibrations in the initial results. In Figure 9C, radiocarbon dates from this study are compared with age ranges for volcanic ash in lake cores as estimated from sediment accumulation curves in previous work (Sims, 1976; Sarna-Wojcicki et al., 1988; Sims et al., 1988). However, since the radiocarbon dates constraining the sediment curves were originally uncalibrated and it is unclear whether δ13C corrections were applied, the lake core ash ages are rough estimates only.
FIGURE 9. Examples of layered carbonized material at S12 (A, B), as well as the distribution of calibrated radiocarbon dates (C) with error bars indicating 2σ cal BP ranges. Ages of volcanic ash (tephra) in lake cores as estimated from sediment accumulation curves (Sims and Rymer, 1975c, 1975a, 1975b, 1975d; Sims, 1976; Sarna-Wojcicki et al., 1988) are shown in black, and the K-Ar age of the andesite of Sulphur Bank (one of the youngest dated lava flows; White and Roberson, 1962) is shown in blue.
This suite of radiocarbon dates confirms that there was maar volcanism occurring in the CLVF as early as 9,000 yr. BP (Figure 9C). This is coincident with the youngest date of about 8,000–9,000 cal yr. BP estimated for tephra layers in lake cores 73–7 (northeast of Konocti Bay) and 73–8 (northwest end of Clear Lake). The oldest 14C dates at S12 (13,170 ±50 cal yr. BP) and S6 (13,250 ±260 cal yr. BP) could correlate with lake core tephra ages of about 14,000–15,000 cal yr. BP in core 73–1 (northwest of Soda Bay) and 73–6 (Clearlake Oaks arm), given the large errors associated with those tephra ages. Second, these dates indicate that maar volcanism occurred at multiple times at different locations. While S12 and S13 are located within 3 km of each other, S6 and S12 occur during the same 1,500-year time frame more than 10 km apart, though they are both co-located with segments of the Konocti Bay Fault Zone (Figure 2). For this reason, unraveling any stratigraphic correlations between clustered outcrops may be difficult unless there is both geochemical and radiometric evidence that the layers were produced by a single maar.
S12’s range of radiocarbon dates highlights potential sources of uncertainty for the radiocarbon method used in the CLVF; while the stratigraphy at S12 appears to represent multiple explosions within a single eruptive period, the resulting radio carbon dates vary by several thousand years. As previously stated, all of the sample locations surveyed for this study likely represent individual eruptive episodes on the timescale of days to months, as evidenced by the lack of soil development between layers. It is possible that local contamination from groundwater and/or other sources (CO2 gas dissolved in local waters and creating carbonate sediments; Robinson et al., 1988) caused the variance in ages from this single outcrop, given that it was excavated from a roadcut at the base of a steep slope (a natural location for water drainage).
The stratigraphy of CLVF maar deposits is complicated and there are no clear patterns linking the different outcrops, other than that lithic-rich deposits tend to be located at the base of outcrops (i.e., representing early stages in the maar-eruptive process). The suite of radiocarbon dates shown in Figure 9C does correlate, in part, with several of the tephra ages derived from lake cores 73–1, 73–6, 73–7, and 73–8 (Figure 9C, black markers), which bolsters the evidence for at least two periods of maar eruptions. It may be possible to derive some information about the aereal extent of tephra deposition from these lake cores if additional work on subaerial tephrachronology is combined with detailed chemical and textural examination of the volcanic ash found in lake core sediments.
However, because of the close proximity of many of the known maar craters to each other, and the typical characteristics of maar eruptions (multiple eruptions from a single crater, migrating craters, or even multiple crater formation) (Graettinger et al., 2014; Sonder et al., 2015; Valentine et al., 2017; Graettinger and Bearden, 2021), it is possible that individual stratigraphic sections record not only multiple explosive events from single or even multiple craters in the same eruption, but also interlayered deposits from multiple maars, if there were coeval eruptions in the CLVF. Additional radiocarbon dating, as well as systematic geochemical and textural analyses of tephra are the necessary next steps in unraveling these relationships. Even that task may be complicated if the juvenile material is geochemically homogenous as a result of a shared magma source or short durations between eruptions.
While correlation of individual layers in the CLVF is difficult, the commonly observed facies at all of the outcrops can be interpreted in terms of possible eruptive processes based on their componentry and grain-size distribution analyses.
Because Facies 1 deposits are composed primarily of lithics sourced from either local lava flows or deeper metamorphic rocks, they are interpreted as crater-forming explosions early in the eruptive, maar-forming process. Alternatively, if they are found stratigraphically higher in an outcrop, these deposits could be the result of vent-clearing following collapse of crater walls (Lorenz, 1986; Graettinger et al., 2015a). The lack of juvenile ash and larger tephra clasts indicates that magma had probably not yet reached the surface during Facies 1 formation.
Facies 2 and 6 may represent the “ballistic curtains” described in Graettinger et al. (2015b) after bolide impact ejecta descriptions by Melosh (1986). Such deposits are created by directed jets of material in an explosion, often angled, and may be localized in “rays” (which are difficult to distinguish in isolated outcrops). Facies 2 contains both juvenile and lithic material, suggesting that explosions were either still expanding the crater, clasts were being recycled in the crater (Houghton and Smith, 1993), or crater-wall collapse was occurring. Since Facies 6 is dominated by juvenile material, it may represent a similar process occurring during a more magma-dominated phase of the eruption, possibly even a Strombolian eruption style with limited magma-water interaction (Self et al., 1980; Wohletz, 1983; Houghton and Hackett, 1984; Murtagh and White, 2013).
Facies 3 deposits, with their distinctive graded appearance and mixed juvenile and lithic contents, probably represent the repeated, pulsatory explosions characteristic of maar eruptions in their phreatomagmatic phases (Self et al., 1980; Taddeucci et al., 2010; Houghton et al., 2015; Sonder et al., 2015). The poorly-sorted nature of these deposits ties them to the more turbulent processes of pyroclastic density currents, although potentially from less powerful explosions. .
Facies 4 and 5 deposits are similar in componentry and overall grain size but are primarily distinguished by their sorting and depositional structures (or lack thereof). Facies 4 deposits show poor-to-moderate sorting, suggesting that this facies either represents ash fallout largely unaffected by turbulent processes (Walker, 1971; Fisher, 1979; Graettinger et al., 2014; Ort et al., 2018). Facies 5 deposits, which are poorly to very-poorly-sorted but ash-dominated, could represent dilute pyroclastic density currents, although the lack of characteristic structures (ripples, dunes, chute-and-pool) (Fisher and Waters, 1970; Schmincke et al., 1973; Walker, 1984) makes it difficult to characterize these specifically as the “surges” or “base surges” discussed by some previous workers (Moore et al., 1966; Fisher and Waters, 1970; Moorhouse and White, 2016). This facies may also represent the turbulent clouds accompanying more concentrated PDCs. The presence of carbon (Figures 9A,B) beneath many of these deposits is, however, a puzzling association. There is no indication these PDCs were hot, so the material is probably not carbonized (i.e., charred) organic matter due to high temperatures. Explosions could have disrupted carbon-rich materials such as peat or carbonaceous sediment on the floor of Clear Lake (Sims et al., 1988; Sims et al., 1988), but the mechanism for depositing them in such coherent layers is unclear.
Prior to the 1830s, the Clear Lake basin was primarily home to various groups of Pomo people, including the Elem, Koi, Kamdot, Lileek, Habenapo, Kuhlanapo, Komli, Boalke, Kaiyao, Yobotui, Howalek, Danoha, and Shigom communities (Kniffen, 1939). The oldest archaeological sites in the Clear Lake basin have been dated between 14,000 and 20,000 years (White and Fredrickson, 2002; Parker, 2007, 2008, 2012). In addition to natural resources in and around the lake, the area was known for its role in the obsidian trade, particularly from sites in the rhyolite flows of Thurston Creek (rto) (Hearn et al., 1995; Hodgson, 2005, 2007). Thus, the volcanic history of the CLVF is closely tied with the history of the indigenous groups who still dwell there, and the radiocarbon dates in this study indicate that their experiences may contain clues to past volcanic processes and hazards associated with maar volcanism.
A preliminary examination of oral histories collected by Henry Mauldin of the Lake County Historical Society from local indigenous historians (Mauldin, 1972, 1977; Mauldin and Museums of Lake County, 2018) identified many references to phenomena which may be contemporary interpretations of explosive volcanic activity:
• “After Graysquirrel was safely in Coyote’s house there was a terrific explosion and Rock blew to pieces. From this came all the loose boulders of the world” (From “Rocks of the world,” a story about Graysquirrel and Rock Man by Francisco John, as told to Henry Mauldin)
• “Nu-coo-ee noticed a place under Rock Man’s arm and hit him there with the sphere. The world started to shake and with the noise of thunder. The people heard it and started packing up” (From “The Story of Nu-Coo-Ee” as told to Henry Mauldin by Harris Holms of the Big Valley Rancheria near Finley)
• “Kah-bel also was now full of wrath and shouted angrily at Konocti. They argued long and loud and were soon hurling great boulders at each other across the water … On the shore of the Lake east of Soda Bay there may be seen many boulders which fell short of their mark …” (From “The Battle of The Giants” by Francisco John as told to Henry Maudlin)
• “After [Nu-Coo-Ee] sang he threw his spear into the air and while it was up there it gave off fire and sparks, which showed his power” (From “The Story of Nu-Coo-Ee” as told to Henry Mauldin by Harris Holms of the Big Valley Rancheria near Finley)
These descriptions are consistent with maar-type activity, in which the ‘fire and sparks’ of an eruption might be seen over hilltops (depending on the observer’s location around the lake) and ‘great boulders’ (volcanic bombs) could be thrown high above an eruption crater.
This coincidence of geologic events and oral histories may present an important avenue of research collaboration with indigenous scholars, to better constrain exact eruption timing and duration as well as to characterize the human dimensions of volcanic hazards at the CLVF. These types of effort to co-produce geologic hazards knowledge (Nunn et al., 2019; Scarlett and Riede, 2019) have resulted in much richer eruption histories at Volcán Tungurahua in Ecuador (Le Pennec et al., 2008); Kīlauea Volcano in Hawaii (Swanson, 2008); Central Java, Indonesia (Griffin and Barney, 2021); Tseax Volcano, British Columbia, Canada (Le Moigne et al., 2022); and the Taupō Volcanic Zone in New Zealand (Pardo et al., 2015). If eruptions did occur during early human occupation of the CLVF, the oral histories preserved by their descendants may contain useful insights about precursors to future eruptions, as well as severity and impacts of past maar eruptions. Both types of information could inform plausible eruption scenarios and hazard zonations for an eventual volcanic hazards assessment at Clear Lake.
Radiocarbon dates of less than 10,000 years B.P. place CLVF maar eruptive activity firmly in the Holocene period, though it is unknown whether future dates will place any eruptions within the past 2,000–3,000 years (indicating a higher-threat volcano following the ranking scheme of Ewert et al., 2005). These ages, in combination with the populations, infrastructure, and transportation routes at risk in the CLVF (Abdollahian et al., 2018; Mangan et al., 2019), reinforce the need for monitoring and hazard assessment. Worldwide, hazards from phreatomagmatic explosions, such as base surges, lahars, and tsunamis, have produced about 20% of all fatalities associated with volcanic activity in historical time (Mastin and Witter, 2000).
While most of the known CLVF maar deposits are found immediately adjacent to Clear Lake itself, some pyroclastic density currents and tephra may have reached up to 5 km from sources, a serious implication for the reach of near-vent eruptive hazards. The Whakaari, New Zealand, eruption of 2019 (Dempsey et al., 2020) illustrates the devastating consequences of being in close vicinity to even relatively small phreatic eruptions. Additionally, maar eruption cloud heights of 6,000+ m and associated ashfall (Ort et al., 2018) have the potential to disrupt regional populations, infrastructure, and transportation (including air traffic) given the proximity of the CLVF to the cities of Lakeport, Clearlake, Ukiah, Santa Rosa, and Napa (Abdollahian et al., 2018; Mangan et al., 2019). The association of known CLVF maar craters (and potentially more beneath the lake) with faults also suggests that future eruptions may occur preferentially along faults. Geophysical analyses (Chapman, 1975; Majer et al., 1991; Stanley and Blakely, 1995; Peacock et al., 2020) indicate that magma is still present at around 5 km below parts of the CLVF, and future intrusions and eruptions in and around Clear Lake are possible. Additional study of the maars and their deposits will be crucial to determining the full extent of potential hazards of future explosive eruptions in the CLVF.
This stratigraphic study has revealed a number of important insights into the young phreatomagmatic history in the Clear Lake Volcanic Field (CLVF):
• Stratigraphic and grain-size analysis of 11 outcrops of pyroclastic material in the Clear Lake Volcanic Field confirms a complicated history of phreatomagmatic eruptions in and around Clear Lake. Deposit features characteristic of maar eruptions (repetitive graded layers, evidence for dilute pyroclastic density currents, and deposits formed by ballistic curtains) help distinguish maar deposits from other young pyroclastic deposits in the CLVF.
• Unraveling individual eruptions in the CLVF is complicated by the characteristics of maar eruptions and the potential for multiple, coeval eruptions. Future geochemical, textural, geochronological, and geographical analyses will help decipher the tangled history of the maars.
• Radiocarbon analysis of carbon found within maar deposits reveals that some eruptions occurred between ∼13,500 and 9,000 cal yr. BP, roughly coincident with the ages of tephra recovered from lake core sediments by previous workers.
Ongoing work to characterize lake bathymetry and the geochemical patterns and evolution of the phreatomagmatic eruptions will provide important insights into the extent, progression, and evolution of maar volcanism in the CLVF. The high-resolution bathymetry in particular will help identify the location and number of maars, and combined with radiocarbon dating, lithological and geochemical analysis of juvenile material, and spatial analysis of vent locations, will help us further expand an existing tephrochronology and eruptive record. There is also much to be learned from geochemical textural analysis of juvenile products, which can elucidate eruption behavior over time and constrain parameters for future eruption modeling of both tephra-fall and ballistic emplacement. Finally, building relationships with local indigenous groups to exchange knowledge of pre-European-contact landscape history will provide an additional insights into the region’s youngest eruptions. Combined, these research tracks will feed into a detailed eruption history and future volcanic hazard assessment for the Clear Lake Volcanic Field.
The datasets presented in this study can be found in online repositories. The names of the repository/repositories and accession number(s) can be found below: USGS ScienceBase: https://doi.org/10.5066/P9OH9DSD.
JB conducted stratigraphic surveys in the field with the assistance of Dawn Ruth (USGS California Volcano Observatory). Laboratory assistance in sample preparation was provided by Nicole Thomas and Grace Hruska (USGS California Volcano Observatory). JB completed sample data collection and statistical analyses, figure production, writing, and interpretation for this manuscript. Radiocarbon analyses were performed by the Center for Applied Isotope Studies at the University of Georgia (https://cais.uga.edu/).
This research was funded by the USGS Volcano Science Center. Any use of trade, firm, or product names is for descriptive purposes only and does not imply endorsement by the U.S. Government.
The author would like to acknowledge the USGS California Coast Range Volcanic Centers Project and its members for field, lab, and research assistance; partners at the UC Davis Center for Regional Change and Tahoe Environmental Research Center (https://clearlake.ucdavis.edu/) for bathymetric survey efforts; the Center for Applied Isotope Studies at the University of Georgia for radiocarbon dating; and Mike Brown of the Elem Indian Colony of Pomo Indians of the Sulphur Bank Rancheria, California for allowing sampling access and taking the time to discuss Pomo oral history. Two journal reviews and three internal USGS reviews greatly improved and focused this manuscript. This work was conducted on the lands of the Elem Indian Colony of Pomo Indians of the Sulphur Bank Rancheria, California, the Big Valley Band of Pomo Indians of the Big Valley Rancheria, California, the Habematolel Pomo of Upper Lake, California, and the Scotts Valley Band of Pomo Indians of California.
The author declares that the research was conducted in the absence of any commercial or financial relationships that could be construed as a potential conflict of interest.
All claims expressed in this article are solely those of the authors and do not necessarily represent those of their affiliated organizations, or those of the publisher, the editors and the reviewers. Any product that may be evaluated in this article, or claim that may be made by its manufacturer, is not guaranteed or endorsed by the publisher.
The Supplementary Material for this article can be found online at: https://www.frontiersin.org/articles/10.3389/feart.2022.911129/full#supplementary-material
Abdollahian, N., Jones, J. L., Ball, J. L., Wood, N. J., and Mangan, M. T. (2018). Data release for results of societal exposure to California’s volcanic hazards (ver. 2.0, September 2018). doi:10.5066/F7W66JRH
Barberi, F., Bertagnini, A., Landi, P., and Principe, C. (1992). A review on phreatic eruptions and their precursors. J. Volcanol. Geotherm. Res. 52, 231–246. doi:10.1016/0377-0273(92)90046-G
Bergfeld, D., Goff, F., and Janik, C. J. (2001). Carbon isotope systematics and CO2 sources in the Geysers-Clear Lake region, northern California, USA. Geothermics 30, 303–331. doi:10.1016/S0375-6505(00)00051-1
Blott, S. J., and Pye, K. (2001). Gradistat: A grain size distribution and statistics package for the analysis of unconsolidated sediments. Earth Surf. Process. Landf. 26, 1237–1248. doi:10.1002/esp.261
Brand, B. D., and Heiken, G. (2009). Tuff cones, tuff rings, and maars of the Fort Rock–Christmas Valley basin, Oregon: Exploring the vast array of pyroclastic features that record violent hydrovolcanism at Fort Rock and the Table Rock Complex. Geol. Soc. Am. Field Guide 15, 521–538. doi:10.1130/2009.fld015(25
Buller, A. T., and McManus, J. (1973). Distinction among pyroclastic deposits from their grain-size frequency distributions. J. Geol. 81, 97–106. doi:10.1086/627810
California Natural Resources Agency (2020). Blue ribbon committee for the rehabilitation of Clear Lake 2020 report to the governor and California state legislature. The consensus and collaboration Program. College of Continuing Education, Sacramento State University. Available at: https://resources.ca.gov/-/media/CNRA-Website/Files/Initiatives/Clearlake- BRC/2020-BRC-Annual-Report-_20200106_FINAL.pdf (Accessed March 30, 2022).
Cas, R. A. F., and Wright, J. V. (1988). “Three types of pyroclastic deposits and their eruption: An introduction,” in Volcanic successions modern and ancient: A geological approach to processes, products and successions. Editors R. A. F. Cas,, and J. V. Wright (Dordrecht, Netherlands: Springer), 92–126. doi:10.1007/978-94-009-3167-1_5
Chapman, R. H. (1975). Geophysical study of the Clear Lake region. CaliforniaSacramento, CA, United States: California Division of Mines and Geology. Available at: https://www.osti.gov/biblio/7300477-geophysical-study-clear-lake-region-california.
Cole, P. D., Guest, J. E., Duncan, A. M., and Pacheco, J.-M. (2001). Capelinhos 1957–1958, faial, azores: Deposits formed by an emergent surtseyan eruption. Bull. Volcanol. 63, 204–220. doi:10.1007/s004450100136
Dempsey, D. E., Cronin, S. J., Mei, S., and Kempa-Liehr, A. W. (2020). Automatic precursor recognition and real-time forecasting of sudden explosive volcanic eruptions at Whakaari, New Zealand. Nat. Commun. 11, 3562. doi:10.1038/s41467-020-17375-2
Donnelly-Nolan, J. M., Burns, M. G., Goff, F. E., Peters, E. K., and Thompson, J. M. (1993). The Geysers-Clear Lake area, California; thermal waters, mineralization, volcanism, and geothermal potential. Econ. Geol. 88, 301–316. doi:10.2113/gsecongeo.88.2.301
Donnelly-Nolan, J. M., Hearn, B. C., Curtis, G. H., and Drake, R. E. (1981). “Geochronology and evolution of the Clear Lake volcanics,” in Research in the Geysers - clear Lake geothermal area. Northern California U.S. Geological Survey Professional Paper 1141, 47–60. Available at: https://pubs.er.usgs.gov/publication/pp1141.
Ewert, J. W., Guffanti, M., and Murray, T. L. (2005). An assessment of volcanic threat and monitoring capabilities in the United States: Framework for a national volcano early warning system (NVEWS). U.S. Geol. Surv. Open-File Rep. 2005-1164, 1–62. doi:10.3133/ofr20051164
Fierstein, J., and Hildreth, W. (2017). Eruptive history of the ubehebe crater cluster, death valley, California. J. Volcanol. Geotherm. Res. 335, 128–146. doi:10.1016/j.jvolgeores.2017.02.010
Finnegan, H. E., Jones, E. E., Keith, H. W., and Rushford, D. G. (1948). U.S. Coast and Geodetic Survey. Clear Lake, California. Available at: https://data.ngdc.noaa.gov/platforms/ocean/nos/coast/H06001-H08000/ H07192B/DR/H07192B.pdf (Accessed June 22, 2022).Descriptive report to accompany hydrographic survey.
Fisher, R. V. (1979). Models for pyroclastic surges and pyroclastic flows. J. Volcanol. Geotherm. Res. 6, 305–318. doi:10.1016/0377-0273(79)90008-8
Fisher, R. V., and Schmincke, H.-U. (Editors) (1984). “Pyroclastic flow deposits,” Pyroclastic rocks (Berlin: Springer-Verlag), 186–230.
Fisher, R. V., and Waters, A. C. (1970). Base surge bed forms in maar volcanoes. Am. J. Sci. 268, 157–180. doi:10.2475/ajs.268.2.157
Folk, R. L., and Ward, W. C. (1957). Brazos River bar [Texas]; a study in the significance of grain size parameters. J. Sediment. Res. 27, 3–26. doi:10.1306/74D70646-2B21-11D7-8648000102C1865D
Giaccio, B., Sposato, A., Gaeta, M., Marra, F., Palladino, D. M., Taddeucci, J., et al. (2007). Mid-distal occurrences of the Albano Maar pyroclastic deposits and their relevance for reassessing the eruptive scenarios of the most recent activity at the Colli Albani Volcanic District, Central Italy. Quat. Int. 171–172, 160–178. doi:10.1016/j.quaint.2006.10.013
Gjerløw, E., Höskuldsson, A., and Pedersen, R.-B. (2015). The 1732 surtseyan eruption of eggøya, jan mayen, north atlantic: Deposits, distribution, chemistry and chronology. Bull. Volcanol. 77, 14. doi:10.1007/s00445-014-0895-6
Graettinger, A. H., and Bearden, A. T. (2021). Lateral migration of explosive hazards during maar eruptions constrained from crater shapes. J. Appl. Volcanol. 10, 3. doi:10.1186/s13617-021-00103-w
Graettinger, A. H., Valentine, G. A., and Sonder, I. (2015a). Circum-crater variability of deposits from discrete, laterally and vertically migrating volcanic explosions: Experimental evidence and field implications. J. Volcanol. Geotherm. Res. 308, 61–69. doi:10.1016/j.jvolgeores.2015.10.019
Graettinger, A. H., Valentine, G. A., Sonder, I., Ross, P.-S., and White, J. D. L. (2015b). Facies distribution of ejecta in analog tephra rings from experiments with single and multiple subsurface explosions. Bull. Volcanol. 77, 66. doi:10.1007/s00445-015-0951-x
Graettinger, A. H., Valentine, G. A., Sonder, I., Ross, P.-S., White, J. D. L., and Taddeucci, J. (2014). Maar-diatreme geometry and deposits: Subsurface blast experiments with variable explosion depth. Geochem. Geophys. Geosyst. 15, 740–764. doi:10.1002/2013GC005198
Griffin, C., and Barney, K. (2021). Local disaster knowledge: Towards a plural understanding of volcanic disasters in Central Java’s highlands, Indonesia. Geogr. J. 187, 2–15. doi:10.1111/geoj.12364
Gutmann, J. T. (2002). Strombolian and effusive activity as precursors to phreatomagmatism: Eruptive sequence at maars of the Pinacate volcanic field, Sonora, Mexico. J. Volcanol. Geotherm. Res. 113, 345–356. doi:10.1016/S0377-0273(01)00265-7
Hammersley, L., and DePaolo, D. J. (2006). Isotopic and geophysical constraints on the structure and evolution of the Clear Lake volcanic system. J. Volcanol. Geotherm. Res. 153, 331–356. doi:10.1016/j.jvolgeores.2005.12.003
Hearn, B. C., Donnelly-Nolan, J. M., and Goff, F. E. (1995). Geologic map and structure sections of the Clear Lake Volcanics, northern California. U.S. Geological Survey Miscellaneous Investigations Map I-2362. Available at: https://pubs.usgs.gov/imap/2362/ (Accessed March 29, 2022).
Hearn, B. C., Donnelly-Nolan, J. M., and Goff, F. E. (1981). “The Clear Lake volcanics: Tectonic setting and magma sources,” in Research in the Geysers-Clear Lake geothermal area, Northern California U.S. Geological Survey Professional Paper 1141, 25–45. Available at: https://pubs.er.usgs.gov/publication/pp1141.
Hodgson, S. F. (2005). Obsidian spirits just speak once”—California Indians in a geothermal land. Available at: https://www.geothermal-energy.org/pdf/IGAstandard/WGC/2005/0204.pdf (Accessed June 22, 2022).Proceedings World Geothermal CongressAntalya, Turkey10.
Hodgson, S. F. (2007). Obsidian: Sacred glass from the California sky. Geol. Soc. Lond. Spec. Publ. 273, 295–313. doi:10.1144/GSL.SP.2007.273.01.23
Houghton, B. F., and Hackett, W. R. (1984). Strombolian and phreatomagmatic deposits of ohakune craters, ruapehu, New Zealand: A complex interaction between external water and rising basaltic magma. J. Volcanol. Geotherm. Res. 21, 207–231. doi:10.1016/0377-0273(84)90023-4
Houghton, B. F., and Smith, R. T. (1993). Recycling of magmatic clasts during explosive eruptions: Estimating the true juvenile content of phreatomagmatic volcanic deposits. Bull. Volcanol. 55, 414–420. doi:10.1007/BF00302001
Houghton, B., White, J. D. L., and Van Eaton, A. R. (2015). Phreatomagmatic and related eruption styles. Encycl. Volcanoes, 537–552. doi:10.1016/B978-0-12-385938-9.00030-4
Inman, D. L. (1952). Measures for describing the size distribution of sediments. SEPM J. Sediment. Res. 22 (3), 125–145. doi:10.1306/D42694DB-2B26-11D7-8648000102C1865D
IVHHN (2005). IVHHN guidelines for grain-size distribution analysis. International volcanic health hazard network. Available at: https://www.ivhhn.org/uploads/es/ivhhn_gsd_guidelines.pdf (Accessed March 30, 2022).
Kniffen, F. B. (1939). Pomo geography, 36. University of California Publications in America Archaeology and Ethnology, 353–400.
Kokelaar, P. (1986). Magma-water interactions in subaqueous and emergent basaltic. Bull. Volcanol. 48, 275–289. doi:10.1007/BF01081756
Le Moigne, Y., Williams-Jones, G., Vigouroux, N., and Russell, J. K. (2022). Chronology and eruption dynamics of the Historic∼1700 CE eruption of Tseax Volcano, British Columbia, Canada. Front. Earth Sci. 10. Available at: https://www.frontiersin.org/article/10.3389/feart.2022.910451 (Accessed June 22, 2022).
Le Pennec, J.-L., Jaya, D., Samaniego, P., Ramón, P., Moreno Yánez, S., Egred, J., et al. (2008). The AD 1300–1700 eruptive periods at Tungurahua volcano, Ecuador, revealed by historical narratives, stratigraphy and radiocarbon dating. J. Volcanol. Geotherm. Res. 176, 70–81. doi:10.1016/j.jvolgeores.2008.05.019
Lirer, L., and Vinci, A. (1991). Grain-size distributions of pyroclastic deposits. Sedimentology 38, 1075–1083. doi:10.1111/j.1365-3091.1991.tb00372.x
Liu, M., and Furlong, K. P. (1992). Cenozoic volcanism in the California Coast ranges: Numerical solutions. J. Geophys. Res. 97, 4941–4951. doi:10.1029/92JB00193
Lorenz, V. (1986). On the growth of maars and diatremes and its relevance to the formation of tuff rings. Bull. Volcanol. 48, 265–274. doi:10.1007/BF01081755
Majer, E. L., Chapman, R. H., Stanley, W. D., and Rodriguez, B. D. (1991). Geophysics at the Geysers. Special report 17. Davis, California: Geothermal Resources Council (now Geothermal Rising). Available at: https://publications.mygeoenergynow.org/grc/1005479.pdf.
Mangan, M., Ball, J., Wood, N., Jones, J. L., Peters, J., Abdollahian, N., et al. (2019). California’s exposure to volcanic hazards. U.S. Geological Survey Scientific Investigations Report 2018–5159, 58.
Manson, M. W. (1989). “Landslide hazards in the eastern Clear Lake area,” in Landslide hazards identification map No. 16 (Lake County, California. Available at: https://filerequest.conservation.ca.gov/?q=ofr_89-27 (Accessed March 29, 2022).
Mastin, L. G., and Witter, J. B. (2000). The hazards of eruptions through lakes and seawater. J. Volcanol. Geotherm. Res. 97, 195–214. doi:10.1016/S0377-0273(99)00174-2
Mauldin, H.,and Museums of Lake County (2018). Mauldin Files. The Mauldin Files: 10,000 Pages of Lake County History. Available at: https://museumsoflakeca.wixsite.com/website/mauldin-files (Accessed March 30, 2022).
Mauldin, H. K. (1972). Two indian legends of lake county, California. Lakeport, California: Lakeport, Calif: Lake County Library Project.
Mauldin, H. (1991). Lake County (California) history. Salt Lake City, Utah: Genealogical Society of Utah.
Mauldin, H. (1977). Lake County Indian lore. Lake County Historical Society. Lakeport, California: Lake County Historical Society, 39p.)
McLaughlin, R. J., and Ohlin, H. N. (1984). Tectonostratigraphic framework of Geysers-Clear Lake region, California. Pacific Section SEPM, 43. Broken Arrow, OK: Franciscan Geology of Northern California, 221–254. Available at: https://archives.datapages.com/data/pac_sepm/059/059001/pdfs/221.htm (Accessed March 31, 2022).
Moore, J. G., Nakamura, K., and Alcaraz, A. (1966). The september 28–30, 1965 eruption of taal volcano, Philippines. Bull. Volcanol. 29, 75–76. doi:10.1007/BF02597143
Moorhouse, B. L., and White, J. D. L. (2016). Interpreting ambiguous bedforms to distinguish subaerial base surge from subaqueous density current deposits. Depos. Rec. 2, 173–195. doi:10.1002/dep2.20
Murtagh, R. M., and White, J. D. L. (2013). Pyroclast characteristics of a subaqueous to emergent Surtseyan eruption, Black Point volcano, California. J. Volcanol. Geotherm. Res. 267, 75–91. doi:10.1016/j.jvolgeores.2013.08.015
Németh, K., Cronin, S. J., Charley, D., Harrison, M., and Garae, E. (2006). Exploding lakes in Vanuatu - “surtseyan-style” eruptions witnessed on ambae island. Episodes 26, 87–92. doi:10.18814/epiiugs/2006/v29i2/002
Németh, K., and Kósik, S. (2020). Review of explosive hydrovolcanism. Geosciences 10, 44. doi:10.3390/geosciences10020044
Nunn, P. D., Lancini, L., Franks, L., Compatangelo-Soussignan, R., and McCallum, A. (2019). Maar stories: How oral traditions aid understanding of maar volcanism and associated phenomena during preliterate times. Ann. Am. Assoc. Geogr. 109 (5), 1618–1631. doi:10.1080/24694452.2019.1574550
Ort, M. H., Lefebvre, N. S., Neal, C. A., McConnell, V. S., and Wohletz, K. H. (2018). Linking the Ukinrek 1977 maar-eruption observations to the tephra deposits: New insights into maar depositional processes. J. Volcanol. Geotherm. Res. 360, 36–60. doi:10.1016/j.jvolgeores.2018.07.005
Pardo, N., Cronin, S. J., Németh, K., Brenna, M., Schipper, C. I., Breard, E., et al. (2014). Perils in distinguishing phreatic from phreatomagmatic ash; insights into the eruption mechanisms of the 6 August 2012 Mt. Tongariro eruption, New Zealand. J. Volcanol. Geotherm. Res. 286, 397–414. doi:10.1016/j.jvolgeores.2014.05.001
Pardo, N., Wilson, H., Procter, J. N., Lattughi, E., and Black, T. (2015). Bridging Māori indigenous knowledge and Western geosciences to reduce social vulnerability in active volcanic regions. J. Appl. Volcanol. 4, 5. doi:10.1186/s13617-014-0019-1
Parker, J. (2012). 20,000 Years of cultural change in the Clear Lake basin. Archaeological Research, Dr. John Parker. Available at: https://www.academia.edu/4138841/20_000_Years_of_Cultural_Change _in_Clear_Lake_Basin_California (Accessed March 30, 2022).
Parker, J. (2007). Cultural resource inspection of the Elem Indian Colony (and A small portion of Sulphur Bank mine). Archaeological Research, Dr. John Parker. Available at: https://www.academia.edu/4138899/Elem_Indian_Colony_Cultural_Resource_Inspection (Accessed March 30, 2022).
Parker, J. (2008). Cultural resource mitigation of the Elem storm drain Project archaeological site CA-LAK-76/H. Archaeological Research, Dr. John Parker. Available at: https://www.academia.edu/4139637/Elem_Indian_Colony_Archaeology_of_Storm_Drain_Project (Accessed March 30, 2022).
Peacock, J. R., Earney, T. E., Mangan, M. T., Schermerhorn, W. D., Glen, J. M., Walters, M., et al. (2020). Geophysical characterization of the Northwest Geysers geothermal field, California. J. Volcanol. Geotherm. Res. 399, 106882. doi:10.1016/j.jvolgeores.2020.106882
Pelletier, J. D. (2017). Quantifying the controls on potential soil production rates: A case study of the san gabriel mountains, California. Earth Surf. Dynam. 5, 479–492. doi:10.5194/esurf-5-479-2017
Reimer, P. J., Austin, W. E. N., Bard, E., Bayliss, A., Blackwell, P. G., Ramsey, C. B., et al. (2020). The IntCal20 northern Hemisphere radiocarbon age calibration curve (0–55 cal kBP). Radiocarbon 62, 725–757. doi:10.1017/RDC.2020.41
Robinson, S. W., Adam, D. P., and Sims, J. D. (1988). “Radiocarbon content, sedimentation rates, and a time scale for core CL-73-4 from Clear Lake, California,” in late quaternary climate, tectonism, and Sedimentation in Clear Lake, northern California Coast ranges. GSA special papers 214. Available at: doi:10.1130/SPE214[Accessed April 21, 2022].
Rytuba, J. J., Hothem, R. L., May, J. T., Kim, C. S., Lawler, D., Goldstein, D., et al. (2009). Environmental impact of the helen, research, and chicago mercury mines on water, sediment, and biota in the upper dry Creek watershed. U.S. Geological Survey Open-File Report 1382, 59p. Lake County, California. Available at: https://pubs.usgs.gov/of/2008/1382/.
Sarna-Wojcicki, A. M., Meyer, C. E., Adam, D. P., and Sims, J. D. (1988). “Correlations and age estimates of ash beds in late Quaternary sediments of Clear Lake, California,” in Late quaternary climate, tectonism, and sedimentation in Clear Lake, northern California Coast ranges. Editor J. D. Sims (Boulder, CO: Geological Society of America). doi:10.1130/SPE214-p141
Scarlett, J. P., and Riede, F. (2019). The dark geocultural heritage of volcanoes: Combining cultural and geoheritage perspectives for mutual benefit. Geoheritage 11, 1705–1721. doi:10.1007/s12371-019-00381-2
Schmincke, H.-U., Fisher, R. V., and Waters, A. C. (1973). Antidune and chute and pool structures in the base surge deposits of the Laacher See area, Germany. Sedimentology 20, 553–574. doi:10.1111/j.1365-3091.1973.tb01632.x
Self, S., Kienle, J., and Huot, J. P. (1980). Ukinrek Maars, Alaska, II. Deposits and formation of the 1977 craters. J. Volcanol. Geotherm. Res. 7, 39–65. doi:10.1016/0377-0273(80)90019-0
Sims, J. D. (1976). Proceedings of the first international symposium on global-scale paleolimnology and paleoclimatology, 45. Kyoto, Japan: University of Kyoto.Paleolimnology of Clear Lake, California, U.S.A.
Sims, J. D., Rymer, M. J., and Perkins, J. A. (1988). “Late Quaternary deposits beneath Clear Lake, California; Physical stratigraphy, age, and paleogeographic implications,” in Late quaternary climate, tectonism, and sedimentation in Clear Lake, northern California Coast ranges. Editor J. D. Sims (Boulder, CO: Geological Society of America). doi:10.1130/SPE214-p21
Sims, J. D., and Rymer, M. J. (1975a). Preliminary description and interpretation of cores and radiographs from Clear Lake. Lake County, California: U.S. Geological Survey Open-File Report, 75–381. Core 5. doi:10.3133/ofr75381
Sims, J. D., and Rymer, M. J. (1975b). Preliminary description and interpretation of cores and radiographs from Clear Lake. Lake County, California: U.S. Geological Survey Open-File Report, 75–569. Core 6. doi:10.3133/ofr75569
Sims, J. D., and Rymer, M. J. (1975c). Preliminary description and interpretation of cores and radiographs from Clear Lake. Core 7. Lake County, California: U.S. Geological Survey Open-File Report, 75–144. doi:10.3133/ofr75144
Sims, J. D., and Rymer, M. J. (1975d). Preliminary description and interpretation of cores and radiographs from Clear Lake. Core 8, 75. Lake County, California: U.S. Geological Survey Open-File Report–306. doi:10.3133/ofr75306
Smith, D. W., and Broderson, W. D. (1989). Soil survey of lake county. U.S. Department of Agriculture Soil Conservation Service. California: Washington, D.C. Available at: https://www.nrcs.usda.gov/Internet/FSE_MANUSCRIPTS/california/CA033/0/lake.pdf.
Sonder, I., Graettinger, A. H., and Valentine, G. A. (2015). Scaling multiblast craters: General approach and application to volcanic craters. J. Geophys. Res. Solid Earth 120, 6141–6158. doi:10.1002/2015JB012018
Stanley, W. D., and Blakely, R. J. (1995). The Geysers-Clear Lake geothermal area, California—An updated geophysical perspective of heat sources. Geothermics 24, 187–221. doi:10.1016/0375-6505(94)00048-H
Stanley, W. D., and Rodriguez, B. D. (1995). A Revised Tectonic Model for the Geysers-Clear Lake Geothermal Region, California in World Geothermal Congress Proceedings: Section 4- Exploration and Conceptual Modeling (International Geothermal Association), 6p. Available at: http://www.geothermal-energy.org/pdf/IGAstandard/WGC/1995/2-Stanley.pdf.
Stimac, J. A., Goff, F., and Wohletz, K. (2001). Thermal modeling of the Clear Lake magmatic-hydrothermal system, California, USA. Geothermics 30, 349–390. doi:10.1016/S0375-6505(00)00062-6
Swanson, D. A. (2008). Hawaiian oral tradition describes 400 years of volcanic activity at Kilauea. J. Volcanol. Geotherm. Res. 176, 427–431. doi:10.1016/j.jvolgeores.2008.01.033
Taddeucci, J., Sottili, G., Palladino, D. M., Ventura, G., and Scarlato, P. (2010). A note on maar eruption energetics: Current models and their application. Bull. Volcanol. 72, 75–83. doi:10.1007/s00445-009-0298-2
U.S. Geological Survey, and California Geological Survey (2022). Quaternary fault and fold database for the United States. Available at: https://www.usgs.gov/natural-hazards/earthquake-hazards/faults (Accessed August 1, 2020).
Valentine, G. A., Graettinger, A. H., and Sonder, I. (2014). Explosion depths for phreatomagmatic eruptions. Geophys. Res. Lett. 41, 3045–3051. doi:10.1002/2014GL060096
Valentine, G. A., Shufelt, N. L., and Hintz, A. R. L. (2011). Models of maar volcanoes, Lunar Crater (Nevada, USA). Bull. Volcanol. 73, 753–765. doi:10.1007/s00445-011-0451-6
Valentine, G. A., Sottili, G., Palladino, D. M., and Taddeucci, J. (2015). Tephra ring interpretation in light of evolving maar–diatreme concepts: Stracciacappa maar (central Italy). J. Volcanol. Geotherm. Res. 308, 19–29. doi:10.1016/J.JVOLGEORES.2015.10.010
Valentine, G. A., White, J. D. L., Ross, P.-S., Graettinger, A. H., and Sonder, I. (2017). Updates to Concepts on Phreatomagmatic Maar-Diatremes and Their Pyroclastic Deposits. Front. Earth Sci. (Lausanne). 5, 68. doi:10.3389/feart.2017.00068
Verolino, A., White, J. D. L., Dürig, T., and Cappuccio, F. (2019). Black Point – Pyroclasts of a Surtseyan eruption show no change during edifice growth to the surface from 100 m water depth. J. Volcanol. Geotherm. Res. 384, 85–102. doi:10.1016/j.jvolgeores.2019.07.013
Walker, G. P. L. (1984). Characteristics of dune-bedded pyroclastic surge bedsets. J. Volcanol. Geotherm. Res. 20, 281–296. doi:10.1016/0377-0273(84)90044-1
Walker, G. P. L. (1971). Grain-Size Characteristics of Pyroclastic Deposits. J. Geol. 79, 696–714. doi:10.1086/627699
White, D. E., and Roberson, E. E. (1962). “Sulphur Bank, California, a major hot-spring quicksilver deposit,” in Petrologic studies: A volume in honor of A.F. Buddington (New York: Geological Society of America), 397–428.
White, G. G., and Fredrickson, David A. (2002). Cultural diversity and culture change in prehistoric Clear Lake basin: Final report of the anderson Flat Project. Davis, California: Department of Anthropology, University at Davis, 580p.
White, J. D. L., and Houghton, B. F. (2006). Primary volcaniclastic rocks. Geol. 34, 677–680. doi:10.1130/G22346.1
White, J. D. L., and Ross, P.-S. (2011). Maar-diatreme volcanoes: A review. J. Volcanol. Geotherm. Res. 201, 1–29. doi:10.1016/j.jvolgeores.2011.01.010
White, J. D. L., and Valentine, G. A. (2016). Magmatic versus phreatomagmatic fragmentation: Absence of evidence is not evidence of absence. Geosphere 12, 1478–1488. doi:10.1130/GES01337.1
Wohletz, K. H. (1983). Mechanisms of hydrovolcanic pyroclast formation: Grain-size, scanning electron microscopy, and experimental studies. J. Volcanol. Geotherm. Res. 17, 31–63. doi:10.1016/0377-0273(83)90061-6
Keywords: maar, stratigraphy, Clear Lake Volcanic Field, California, GSD, radiocarbon
Citation: Ball JL (2022) Stratigraphy and eruption history of maars in the Clear Lake Volcanic Field, California. Front. Earth Sci. 10:911129. doi: 10.3389/feart.2022.911129
Received: 02 April 2022; Accepted: 26 July 2022;
Published: 02 September 2022.
Edited by:
Alison Hollomon Graettinger, University of Missouri–Kansas City, United StatesReviewed by:
Dario Pedrazzi, Institute of Earth Sciences Jaume Almera (CSIC), SpainCopyright © 2022 Ball. This is an open-access article distributed under the terms of the Creative Commons Attribution License (CC BY). The use, distribution or reproduction in other forums is permitted, provided the original author(s) and the copyright owner(s) are credited and that the original publication in this journal is cited, in accordance with accepted academic practice. No use, distribution or reproduction is permitted which does not comply with these terms.
*Correspondence: Jessica L. Ball, amxiYWxsQHVzZ3MuZ292
Disclaimer: All claims expressed in this article are solely those of the authors and do not necessarily represent those of their affiliated organizations, or those of the publisher, the editors and the reviewers. Any product that may be evaluated in this article or claim that may be made by its manufacturer is not guaranteed or endorsed by the publisher.
Research integrity at Frontiers
Learn more about the work of our research integrity team to safeguard the quality of each article we publish.