- 1Department of Geology, University of Vienna, Vienna, Austria
- 2Department of Geography and Earth Sciences, Aberystwyth University, Aberystwyth, United Kingdom
- 3Department of Geology, PPM Research Group, University of Johannesburg, Johannesburg, South Africa
The Makganyene Formation is a Siderian (2.45–2.22 Ga) diamictite-dominated succession, with both outcrop and subcrop in the Griqualand West Basin of the Transvaal Group of South Africa. We provide new outcrop and core descriptions from this succession, supplemented by microscopic analyses, to present an updated depositional model for a classic Palaeoproterozoic diamictite. Although internal correlation of core and outcrop successions is not possible, a recurring pattern is observed where diamictites are organised into coarsening-upward motifs at the tens of metres scale. With additional finds of striated clasts, and evidence for dropstones both at the core scale and at the microscopic scale, earlier interpretations of glacial control on sedimentation can be substantiated, with modification of glacial diamictites by mass flow processes also recognised. Overall, given the characteristic progradational stratigraphic architecture, we propose a new model for the Makganyene Formation which is considered to represent deposition of a grounding zone wedge at an ancient, oscillating ice margin.
Introduction
The origins of poorly sorted sedimentary rocks (diamictites) have a special significance in studies of the geological record, because 1) they may indicate the presence of hinterland ice, 2) if glacial, they can reflect a wide variety of settings (Eyles and Lazorek, 2014) and 3) if these settings can be brought into focus, they have the potential to reveal the behaviour of the cryosphere in deep time. It is, however, well established that diamictites do not always indicate the presence of ice in the rock record, where several studies have shown a clear genetic link to solely tectonic processes, such as crushing of material during orogenesis (e.g., Wendorff, 2000), or catastrophic olistostrome emplacement (Nascimento et al., 2016). Even diamictites known to have been deposited during a major ice age may paradoxically contain little to no evidence for direct glacial processes. The emplacement process may simply record mass flow deposition, as is particularly well documented in the Cryogenian (e.g., the SE Congo Basin: Kennedy et al., 2019) and Late Palaeozoic ice ages (Botswana: Dietrich et al., 2019). Thus, a vital first step is establishing the strength of evidence for a glacial influence, especially in hybrid situations where glacial and non-glacial diamictites are intimately interbedded with one another (e.g., Le Heron et al., 2017). Where a glacial origin is demonstrable, unravelling the specific glacial process and glaciological context to thereby shed light on cryosphere dynamics, is the higher level objective and aspiration.
For the deep time glacial record, including strata deposited during the Siderian period of the Palaeoproterozoic, it is clear that comparison to better preserved, younger records is critical. Rocks of this period expose some excellent diamictites, including formations such as the Gowganda in Ontario, Canada (Eyles, 1988) or the Makganyene Formation of South Africa. Each of these formations was argued to have been laid down during a Palaeoproterozoic Snowball Earth event (Kirschvink et al., 2000) and, consequently the palaeoenvironmental significance of these deposits could is far reaching, potentially representing the first great ice age on Earth. In this paper, we incorporate observations from outcrop and drill core to present an updated depositional model for the Makganyene Formation as a Palaeoproterozoic grounding zone wedge (GZW). In so doing, we provide an updated perspective on the palaeoenvironmental interpretations that can be extracted from the ancient diamictite record.
Geological background
Palaeoproterozoic snowball earth
The Palaeoproterozoic contains evidence for multiple glacial episodes, some of which can be readily correlated, others less so. An early protracted snowball Earth episode (e.g., Evans et al., 1997; Hoffman, 2013) has been proposed, which contrasts with the view of multiple, diachronous glaciations suggested by others (Young, 2014). Siderian (ca. 2.45–2.22 Ga) diamictites and associated iron-rich deposits are certainly widespread (Rasmussen et al., 2013a), including in South Africa where they are represented by the Makganyene Formation. The dual occurrence of purportedly glacial diamictites and iron formations has motivated some authors to propose a Palaeoproterozoic snowball Earth (Kirschvink et al., 2000) and to try to correlate the deposits across wide areas (Hoffman, 2013). Kopp et al. (2005) went so far as to broadcast the event as “a climate disaster” driven by the rapid development of photosynthesis. The Makganyene Formation of South Africa was one of the inspirations for this idea and is widely assumed to be glacial in origin (Kirschvink et al., 2000). It was deposited on the Kaapvaal Craton, which formed part of the Superia Supercraton during the Palaeoproterozoic and which occupied low palaeolatitudes (Figure 1A; Gumsley et al., 2017).
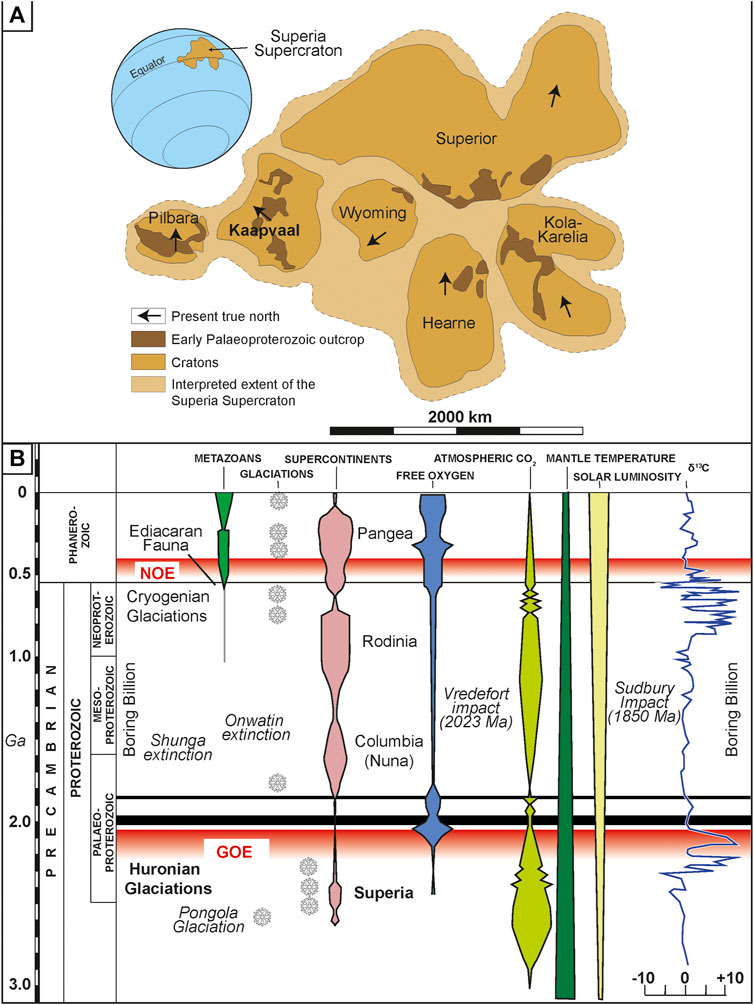
FIGURE 1. (A): Palaeoproterozoic craton configuration and palaeogeography, showing the location of the Kaapvaal Craton within the proposed Supercraton of Superia. Redrawn and simplified after Gumsley et al. (2017). (B): Timeline of events throughout the Proterozoic, including the Huronian glaciations of the Siderian (Palaeoproterozoic). Redrawn and slightly modified from Young (2013).
The geochronology of Palaeoproterozoic glaciations remains poorly constrained. Three to four widespread glaciations are interpreted between 2.45 and 2.22 Ga (Zakharov et al., 2017). Thus, these glaciations may reflect a similar situation to Cryogenian events, where a number of diachronous glaciations can be recognised (Spence et al., 2016; Le Heron et al., 2020), in contrast to the globally synchronous Snowball Earth events originally hypothesised (e.g., Hoffman et al., 2011). Currently, the Makganyene Formation is assigned to the Huronian Glacioera (Chumakov, 2015) (Figure 1B). This glacioera preceded the so-called Great Oxygenation Event, followed on from a phase of high atmospheric CO2 levels and significant δ13C carbon isotope excursions (Figure 1B; Young, 2013). Recent sedimentological studies of Siderian diamictites focused on core datasets are rare. Notable exceptions include the work of Araújo and Nogueira (2019) from the Amazonian craton, with high quality core data permitting a reconstruction of subglacial and proglacial environments. Through careful facies analysis, those workers recognised a coastal diamictite (on the basis of a quartz and magnetite-rich matrix) and a marine diamictite (with a mud-rich matrix). That study illustrated the potential of pristine, unweathered cores for sedimentological analysis, and thus serves as inspiration for the present paper.
Stratigraphic context and previous work on the Makganyene Formation
The Makganyene Formation is a Siderian unit that crops out in the areas around Griquatown in the Northern Cape Province, South Africa (Figure 2). Rogers (1906) first discovered diamictites that became known as the Makganyene Diamictite Formation in the Griqualand West Basin and correlated these with the Rietfontein Diamictite Member of the Transvaal Basin, South Africa. Visser (1971) argued strongly for a glacial mode of origin, citing striated clasts and lonestones in finer-grained strata that were interpreted as dropstones. Based on the analysis of core material west and north of Postmasburg, De Villers and Visser (1977, p. 7) developed these ideas and used thickness increases to the south to propose that “glaciation started on land to the far north.” Underpinning this, excellent lithological descriptions were made, albeit with relatively little information on grain size variations and diamictite texture. In a summary paper of the key characteristics of these rocks, Visser (1981) lamented the outcrop quality and relied chiefly on borehole data from two cores, ML2 and KP1, to describe the succession. Visser (1981, p.183) interpreted the Makganyene Formation to have been deposited via laminar and turbulent remobilization of glaciodeltaic sediments. The glacial origin was reinforced by Moore et al. (2001) who argued for a long-lived (100 Ma) ice age over which the Makganyene was deposited. Despite strong depositional evidence for a glacial origin, it is worth noting that noglacial geomorphological features (e.g., striated pavements, 3D glacial landforms) have been reported to date from either beneath or within the Makganyene Formation.
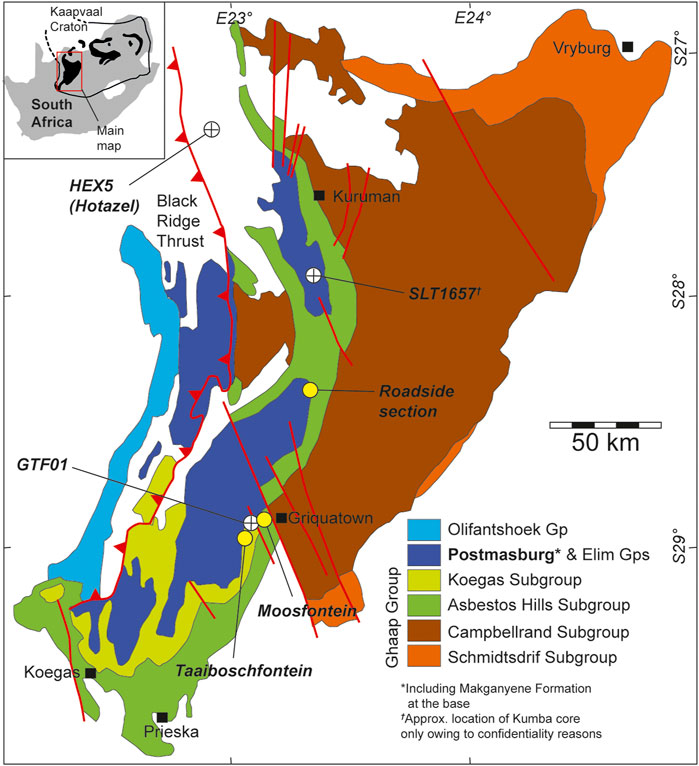
FIGURE 2. Geological sketch map (redrawn after Schröder et al., 2011) including the location of outcrops visited and boreholes examined in this study.
Debate has surrounded the bounding relationships between diamictites and underlying strata. The Postmasburg Group (with the Makganyene Formation at the base) was considered to rest unconformably upon the Ghaap Group (Beukes, 1983; Beukes and Smit, 1987) (Figure 2). The Ghaap Group consists of dolomitic carbonates that are capped by banded iron formations of the Asbestos Hills Subgroup and mixed marine clastic and chemical sedimentary rocks of the Koegas Subgroup (Lantink et al., 2018 and refs therein). Based on mapping, Polteau et al. (2006) argued that the unconformity between the Ghaap and Postmasburg groups was only locally developed at basin margins and conformable contacts were more typical. Schröder et al. (2011) emphasised the complexities in correlating between the Griqualand West and Transvaal basins. This was specifically owing to the issue of the Griqualand West basin having a considerably more condensed section than the Transvaal Basin, and hence the requirement to interpret a number of “converging unconformities” between the two basins recording Palaeoproterozoic rifting (Schroeder et al., 2016; their Figure 2). In terms of geotectonic setting, Bekker et al. (2020) interpreted that the Koegas Subgroup sat within the foredeep of a foreland basin system, with the orogen to the SW and the backbulge/craton interior to the NE. An important stratigraphic observation in this regard is that the Makganyene Formation locally interfingers with and is covered by Ongeluk Formation flood basalts (Lantink et al., 2018), raising questions about the depositional context of the diamictites and whether they are really of glaciogenic origin. Schröder et al. (2011) provided a detailed stratigraphic and sedimentological analysis of formations of the Ghaap Group, the succession lying immediately underneath the Makganyene. This analysis was focussed on the GTF01 borehole, to which we refer herein (Figure 2), together with analysis of nearby outcrops, allowing palaeocurrent information for shallow marine sandstones to be integrated. That work showed a spread of foreset and current ripple azimuths, allowing a broad SW to NW palaeocurrent dispersal to be interpreted (Schröder et al., 2011), broadly compatible with the geotectonic configuration envisaged later by Bekker et al. (2020).
Gumsley et al. (2017) proposed a maximum age for the Makganyene Formation from the Griqualand West sub-basin from sandstone intervals of 2,436 ± 14 Ma. Building on previous correlations that “expanded” the Makganyene stratigraphy of the Griqualand West Basin into three separate diamictite horizons separated by shales, sandstone and carbonates in the Transvaal Basin, Li et al. (2020) proposed a new maximum depositional age of 2,424 ± 12 Ma for the Duitschland Formation and 2,324 ± 17 Ma in Timeball Hill Formation of the latter basin. Both of these units are considered to lie considerably above the equivalent to the Makganyene Formation, conflicting with the maximum age proposed by Gumsley et al. (2017). More recently, however, the correlations have been called into question by Bekker et al. (2020). These authors viewed the Makganyene as older than all of the diamictites in the Transvaal Basin. The precise age of the Makganyene Formation and its correlation to neighbouring basins and beyond thus remains contested and contentious.
Materials and methods
In addition to fieldwork, which focussed on sedimentary logging, material from three shallow drill cores were studied, namely HEX 5, SLT1657, and GTF 01 (Figure 2). The former two cores were obtained in the course of iron and manganese ore prospecting and made available to the authors, whereas GTF 01 was obtained during the Agouron-Griqualand Paleoproterozoic Drilling Project (AGPDP) in 2004. The GTF 01 core is stored at the Council for Geoscience (South Africa) in Pretoria. The core was drilled at Taaiboschfontein farm (Coordinates: S 28° 49′ 39.7″; E 023° 07′ 24.1″; Surface elevation: 1,306 m) and has a total length of 328.6 m. In the laboratory, core samples were cut for thin section analysis, and the thin sections were captured using a flat-bed scanner for micromorphological interpretation.
Results
Overview: Sedimentary logs
In the following, we consider both outcrop and borehole material together. Sedimentary logs were produced from three cores (HEX 5, GTF 01 and SLT1657) and two outcrops (Figure 3). We recognise 1) a predominantly clay-matrix diamictite facies, 2) a predominantly silt-matrix facies, 3) sandstone and pebbly sandstone facies, and finally 4) an iron-rich mudstone facies. On several of our measured sections, iron formation is found at the base and comprises a dense, crystalline lithology locally ascribed to individual iron formations (e.g., the Rooinekke Iron Formation of the Koegas Subgroup at Taaiboschfontein; Figure 3). However, in the SLT1657 core, iron formation is found sandwiched between diamictite (Figure 3). Vertical transitions between facies are complex. Coarsening up motifs are recognised from the clay-matrix diamictite facies to the silt-matrix diamictites (e.g., HEX 5 Core, Figure 3). These transitions occur at both the m-scale and at the tens of metres scale. The diamictites are interrupted by 1–10 m thick accumulations of a sandstone to pebbly sandstone facies (c.f. HEX 5 Core, GTF 01 Core, Moosfontein outcrop: Figure 3). This latter facies locally expresses highly complex grain-size changes: granules and pebbles cluster at intervals, whereas the matrix shows coarsening up at the base and fining up at the top (HEX 5 Core, 8–18 m, Figure 3). Other vertical variations include alternating clast-rich and clast-poor zones of diamictite (GTF Core, Figure 3) and vertical trends from massive to stratified diamictite (Taaiboschfontein section, Figure 3).
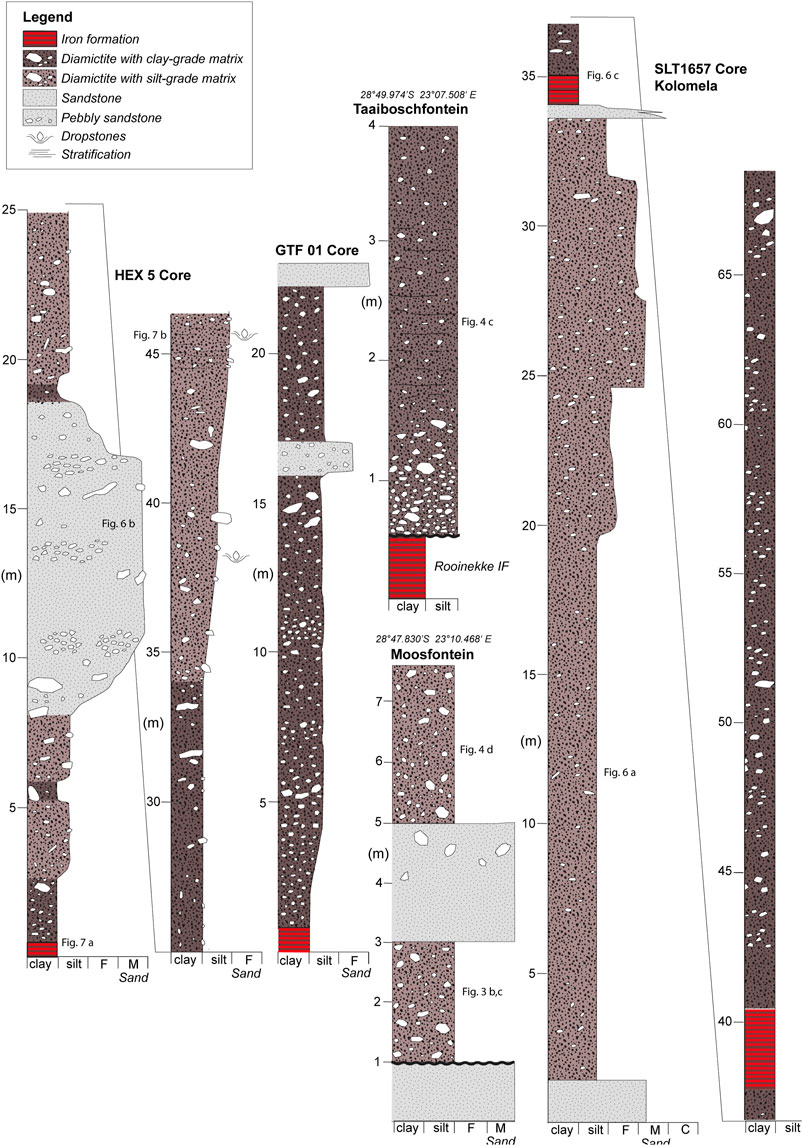
FIGURE 3. Sedimentary logs of the Makganyene Formation, drawn from both shallow subsurface boreholes (HEX 5, GTF 01 and SLT1657) and from outcrop (Taaiboschfontein and Moosfontein) outcrops. Further outcrop observations made at a roadside section Postmasburg and Kimberley (see Figure 1 for location) are also included in this paper, but the section only exposed 3 m of stratigraphy and was not logged.
Descriptions
Outcrops of the Makganyene Formation were studied at a roadside section near Postmasburg and Kuruman and at Taaiboschfontein and Moosfontein farms (Figure 2). Exposures are partial and incomplete. The roadside section exposes neither lower nor upper contacts. At Taaiboschfontein, an irregular basal contact with the Rooinekke Iron Formation is observed, but the top contact is not exposed. Likewise, at Moosfontein, a well-expressed basal contact can be seen, whereby the Makganyene Formation sits in angular unconformity on sandstone of the Pannetjie Formation (Koegas Subgroup). The basal contact expresses an elevation difference of >100 m between the Taaiboschfontein and Moosfontein outcrops.
Aerial photography over Moosfontein illustrates the subhorizontal aspect of the present-day outcrop and allows the Makganyene lower boundary to be summarised as sub-planar over a scale of about 100 m (Figure 4A). At the local scale, the strike and dip of the Rooinekke and overlying Makganyene Formations are parallel to one another. However it should be noted that the contact with the Pannetjie Formation is not conformable, since the contact implies several missing formations including the Rooinekke Iron Formation (Bekker et al., 2020). At this outcrop, diamictites contain a range of both rounded and angular clasts (Figure 4B). At ground level the base of the diamictites cannot be determined owing to scree cover (Figure 4C). Clasts consist predominantly of chert and sandstone with subordinate carbonate fragments, do not exceed cobble size, and range from very angular to sub-angular. Locally, pebble “core-stones” are surrounded by granule-sized clasts. Examination of the surfaces of sandstone clasts at the Postmasburg-Kimberley roadside section reveals well preserved cross-cutting striations on the surface (Figure 5A). The preservation of these structures is remarkable at this outcrop, owing to evidence for intense surface weathering. Broken blocks at the roadside show a dm-thick alteration rind that penetrates the massive diamictite (Figure 5B), taking the form of Liesegang laminations which obscure primary stratification. Appraisal of other outcrops, however (Figures 5C,D), reveals that stratification is well developed, and extends for tens of metres at the outcrop-scale. Both below and above the stratified diamictite intervals, massive diamictites occur (Figure 5D). A m-scale alternation of clay-rich and silt-rich matrix diamictites also occurs, allowing the stratification to be clearly seen.
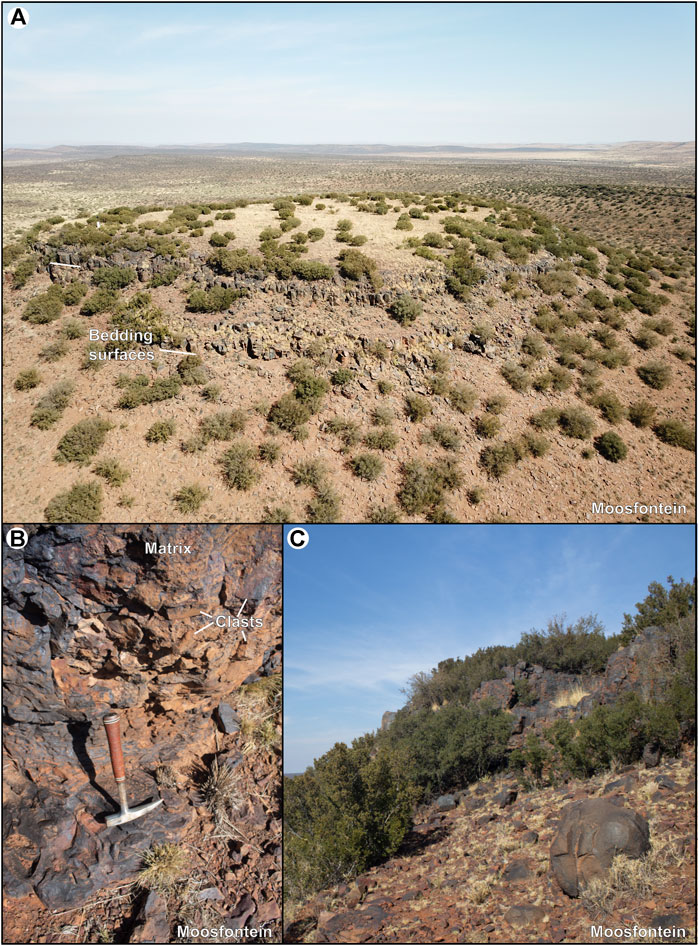
FIGURE 4. Outcrop character and facies at Moosfontein Farm. (A) Aerial photograph taken with a DJI Mavic Pro UAV, showing the regionally flat character of the stratigraphy in the Makganyene Formation. (B) Typical exposure of ferruginous diamictite, containing a range of angular to rounded clasts. (C) Typical weathering character.
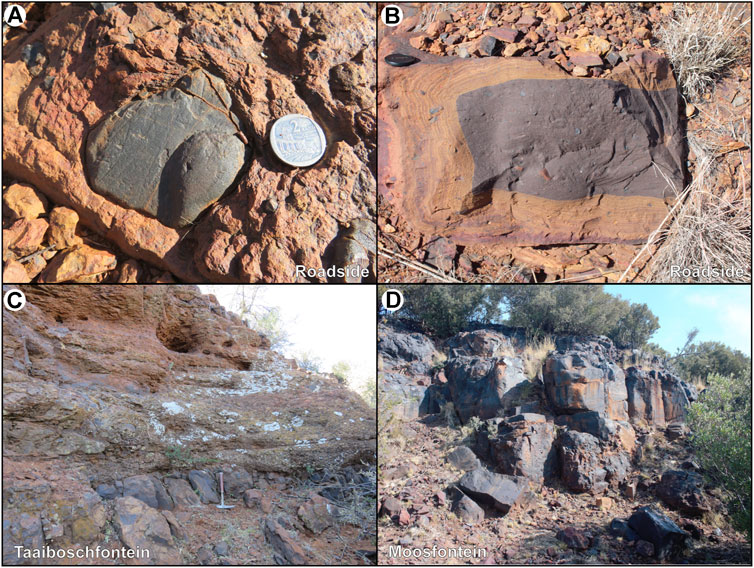
FIGURE 5. (A) Facetted and striated clast embedded in ferruginous diamictite. (B) Typical yellow-red weathering rind of ferruginous diamictite, expressed as concentric Liesegang laminae. Toward the centre of the broken block, unaltered brown diamictite occurs. (C) Alternating massive (base of exposure) and stratified (above hammer) variations of diamictite at Taaiboschfontein farm. Note subhorizontal to gently dipping beds. (D) Massive ferruginous diamictite at Moosfontein farm. Also note gently dipping beds.
In contrast to the outcrops, as noted by Visser (1981), the quality of Makganyene drill core material is generally high, with excellent recovery and continuity for tens of metres in some boreholes such as HEX 5 (Figure 6). In that example, the basal contact between the Makganyene and the Rooinekke Iron Formation is sharp, and the comparatively unweathered core material reveals distinct colour differences that allow iron-rich mudstones, clay-matrix diamictites, silt-matrix diamictites, and pebbly sandstones to be recognised (Figure 6). In fresh hand specimen (from core), clasts mostly consist of sandstone, with subordinate chert and jasper derived from the underlying iron formations (Figure 7A). In the pebbly sandstone intervals, diffuse parallel stratification is observed which is defined by subtle variations in grain size within granule-conglomerate to coarse-grained sandstone intervals (Figure 7B). The full range of clast roundness is recognised in many cores, and clast-poor muddy diamictites are observed to show gradual vertical transitions into wispy, stratified facies (Figure 7C). In a number of examples, lonestones are associated with impact structures at the core-scale (e.g., HEX 5, 38 and 46 m) (Figure 3).
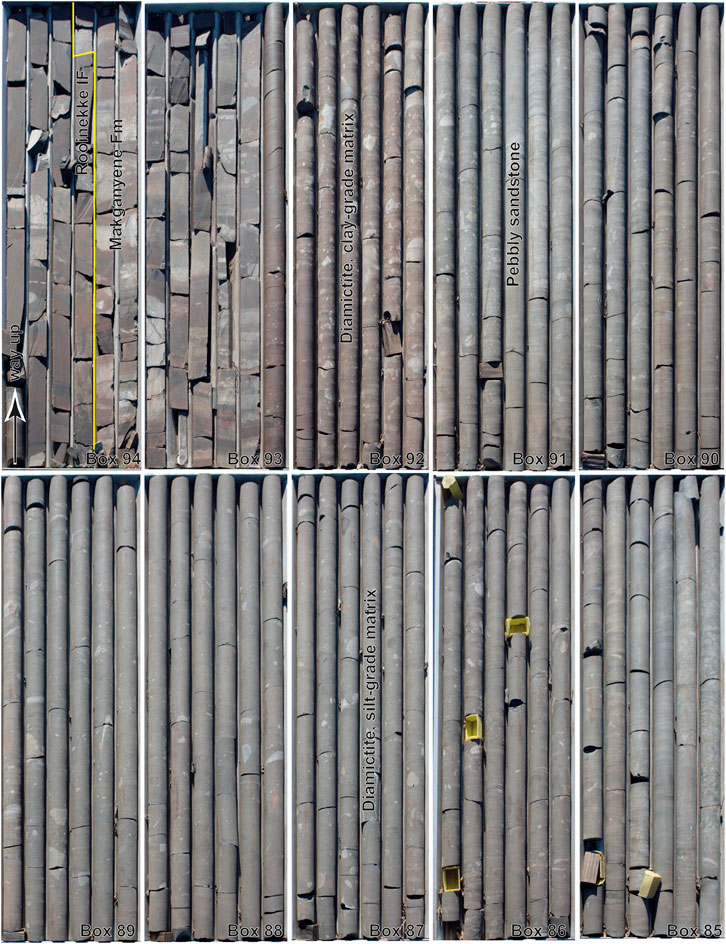
FIGURE 6. Example interval from the HEX 5 core, showing how clearly the facies can be distinguished in contrast to the deeply weathered outcrops.
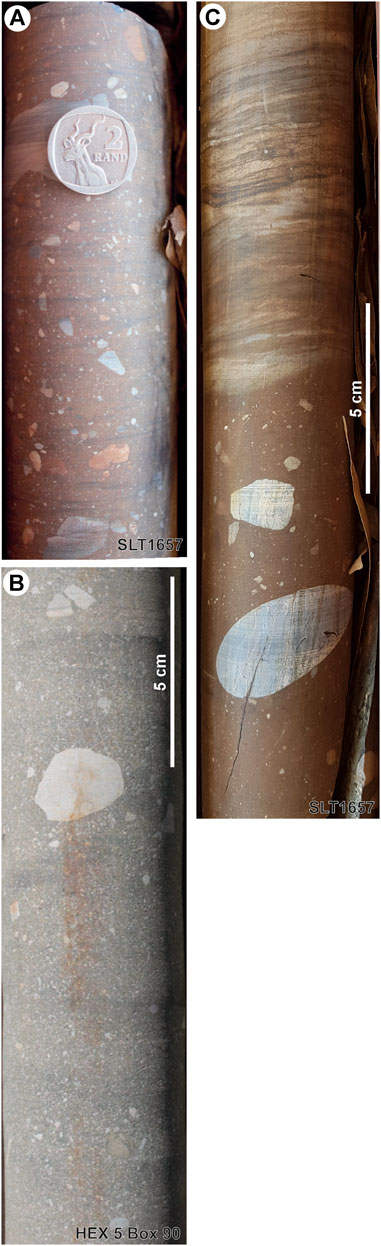
FIGURE 7. Detail of facies from core photographs. (A) Massive, ferruginous diamictite with clay-rich matrix from the SLT1657 core. (B) Pebbly sandstone with diffuse, subparallel stratification, from the HEX 5 core. (C) Contact between massive, clast-poor diamictite and stratified diamictite in core SLT1657. The stratigraphic position of each of the photographed core intervals are shown on Figure 2.
In thin section, micromorphological analysis provides additional insights not possible at the hand specimen to outcrop scale. For instance, in the HEX 5 borehole, the contact between a basal iron formation and the Makganyene Formation (Figure 2) is clearly expressed as an angular contact at the mm-scale, where truncation of the normally graded underlying laminae can be demonstrated (Figure 8A). Dipping laminae downlap or drape onto this contact, and contain sand-sized outsized clasts with locally puncture the underlying laminae. The laminae are crosscut by cement-filled fractures. At other intervals, such as at the top of the logged HEX 5 core, lamination is wavy in character, is defined by clast-poor zones, and shows evidence for small-scale deformation and folding (Figure 8B).
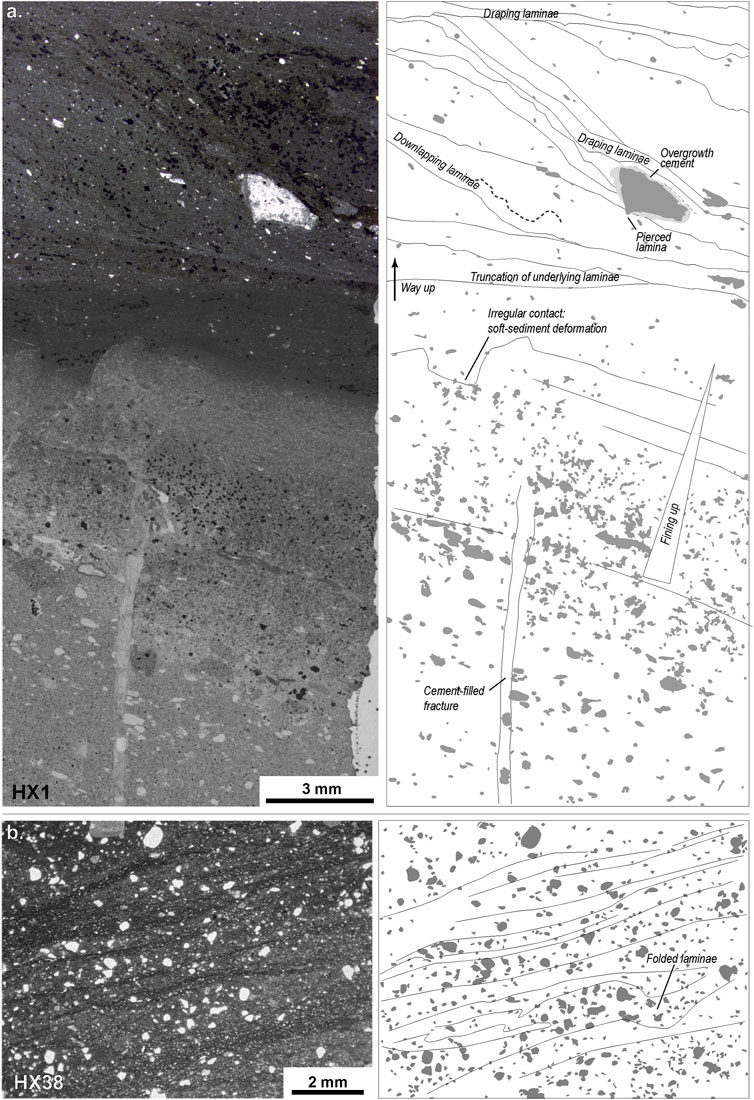
FIGURE 8. Thin section analysis/micromorphological interpretation of two intervals in the HEX 5 core (see Figure 3 for stratigraphic position of samples). (A) Contact between the basal (Rooinekke) ironstone and diamictite of the Makganyene above. Note presence of small lonestone that pierces underlying laminations. (B) Pervasively stratified deposits with evidence for small-scale folding.
Interpretation
Our interpretation builds on a rich tradition of papers that envisage a glacial origin for the Makganyene Formation (De Villiers and Visser, 1977; Visser, 1981; Polteau et al., 2006), but places it in a more tightly constrained depositional context. Aside from the bounding contacts at the base, the sedimentary logs reveal no marker horizons that may allow them to be correlated. Evidence for lonestones associated with impact structures at the core-scale (e.g., HEX 5, 38 and 46 m) supports an ice-rafted debris (dropstone) interpretation. This interpretation is reinforced by similar textures in delicately laminated diamictites at the microscopic scale (Figure 8), and could have two possible origins. The first is introduction of dropstones via floating icebergs, a model that is widely appealed to in interpretations of other ancient records (e.g., Le Heron, 2015; Le Heron et al., 2021). However, given the dominance of diamictite in the succession, in conjunction with the recognition of coarsening-upward cycles over tens of metres (Figure 3), we propose that dropstones were probably introduced beneath a floating ice shelf near the grounding zone which delivered an abundance of material by rain-out. Under this mechanism, the development of stratification at many scales is explained, together with those intervals in which stratification is defined by the repeated appearance of coarse sand layers within the diamictite (e.g., 18–30 m, SLT1657 core, Figure 3). This is because debris in the basal ice would have been distributed heterogeneously, promoting the development of stratification upon deposition. In addition to those described by us (Figure 5A), striated clasts are well known and documented from other outcrops west of Griquatown (e.g., De Villiers and Visser, 1977; Melezhik et al., 2013, their Figure 7.23), adding further weight to the evidence for glacial abrasion on clast surfaces (Visser, 1981). The available evidence, both from our observations and those of previous authors, does not permit us to determine whether clasts represent in situ subglacially striated clasts, or alternatively the remobilisation and reworking of primary subglacial material by mass flows.
The thick pebbly sandstones in core HEX 5 (Figure 3) are interpreted as the deposits of a waxing then waning flow, or alternatively to a thick turbidite bed deposited upon a high density traction carpet (Henstra et al., 2016). Likewise, in the SLT1657 core (Figure 3), the isolated well expressed normally-graded sandstone in the middle part of the succession is interpreted to record low density turbidite deposition (Lowe, 1982; Mulder and Alexander, 2001). These interpretations reinforce the general picture painted by Visser (1981). Nevertheless, in contrast to other core studies of similar diamictite intervals elsewhere (Kennedy et al., 2019), we suggest that a glacial source for the material is most likely. We specifically reference the abundant striated clasts reported not only by us but by previous studies (De Villiers and Visser, 1977; Melezhik et al., 2013) and the dropstone textures in both core and thin section. Moreover, as outlined below, we contend that the stratigraphic architecture, including the grain size organisation of the diamictites, can be explained via a direct glacial depositional mechanism.
Thick diamictite accumulations are recognised in a number of glacial contexts (Eyles and Lazorek, 2014), which must be considered before proposing a depositional model. Potential settings include, broadly from proximal to distal: 1) a moraine, 2) an ice contact delta, 3) a till sheet, 4) a trough mouth fan, and 5) a grounding zone wedge. The moraine interpretation is discounted owing to the limited evidence of meltwater reworking or channelization characteristic of their typically more temperate or polythermal setting (Powell & Alley, 1997; Batchelor & Dowdeswell, 2015), whilst the absence of evidence for push-squeeze processes, though not a prerequisite, may lend further support to this interpretation. Furthermore, large moraine ridges are typically associated with slower-flowing, inter-ice stream areas where rates of sediment supply are reduced compared to high flux ice streams (Ottesen & Dowdeswell, 2009; Batchelor & Dowdeswell, 2015), and thus less likely to be characterized by a progradational stratigraphic architecture. Ice contact deltas, which are commonly progradational, are characterized by sandy deposits with large concentrations of well-sorted, supercritical flow deposits (Winsemann et al., 2007) and hence do not reflect the lithologies of the Makganyene Formation. Although a till sheet deposited under a floating ice shelf could explain multiple layers of diamictite intercalated with dropstone intervals (Domack et al., 1999), this model does not reconcile with repeated coarsening upward profiles over tens of metres. In terms of deep marine possibilities, interpretation as a trough mouth fan seaward of a major ice stream (Dowdeswell et al., 2019) suffers from the paucity of anticipated well developed turbidites (Le Heron et al., 2019) or hemipelagic deposits that would be expected between pulses of sediment delivery (Escutia et al., 2000). Thus, the majority of potential settings for thick diamictite accumulation fail to adequately explain all of the observed phenomena in the Makganyene Formation.
Instead, we propose that the Makganyene Formation represents a grounding zone wedge complex, constructed of interbedded 1) primary or reworked subglacial sediments, 2) the deposits of ice rafting and 3) sediments remobilised by both traction-currents and debris flows. At the scale of the entire formation, organisation of the diamictites into punctuated, large scale coarsening upward units over tens of metres (e.g., HEX 5 core, SLT1657 core) is fully consistent with the development of a grounding zone wedge (e.g., Dietrich and Hoffman, 2019), simply explained as a glacial advance motif in glacial sequence stratigraphy (Busfield and Le Heron, 2018). The punctuated nature of the coarsening-upward profiles is taken to imply complex multiphase development of the GZW. This may either imply oscillating thermal regimes of the interpreted ice mass (Ives, 2021). In this model, the punctuated coarsening-upward character is suggested to record subtle grounding zone fluctuations from a relatively more distal to a relatively more proximal position, with an increasingly large volume of sediment delivered to the sea floor via a combination of direct delivery of subglacial till, local resedimentation by sediment gravity flows, and rain-out of ice-rafted debris (IRD).
Note that although this is the first such interpretation of a GZW in the Palaeoproterozoic glacial record, and thus the oldest example of such a deposit, there are two strong precedents from other geological records of ancient glacial deposits. First, Domack and Hoffman (2011) recognised such phenomena in the pulsed progradational signature (coarsening upward profile) of the Ghaub Formation (late Cryogenian of northern Namibia), built up of massive and stratified diamictites bearing striated clasts and dropstones. Second, Dietrich and Hoffmann (2019) interpreted a GZW from the Dwyka Group (Late Palaeozoic Ice Age) of the eastern Karoo Basin, South Africa in which a similar pulsed progradation was recognised. The latter paper is particularly important as the deposits are very similar to the Makganyene Formation, consisting of massive to stratified diamictites with striated clasts together with dropstone-bearing laminites and sandstones as interbeds. From the perspective of the Dwyka, Dietrich and Hoffmann (2019) developed a theoretical framework to recognise GZWs in the rock record, cognizant of the disparate approaches taken to Quaternary (better geophysical data, lateral control) and deep time (better stratigraphic record) glaciomarine sequences. These authors recognized that although GZWs imply a particular process - line source delivery of subglacial material that is reworked by gravity flows (after Bell et al., 2016)—the lack of criteria was problematic to recognize ancient examples, and they thus proposed a four-part solution incorporating geomorphological, architectural, sedimentological and biological indicators to aid GZW recognition depending on the available datasets (Dietrich and Hoffmann, 2019). The Makganyene Formation satisfies most of the criteria proposed by the latter authors in terms of architectural and sedimentological indicators for GZW recognition (stacking pattern, laminiation and bedding, full range of grain sizes: see their Figure 12) with the geomorphological indicators unavailable (outcrop quality being limited) and the biological indicators being irrelevant to rocks of Palaeoproterozoic age (foraminiferal, diatom assemblages, distribution of bioturbation).
The iron-rich nature of diamictites in the Makganyene Formation can be largely explained by reworking of the underlying iron formations (Polteau et al., 2006). However, the occurrence of banded iron formation, which are devoid of clasts in the SLT1657 core (34–35 m, Figure 3) requires an alternative explanation. In similar Cryogenian strata, Lechte and Wallace. (2016) proposed that iron-rich interbeds within diamictites were deposited as a result of the efflux of oxygenating subglacial brine pools. In that model, efflux of this water occurred at the grounding line, beyond which ice rafted debris was delivered to the basin floor via a floating ice shelf or icebergs. This work was based on comparative analysis of iron-rich Cryogenian deposits of the Holowilena Ironstone (South Australia), the Chuos Formation (Namibia) and the Kingston Peak Formation (United States). Therefore, by analogy, it is possible that some of the iron-rich intervals in the Makganyene may have been deposited in a similar way, and thus at least some of the iron content may be attributable to precipitation concomitant with diamictite deposition, rather than exclusively reworking from underlying iron formations (c.f. Polteau et al., 2006). However, given the described intercalation of the Makganyene Formation diamictites and basalts by other authors it is also possible that the iron formations were generated through a hydrothermal process, which has been inferred for the underlying Transvaal Supergroup iron formations (Beukes and Gutzmer, 2008; Smith, 2018). Differentiating these two processes is required, but note that both mechanisms require precipitation in a standing body of water. Therefore, it should be noted that the interpreted context of a grounding zone wedge at the ice margin is compatible with either model.
Discussion
Makganyene grounding zone wedges and palaeo-ice sheet reconstruction
At modern high-latitude continental margins, a variety of glaciological, topographical, and temporal factors influence the location and morphology of grounding zone wedges, enabling us to make some important inferences on the nature and evolution of the Palaeoproterozoic ice-marginal landsystem in this region. GZWs form during episodic ice mass retreat, where still-stands or minor re-advances in the grounding zone position enable large volumes of diamictic sediment to accumulate (Batchelor & Dowdeswell, 2015). They are characterised by a progradational stratigraphic architecture, with active progradation in the direction of ice flow (Demet et al., 2019). Distinct coarsening- and shallowing-upward sequences can therefore be correlated with higher order fluctuations in the position of the grounding zone (Domack and Hoffman, 2011; Dietrich and Hoffmann, 2019). Our logged sections do not permit internal correlation, precluding our ability to assess whether coarsening-upward sequences at each site are time-equivalent or not, and the absence of recorded top or bottom contacts prevent the full sequence of ice-margin oscillations from being quantified at this time. Nonetheless, even focussing on the HEX 5 core alone at least four distinct progradational stacking patterns can be recognised, which we interpret to reflect a minimum of four ice-margin fluctuations during overall retreat. The predominance of diamictite throughout, and absence of deposits devoid of a glacial influence between these sequences (e.g., hemipelagite or IRD-free sediment gravity flow deposits) indicates that intervening retreat phases of each oscillation may have been of limited lateral extent and/or short duration (e.g., Dietrich and Hoffmann, 2019). These observations are consistent with a grounding zone-proximal position throughout deposition of the Makganyene Formation. Note, however, that it is possible that owing to the potential for erosion during readvance, interglacial deposits associated with ice-minima events may have been eroded.
GZWs have been identified as sedimentary proxies for the presence of former ice shelves (Batchelor and Dowdeswell, 2015; Dowdeswell et al., 2016). It is worth noting that in a comprehensive study of 6,275 grounding line landforms, Simkins et al. (2018) found no strong support for the presence or absence of an ice shelf in determining exclusively whether grounding zone wedges or recessional moraines accumulated in these settings. However, whilst GZWs can form in the absence of an ice shelf (Powell and Alley, 1997; Bart et al., 2018), their sedimentary character can be quite different at tidewater termini due to the availability of meltwater. Greater meltwater reworking permits the development of more heterogeneous ice-contact fans or mixed influenced GZW-fan systems (e.g., Demet et al., 2019; Dietrich and Hofmann, 2019). The predominance of poorly sorted, highly concentrated glaciomarine deposits in the Makganyene Formation outcrops studied herein, the limited evidence for meltwater reworking (e.g., no unidirectional cross-stratification, rare and isolated examples of turbulent sorting), and the oxygenating subglacial brine model of iron formation all point to deposition from a relatively cold glacier, which would be consistent with an ice shelf setting.
GZWs are also considered to be diagnostic of the former presence of ice streams (Batchelor and Dowdeswell, 2015), which are arterial, fast flowing corridors of ice surrounded by slower moving ice (Bamber et al., 2000). They may record rapid grounding zone sedimentation, where fast ice flow is necessary to sustain the high and fairly continuous supply of sediment to these landforms (Dowdeswell et al., 2016). In support, several GZWs are found in close association with or often overprinted by streamlined bedforms (e.g., mega-scale glacial lineations or other subglacial features: Livingstone et al., 2012) indicative of ice-streaming flow (Ó Cofaigh et al., 2005; Ottesen et al., 2008; Graham et al., 2010). This genetic association is of particular significance as, to the authors’ knowledge, palaeo-ice streams have yet to be documented from a Palaeoproterozoic glaciation.
In South Africa, Visser (1971) envisaged that the Griqualand West basin was separated to the north from the Transvaal Basin by a palaeohigh that was named the Vryberg Ridge. Hence, ice masses and thus sediments could be proposed to have flowed to the south (in the case of our study area) and to the northeast (in the case of the Transvaal Basin) respectively. In terms of palaeogeography, by integrating the palaeoflow data from underlying strata (Schröder et al., 2011) and the geotectonic model of Bekker et al. (2020) as a starting point, it is proposed that the ice stream sourcing the Makganyene Formation GZW was initiated in the east on a cratonic platform and flowed westward toward a deep marine basin. Given the active geotectonic context within a retroarc foreland basin system (Bekker et al., 2020), it is premature to attempt to assign a specific component of downcutting beneath the Makganyene Formation to subglacial erosion, and to identify subglacial erosional features. However, the absence of streamlined geomorphic indicators that are so commonly recorded from Phanerozoic palaeo-ice streams (e.g., Assine et al., 2018) is notable, in spite of appropriate outcrop conditions (flat lying strata, paucity of vegetation). Significant advances have been made in the identification, quantification, and 3D reconstruction of former subglacial beds through the use of Uncrewed Aerial Vehicle surveys, photogrammetry, and LiDAR technologies (Thorndycraft et al., 2016; Dietrich et al., 2021; Le Heron et al., 2022), and thus re-examination of the wider region to locate the missing Makganyene ice stream(s) is clearly timely. However, we contend that the identification of GZW diamictic sequences in the geological record offers valuable and underutilised cryptic evidence of palaeo-ice stream activity, particularly in ancient or heavily vegetated terrains where well-exposed 3D bedforms might be harder to locate.
Implications for a palaeoproterozoic “snowball earth”
Palaeomagnetic data from the Ongeluk lavas, emplaced coevally and closely following deposition of the Makganyene Formation, indicate a depositional palaeolatitude of 11°C ± 5°C (Evans et al., 1997), where evidence for glacial conditions at such low, tropical palaeolatitudes has been used to argue in favour of a Palaeoproterozoic “snowball Earth” (Kirschvink et al., 2000). Our observations remain consistent with a glacial origin for the low-latitude Makganyene Formation (sensu De Villiers and Visser, 1977; Visser, 1981; Polteau et al., 2006), and our identification of a Palaeoproterozoic ice margin is an important piece of the puzzle when it comes to reconstructing palaeo-ice sheet position and configuration. However, grounding zone landforms do not enable us to infer the extent of any palaeo-ice shelf beyond the grounding zone (Dowdeswell et al., 2016), limiting our understanding of even regional let alone global ice cover. In the absence of reliable correlation pinpointing synchronous active ice margins at multiple locations globally, the possibility of discrete, diachronous episodes of glaciation cannot be easily refuted (e.g., Young, 2014).
Grounding zone wedges do, however, offer some valuable insights into ice sheet characteristics and ice margin behaviour with important implications for the ongoing debates and efforts to model “snowball Earth” and other palaeoclimate states. Grounding zone wedges occur in marine settings (Batchelor and Dowdeswell, 2015; Simkins et al., 2018), confirming that the Makganyene Formation was deposited by marine-terminating ice sheets. Today, ice shelves form exclusively in settings where the mean annual isotherm remains below −5°C (Robin, 1979; Vaughan and Doake, 1996), supporting development of these landforms at the grounding zone of a cold or polythermal Palaeoproterozoic ice sheet. This is consistent with the absence of sedimentary or geomorphic evidence of meltwater re-working or channelization in the Makganyene sequence (e.g., Dowdeswell et al., 2016; Dietrich and Hoffman, 2019). Their close genetic association with an ice stream indicates that these fast-flowing corridors of ice also drained Palaeoproterozoic ice sheets, in line with the dynamic ice mass behaviour of Ordovician (e.g., Moreau et al., 2005), Late Palaeozoic (e.g., Andrews et al., 2019), and Pleistocene palaeo-ice sheets (e.g., Livingstone et al., 2012), and contemporary ice sheets in Greenland (e.g., Riverman et al., 2019) and Antarctica (e.g., King et al., 2009). This fast ice flow is considered an essential prerequisite for the rapid and fairly continuous sediment flux to the grounding zone necessary to accumulate these landforms (Dowdeswell et al., 2016). Finally, GZW deposition during still-stands or minor re-advances in overall ice sheet retreat is indicative of episodic grounding zone stability (Batchelor and Dowdeswell, 2015; Simkins et al., 2018). In fact, their deposition is thought to have a direct influence on stabilizing grounding zone positions by building positive relief (Alley et al., 2007). Comparison to recent continental margins suggests these periods of stabilization may be on the order of decades to millennia (Anandakrishnan et al., 2007; Nygard et al., 2007; Jakobsson et al., 2012; Klages et al., 2014; Bart et al., 2017, 2018), where longer periods of stabilization may drive higher magnitude and/or more unpredictable subsequent ice retreat (Simkins et al., 2018). Palaeoproterozoic deglaciation, at least in present day South Africa, may therefore be characterised by irregular duration retreat events punctuated by episodes of ice-margin stability.
Conclusion
Reappraisal of the Makganyene Formation using combined field and core data reaffirms the glacial influence on sedimentation first made 50 years ago (Visser, 1971). The best specific evidence is striated clasts and lonestones puncturing or downwarping laminae, which allows them to be interpreted as dropstones. Our new data and analysis also enables us to present further evidence for at least some reworking of the diamictites by mass flow processes. In contrast to interpretations presented for similar diamictite-dominated successions elsewhere, we suggest that the punctuated coarsening up motif of the diamictites represents the complex development of an ice grounding zone wedge. In this way, the process of ice-contact sedimentation is argued to have played a defining role on the resultant architecture of the deposits.
Data availability statement
The original contributions presented in the study are included in the article/Supplementary Material, further inquiries can be directed to the corresponding author.
Author contributions
DL: manuscript. SW, MB, and AS: Fieldwork, data collection. MB and AS: Editing of manuscript.
Acknowledgments
We wish to thank Thomas Rambuda and other staff from South 32 in Hotazel; Deon Nel and other staff from Kumba Iron Ore in Postmasburg; the Council for Geoscience in Donkerhoek, Pretoria. For consultation on field locations and drill core, Nic Beukes. For financial support we thank 1) The PPM Research Group at the Department of Geology at the University of Johannesburg, and 2) The Department of Geology at the University of Vienna. We are very grateful both to LI for her exemplary review of our paper, which challenged us to think very deeply and definitely improved it, and equally to KK for extremely valuable, constructive comments. We also thank the editors, John Isbell and FV, for their patience with us as we tried to complete the revisions in a reasonable timeframe in spite of the ongoing challenges of COVID that we all faced.
Conflict of interest
The authors declare that the research was conducted in the absence of any commercial or financial relationships that could be construed as a potential conflict of interest.
Publisher’s note
All claims expressed in this article are solely those of the authors and do not necessarily represent those of their affiliated organizations, or those of the publisher, the editors and the reviewers. Any product that may be evaluated in this article, or claim that may be made by its manufacturer, is not guaranteed or endorsed by the publisher.
References
Alley, R. B., Anandakrishnan, S., Dupont, T. K., Parizek, B. R., and Pollard, D. (2007). Effect of sedimentation on ice-sheet grounding-line stability. Science 315, 1838–1841. doi:10.1126/science.1138396
Anandakrishnan, S., Catania, G. A., Alley, R. B., and Horgan, H. J. (2007). Discovery of till deposition at the grounding line of whillans ice stream. Science 315, 1835–1838. doi:10.1126/science.1138393
Andrews, G. D., McGrady, A. T., Brown, S. R., and Maynard, S. M. (2019). First description of subglacial megalineations from the late Paleozoic ice age in southern Africa. PLoS One 14 (1), e0210673. doi:10.1371/journal.pone.0210673
Araújo, R., and Nogueira, A. (2019). Serra sul diamictite of the carajás basin (Brazil): A paleoproterozoic glaciation on the amazonian craton. Geology 47, 1166–1170. doi:10.1130/G46923.1
Assine, M. L., de Santa Ana, H., Veroslavsky, G., and Vesely, F. F. (2018). Exhumed subglacial landscape in Uruguay: Erosional landforms, depositional environments, and paleo–ice flow in the context of the late Paleozoic Gondwanan glaciation. Sediment. Geol. 369, 1–12. doi:10.1016/j.sedgeo.2018.03.011
Bamber, J. L., Vaughan, D. G., and Joughin, I. (2000). Widespread complex flow in the interior of the antarctic ice sheet. Science 287, 1248–1250. doi:10.1126/science.287.5456.1248
Bart, P. J., DeCesare, M., Rosenheim, B. E., Majewski, W., and McGlannan, A. (2018). A centuries long delay between a paleo-ice-shelf collapse and grounding-line retreat in the Whales Deep Basin, eastern Ross Sea, Antarctica. Sci. Rep. 8 (1), 12392–12399. doi:10.1038/s41598-018-29911-8
Bart, P. J., Krogmeier, B. J., Bart, M. P., and Tulaczyk, S. (2017). The paradox of a long grounding during west antarctic ice sheet retreat in ross sea. Sci. Rep. 7, 1262–1268. doi:10.1038/s41598-017-01329-8
Batchelor, C. L., and Dowdeswell, J. A. (2015). Ice-sheet grounding-zone wedges (GZWs) on high-latitude continental margins. Mar. Geol. 363, 65–92. doi:10.1016/j.margeo.2015.02.001
Bekker, A., Krapež, B., and Karhu, J. A. (2020). Correlation of the stratigraphic cover of the Pilbara and Kaapvaal cratons recording the lead up to Paleoproterozoic Icehouse and the GOE. Earth-Science Rev. 211, 103389. doi:10.1016/j.earscirev.2020.103389
Bell, T., Cooper, A. K., Solheim, A., Todd, B. J., Dowdeswell, J. A., et al. (2016). “Glossary of glaciated continental margins and related geoscience methods,”. Editors J. A. Dowdeswell, M. Canals, M. Jakobson, B. J. Todd, E. K. Dowdeswell, and K. A. Hogan (London, Memoirs: Geological Society), 46, 555–574.Atlas Submar. Glacial Landforms Mod. Quat. Anc.
Beukes, N. J., and Gutzmer, J. (2008). Origin and paleoenvironmental significance of major iron formations at the archean-paleoproterozoic boundary. Seg. Rev. 15, 5–47. doi:10.5382/Rev.15.01
Beukes, N. J. (1983). “Palaeoenvironmental setting of iron-formations in the depositional basin of the Transvaal Supergroup, South Africa,” in Iron-formations. Facts and problems. Editors A. F. Trendall, and S. C. Morris (Amsterdam: Elsevier), 131–209.
Beukes, N. J., and Smit, C. A. (1987). New evidence for thrust faulting in Griqualand West, South Africa: Implications for stratigraphy and the age of red beds. S. Afr. J. Geol. 90, 378–394.
Busfield, M. E., and Le Heron, D. P. (2018). Snowball Earth under the microscope. J. Sediment. Res. 88, 659–677. doi:10.2110/jsr.2018.34
Chen, X., Kuang, H., Liu, Y., Wang, Y., Yang, Y., Yang, Z., et al. (2020). Subglacial bedforms and landscapes formed by an ice sheet of Ediacaran-Cambrian age in west Henan, North China. Precambrian Res. 344, 105727. doi:10.1016/j.precamres.2020.105727
Chumakov, N. M. (2015). The role of glaciations in the biosphere. Russ. Geol. Geophys. 56, 541–548. doi:10.1016/j.rgg.2015.03.006
De Villiers, P. R., and Visser, J. N. J. (1977). The glacial beds of the Griqualand West Supergroup as revealed by four deep boreholes between Postmasburg and Sishen. Trans. Geol. Soc. S. Afr. 80, 1–8.
Demet, B. P., Nittrouer, J. A., Anderson, J. B., and Simkins, L. M. (2019). Sedimentary processes at ice sheet grounding-zone wedges revealed by outcrops, Washington State (USA). Earth Surf. Process. Landf. 44 (6), 1209–1220. doi:10.1002/esp.4550
Dietrich, P., Franchi, F., Setlhabi, L., Prevec, R., and Bamford, M. (2019). The nonglacial diamictite of toutswemogala Hill (lower Karoo Supergroup, central Botswana): Implications on the extent of the late paleozoic ice age in the kalahari-Karoo Basin. J. Sediment. Res. 89, 875–889. doi:10.2110/jsr.2019.48
Dietrich, P., Griffis, N. P., Le Heron, D. P., Montañez, I. P., Kettler, C., Robin, C., et al. (2021). Fjord network in Namibia: A snapshot into the dynamics of the late paleozoic glaciation. Geology 49, 1521–1526. doi:10.1130/G49067.1
Dietrich, P., and Hofmann, A. (2019). I ce‐margin fluctuation sequences and grounding zone wedges: The record of the Late Palaeozoic Ice Age in the eastern Karoo Basin (Dwyka Group, South Africa ). Depos. Rec. 5, 247–271. doi:10.1002/dep2.74
Domack, E. W., and Hoffman, P. F. (2011). An ice grounding-line wedge from the Ghaub glaciation (635 Ma) on the distal foreslope of the Otavi carbonate platform, Namibia, and its bearing on the snowball Earth hypothesis. Geol. Soc. Am. Bull. 123, 1448–1477. doi:10.1130/B30217.1
Dowdeswell, J. A., Canals, M., Jakobsson, M., Todd, B. J., Dowdeswell, E. K., and Hogan, K. A. (2016). “The variety and distribution of submarine glacial landforms and implications for ice-sheet reconstruction,”. Editors J. A. Dowdeswell, M. Canals, M. Jakobson, B. J. Todd, E. K. Dowdeswell, and K. A. Hogan (London, Memoirs: Geological Society), 46, 519–552.Atlas Submar. Glacial Landforms Mod. Quat. Anc.
Dowdeswell, J. A., Hogan, K. A., and Le Heron, D. P. (2019). “The glacier-influenced marine record on high-latitude continental margins: Synergies between modern, quaternary and ancient evidence,”. Editors D. P. Le Heron, K. A. Hogan, E. R. Phillips, M. Huuse, M. E. Busfield, and A. G. C. Graham (Bath, UK: Geological Society, London, Special Publications), 475, 261–279.Glaciat. Margins Sediment. Geophys. Archive
Escutia, C., Eittreim, S. L., Cooper, A. K., and Nelson, C. H. (2000). Morphology and acoustic character of the Antarctic Wilkes Land turbidite systems: Ice-sheet sourced versus riversourced fans. J. Sediment. Res. 70 (1), 84–93. doi:10.1306/2DC40900-0E47-11D7-8643000102C1865D
Evans, D. A., Beukes, N. J., and Kirschvink, J. L. (1997). Low-latitude glaciation in the Paleoproterozoic era. Nature 386, 262–266. doi:10.1038/386262a0
Eyles, C. (1988). A model for striated boulder pavement formation on glaciated, shallow-marine shelves; an example from the Yakataga Formation, Alaska. J. Sediment. Res. 58, 62–71. doi:10.1306/212F8D14-2B24-11D7-8648000102C1865D
Eyles, N. (2008). Glacio-epochs and the supercontinent cycle after ∼ 3.0 Ga: Tectonic boundary conditions for glaciation. Palaeogeogr. Palaeoclimatol. Palaeoecol. 258, 89–129. doi:10.1016/j.palaeo.2007.09.021
Eyles, N., and Januszczak, N. (2004). ‘Zipper-rift’: A tectonic model for neoproterozoic glaciations during the breakup of rodinia after 750 Ma. Earth-Science Rev. 65, 1–73. doi:10.1016/S0012-8252(03)00080-1
Eyles, N., and Lazorek, M. (2014). Glacigenic lithofacies sediments in glaciated landscapes. In: reference module in earth systems and environmental sciences. Elsevier. doi:10.1016/B978-0-12-409548-9.09427-6
Graham, A. G., Larter, R. D., Gohl, K., Dowdeswell, J. A., Hillenbrand, C. D., Smith, J. A., et al. (2010). Flow and retreat of the late quaternary pine island‐thwaites palaeo‐ice stream, west Antarctica. J. Geophys. Res. 115 (3), F03025. doi:10.1029/2009jf001482
Gumsley, A. P., Chamberlain, K. R., Bleeker, W., Söderland, U., de Kock, M. O., Larsson, E. R., et al. (2017). Timing and tempo of the great oxidation event. Proc. Natl. Acad. Sci. U. S. A. 114, 1811–1816. doi:10.1073/pnas.1608824114
Henstra, G. A., Grundvåg, S. A., Johannessen, E. P., Kristensen, T. B., Midtkandal, I., Nystuen, J. P., et al. (2016). Depositional processes and stratigraphic architecture within a coarse-grained rift-margin turbidite system: The Wollaston Forland Group, east Greenland. Mar. Petroleum Geol. 76, 187–209. doi:10.1016/j.marpetgeo.2016.05.018
Hoffman, P. F., Macdonald, F. A., and Halverson, G. P. (2011). Chemical sediments associated with Neoproterozoic glaciation: Iron formation, cap carbonate, barite and phosphorite. Geol. Soc. Mem. 36, 17–37. doi:10.1144/M36.5
Hoffman, P. F. (2013). The great oxidation and a siderian snowball Earth: MIF-S based correlation of paleoproterozoic glacial epochs. Chem. Geol. 362, 143–156. doi:10.1016/j.chemgeo.2013.04.018
Ives, L. R. W. (2021). Physical Sedimentology and provenance of glacial successions in the tasmanian and transantarctic basins. PhD Thesis. University of Wisconsin – Milwaukee. doi:10.13140/RG.2.2.35028.27522Chapter 3: Contrasting styles of glacial sedimentation and glacier thermal regimes in the lower wynyard formation (pennsylvanian – early permian, tasmanian basin) in A south polar view of late paleozoic glaciation
Jakobsson, M., Anderson, J. B., Nitsche, F. O., Gyllencreutz, R., Kirshner, A. E., Kirchner, N., et al. (2012). Ice sheet retreat dynamics inferred from glacial morphology of the central Pine Island Bay Trough, West Antarctica. Quat. Sci. Rev. 38 (1–10), 1–10. doi:10.1016/j.quascirev.2011.12.017
Kennedy, K., Eyles, N., and Broughton, D. (2019). Basinal setting and origin of thick (1·8 km) mass-flow dominated Grand Conglomérat diamictites, Kamoa, Democratic Republic of Congo: Resolving climate and tectonic controls during Neoproterozoic glaciations. Sedimentology 66, 556–589. doi:10.1111/sed.12494
Kennedy, K., and Eyles, N. (2019). Subaqueous debrites of the grand conglomérat formation, democratic republic of Congo: A model for anomalously thick neoproterozoic: ‘‘glacial’’ diamictites. J. Sediment. Res. 89, 935–955. doi:10.2110/jsr.2019.51
King, E. C., Hindmarsh, R. C., and Stokes, C. R. (2009). Formation of mega-scale glacial lineations observed beneath a West Antarctic ice stream. Nat. Geosci. 2, 585–588. doi:10.1038/ngeo581
Kirschvink, J. L., Gaidos, E. J., Bertani, E., Beukes, N. J., Gutzmer, J., Maepa, L. N., et al. (2000). Paleoproterozoic snowball Earth: Extreme climatic and geochemical global change and its biological consequences. Proc. Natl. Acad. Sci. U. S. A. 7, 1400–1405. doi:10.1073/pnas.97.4.1400
Klages, J. P., Kuhn, G., Hillenbrand, C. D., Graham, A. G., Smith, J. A., Larter, R. D., et al. (2014). Retreat of the west antarctic ice sheet from the Western amundsen sea shelf at a pre-or early LGM stage. Quat. Sci. Rev. 91, 1–15. doi:10.1016/j.quascirev.2014.02.017
Kopp, R. E., Kirschvink, J. L., Hilburn, L. A., and Nash, C. Z. (2005). The paleoproterozoic snowball Earth: A climate disaster triggered by the evolution of oxygenic photosynthesis. Proc. Natl. Acad. Sci. U. S. A. 102, 11131–11136. doi:10.1073/pnas.0504878102
Lantink, M. L., Oonk, P. B. H., Floor, G. H., Tsikos, H., and Mason, P. R. D. (2018). Fe isotopes of a 2.4 Ga hematite-rich IF constrain marine redox conditions around the GOE. Precambrian Res. 305, 218–235. doi:10.1016/j.precamres.2017.12.025
Le Heron, D. P., Busfield, M. E., Chen, X., Corkeron, M., Davies, B. J., Dietrich, P., et al. (2022). New perspectives on glacial geomorphology in earth’s deep time record. Front. Earth Sci. (Lausanne). 10. doi:10.3389/feart.2022.870359
Le Heron, D. P., Busfield, M. E., and Kettler, C. (2021). Ice-rafted dropstones in “postglacial” Cryogenian cap carbonates. Geology 49, 263–267. doi:10.1130/G48208.1
Le Heron, D. P., Eyles, N., and Busfield, M. E. (2020). The laurentian neoproterozoic glacial interval: Reappraising the extent and timing of glaciation. Austrian J. Earth Sci. 113, 59–70. doi:10.17738/ajes.2020.0004
Le Heron, D. P. (2015). The significance of ice-rafted debris in Sturtian glacial successions. Sediment. Geol. 322, 19–33. doi:10.1016/j.sedgeo.2015.04.001
Le Heron, D. P., Tofaif, S., Vandyk, T., and Ali, D. O. (2017). A diamictite dichotomy: Glacial conveyor belts and olistostromes in the Neoproterozoic of Death Valley, California, USA. Geology 45, 31–34. doi:10.1130/g38460.1
Le Heron, D. P., Vandyk, T. M., Hongwei, K., Liu, Y., Chen, X., Wang, Y., et al. (2019). Bird's-eye view of an Ediacaran subglacial landscape. Geology 47, 705–709. doi:10.1130/g46285.1
Lechte, M., and Wallace, M. (2016). Sub-ice shelf ironstone deposition during the Neoproterozoic Sturtian glaciation. Geology 44, 891–894. doi:10.1130/g38495.1
Li, S., Junkin, W. D., Gaschnig, R. M., Ash, R. D., Piccoli, P. M., Candela, P. A., et al. (2020). Molybdenum contents of sulfides in ancient glacial diamictites: Implications for molybdenum delivery to the oceans prior to the Great Oxidation Event. Geochim. Cosmochim. Acta 278, 30–50. doi:10.1016/j.gca.2019.09.011
Livingstone, S. J., Cofaigh, C. Ó., Stokes, C. R., Hillenbrand, C. D., Vieli, A., and Jamieson, S. S. (2012). Antarctic palaeo-ice streams. Earth-Science Rev. 111, 90–128. doi:10.1016/j.earscirev.2011.10.003
Lowe, D. R. (1982). Sediment gravity flows; II, Depositional models with special reference to the deposits of high-density turbidity currents. J. Sediment. Res. 52 (1), 279–297.
Melezhik, V. A., Young, G. M., Eriksson, P. G., Altermann, W., Kump, L. R., and Lepland, A. (2013). “Huronian-age glaciation,”. Editors V. A. Melezhik, R. Prave, E. J. Hanski, A. E. Fallick, A. Lepland, L. R. Kumpet al. (Berlin Heidlberg: Reading the Archive of Earth’s Oxygenation), 3, 1059–1109.Glob. Events Fennoscandian Arct. Russ. – Drill. Early Earth Proj.
Moore, J. M., Tsikos, H., and Polteau, S. (2001). Deconstructing the transvaal Supergroup, south Africa: Implications for palaeoproterozoic palaeoclimate models. J. Afr. Earth Sci. 33, 437–444. doi:10.1016/s0899-5362(01)00084-7
Moreau, J. (2011). The late ordovician deglaciation sequence of the SW murzuq basin (Libya). Basin Res. 23, 449–477. doi:10.1111/j.1365-2117.2010.00499.x
Mulder, T., and Alexander, J. (2001). The physical character of subaqueous sedimentary density flows and their deposits. Sedimentology 48 (2), 269–299. doi:10.1046/j.1365-3091.2001.00360.x
Nascimento, D. B., Ribeiro, A., Trouw, R. A. J., Schmitt, R. S., and Passchier, C. W. (2016). Stratigraphy of the Neoproterozoic Damara Sequence in northwest Namibia: Slope to basin sub-marine mass transport deposits and olistolith fields. Precambrian Res. 278, 108–125. doi:10.1016/j.precamres.2016.03.005
Nygård, A., Sejrup, H. P., Haflidason, H., Lekens, W. A. H., Clark, C. D., and Bigg, G. R. (2007). Extreme sediment and ice discharge from marine-based ice streams: New evidence from the North Sea. Geol. 35, 395–398. doi:10.1130/g23364a.1
Ó Cofaigh, C., Dowdeswell, J. A., Allen, C. S., Hiemstra, J. F., Pudsey, C. J., Evans, J., et al. (2005). Flow dynamics and till Genesis associated with a marine-based Antarctic palaeo-ice stream. Quat. Sci. Rev. 24, 709–740. doi:10.1016/j.quascirev.2004.10.006
Ottesen, D., and Dowdeswell, J. A. (2009). An inter-ice stream glaciated margin: Submarine landforms and a geomorphic model based on marine-geophysical data from svalbard. Geol. Soc. Am. Bull. 121, 1647–1665. doi:10.1130/b26467.1
Ottesen, D., Stokes, C. R., Rise, L., and Olsen, L. (2008). Ice-sheet dynamics and ice streaming along the coastal parts of northern Norway. Quat. Sci. Rev. 27 (9-10), 922–940. doi:10.1016/j.quascirev.2008.01.014
Polteau, S., Moore, J. M., and Tsikos, H. (2006). The geology and geochemistry of the Palaeoproterozoic Makganyene diamictite. Precambrian Res. 148, 257–274. doi:10.1016/j.precamres.2006.05.003
Powell, R. D., and Alley, R. B. (1997). “Grounding-line systems: Processes, glaciological inferences and the stratigraphic record,”. Editors P. F. Barker, and A. K. Cooper (American Geophysical Union), 71, 169–187.Geol. Seismic Stratigr. Antarct. Margin 2
Rasmussen, B., Bekker, A., and Fletcher, I. R. (2013a). Correlation of Paleoproterozoic glaciations based on U-Pb zircon ages for tuff beds in the Transvaal and Huronian Supergroups. Earth Planet. Sci. Lett. 382, 173–180. doi:10.1016/j.epsl.2013.08.037
Riverman, K. L., Anandakrishnan, S., Alley, R. B., Holschuh, N., Dow, C. F., Muto, A., et al. (2019). Wet subglacial bedforms of the NE Greenland Ice Stream shear margins. Ann. Glaciol. 60, 91–99. doi:10.1017/aog.2019.43
Robin, G. de Q. (1979). Formation, flow and disintegration of ice shelves. J. Glaciol. 24, 259–271. doi:10.1017/s0022143000014787
Rogers, A. W. (1906). The glacial beds in the griqua town series. Rep. S Afr. Assoc. Adv. Sci. 4, 261–265.
Schermerhorn, L. J. G. (1974). Late precambrian mixites: Glacial and/or nonglacial? Am. J. Sci. 274, 673–824. doi:10.2475/ajs.274.7.673
Schröder, S., Bedorf, D., Beukes, N. J., and Gutzmer, J. (2011). From BIF to red beds: Sedimentology and sequence stratigraphy of the paleoproterozoic Koegas Subgroup (south Africa). Sediment. Geol. 236, 25–44. doi:10.1016/j.sedgeo.2010.11.007
Schroeder, S., Bedorf, D., Beukes, N. J., and Gutzmer, J. (2011). From BIF to red beds: Sedimentology and sequence stratigraphy of the paleoproterozoic Koegas Subgroup (south Africa). Sediment. Geol. 236, 25–44. doi:10.1016/j.sedgeo.2010.11.007
Simkins, L. M., Greenwood, S. L., and Anderson, J. B. (2018). Diagnosing ice sheet grounding line stability from landform morphology. Cryosphere 12, 2707–2726. doi:10.5194/tc-12-2707-2018
Smith, A. J. B. (2018). “The iron formations of southern Africa,” in Geology of southwest gondwana. Regional Geology reviews. Editors S. Siegesmund, M. Basei, P. Oyhantçabal, and S. Oriolo (Cham: Springer). doi:10.1007/978-3-319-68920-3_17
Spence, G., Le Heron, D. P., and Fairchild, I. J. (2016). Sedimentological perspectives on climatic, atmospheric and environmental change in the neoproterozoic Era. Sedimentology 63, 253–306. doi:10.1111/sed.12261
Thorndycraft, V. R., Cripps, J. E., and Eades, G. L. (2016). Digital landscapes of deglaciation: Identifying late quaternary glacial lake outburst floods using LiDAR. Earth Surf. Process. Landf. 41, 291–307. doi:10.1002/esp.3780
Tofaif, S., Vandyk, T. M., Le Heron, D. P., and Melvin, J. (2019). Glaciers, flows, and fans: Origins of a neoproterozoic diamictite in the saratoga Hills, death valley, California. Sediment. Geol. 385, 79–95. doi:10.1016/j.sedgeo.2019.03.003
Vaughan, D. G., and Doake, C. S. M. (1996). Recent atmospheric warming and retreat of ice shelves on the Antarctic Peninsula. Nature 379 (6563), 328–331. doi:10.1038/379328a0
Visser, J. N. J. (1971). The deposition of the Griquatown glacial member in the Transvaal Supergroup. Trans. Geol. Soc. S Afr. 74, 187–199.
Visser, J. N. J. (1981). “The mid-precambrian tillite in the Griqualand West and transvaal basins, south Africa,” in Earth’s pre-pleistocene glacial record. Editor Harland Hambrey (Cambridge: Cambridge University Press), 180–184.
Wendorff, M. (2000). Genetic aspects of the katangan megabreccias: Neoproterozoic of central Africa. J. Afr. Earth Sci. 26, 703–715. doi:10.1016/s0899-5362(00)00047-6
Winsemann, J., Asprion, U., Meyer, T., and Schramm, C. (2007). Facies characteristics of Middle Pleistocene (Saalian) ice-margin subaqueous fan and delta deposits, glacial Lake Leine, NW Germany. Sediment. Geol. 193 (1-4), 105–129. doi:10.1016/j.sedgeo.2005.11.027
Young, G. M. (2014). Contradictory correlations of Paleoproterozoic glacial deposits: Local, regional or global controls? Precambrian Res. 247, 33–44. doi:10.1016/j.precamres.2014.03.023
Young, G. M. (2013). Precambrian supercontinents, glaciations, atmospheric oxygenation, metazoanevolution and an impact that may have changed the second half of Earth history. Geosci. Front. 4, 247–261. doi:10.1016/j.gsf.2012.07.003
Keywords: diamictite, Siderian, proterozoic, grounding zone wedge, glacial
Citation: Le Heron DP, Busfield ME, Smith AJB and Wimmer S (2022) A grounding zone wedge origin for the Palaeoproterozoic Makganyene Formation of South Africa. Front. Earth Sci. 10:905602. doi: 10.3389/feart.2022.905602
Received: 27 March 2022; Accepted: 31 August 2022;
Published: 28 September 2022.
Edited by:
Fernando Vesely, Federal University of Paraná, BrazilReviewed by:
Libby Ives, United States Geological Survey (USGS), United StatesKirsten Kennedy, University of Toronto Scarborough, Canada
Copyright © 2022 Le Heron, Busfield, Smith and Wimmer. This is an open-access article distributed under the terms of the Creative Commons Attribution License (CC BY). The use, distribution or reproduction in other forums is permitted, provided the original author(s) and the copyright owner(s) are credited and that the original publication in this journal is cited, in accordance with accepted academic practice. No use, distribution or reproduction is permitted which does not comply with these terms.
*Correspondence: D. P. Le Heron, RGFuaWVsLmxlLWhlcm9uQHVuaXZlLmFjLmF0