- 1School of Earth Sciences, University of Bristol, Bristol, United Kingdom
- 2Institute of Vertebrate Paleontology and Paleoanthropology, Chinese Academy of Sciences, Beijing, China
The Triassic has long been recognized as a time during which marine and terrestrial ecosystems modernized dramatically, and it seems to have been a two-step process. First, recovery from the Permian-Triassic mass extinction (PTME) was a time of extraordinary renewal and novelty, and these processes of change were enhanced, it seems, by the effects of the Carnian Pluvial Episode (CPE). After the CPE, in the oceans, not only did the carbonate factory begin to change towards its modern form, but also arguably the Mesozoic Marine Revolution (MMR) speeded up. When the MMR was proposed it was seen as a process that occurred in the Late Jurassic and Cretaceous, as modern crustaceans, gastropods, and fishes enhanced predator-prey arms races. New evidence from China and elsewhere suggests in fact the MMR was already underway in the Middle and Late Triassic, and so was coincident with Sepkoski’s classic idea that Paleozoic faunas were replaced by Modern marine faunas from the beginning of the Triassic. On land, ongoing competition between synapsids and archosauromorphs through the Triassic was marked by a posture shift from sprawling to erect, and a shift in physiology to warm-bloodedness, with insulating skin coverings of hair and feathers. Dinosaurs, for example, originated in the Early or Middle Triassic, but did not diversify until after the CPE. These arms races, the MMR in the sea, and the endothermy shift in tetrapods, were triggered by the PTME, and then enhanced by the CPE.
Introduction
When Phillips (1841) named the geological eras, the Palaeozoic, Mesozoic, and Kainozoic (now, Cenozoic), he noted that each was characterized by very different kinds of animals and plants, and that their boundaries marked major shifts in the faunas and floras. He intended these stratigraphic terms to be overtly biological, reflecting the nature of the fossils, and paleontologists have since understood that the boundaries between Paleozoic and Mesozoic and Mesozoic and Cenozoic mark the end-Permian and end-Cretaceous mass extinctions, dated respectively, at 252 and 66 Ma (million years ago). The times following these two mass extinctions represent notable episodes of the recovery of life, during the Triassic and Paleogene periods respectively. The succession from dinosaurs to mammals and birds in the Paleogene has been described many times, as well as the evolution of new ecosystems in the oceans after the extinctions of marine reptiles, ammonites, belemnites and rudists at the end of the Cretaceous. The recovery of life in the Triassic after the much more severe end-Permian event has long been seen as even more dramatic.
Van Valen (1984) identified the Permian-Triassic boundary (PTB) as a singularity in the evolution of Phanerozoic marine animals, marked by a shift in evolutionary dynamics. Similarly, Sepkoski (1984) argued the “Modern Evolutionary Fauna” emerged after the PTB, in succession to his “Cambrian” and “Paleozoic” evolutionary faunas, but he saw very little evidence for a step-change across the Cretaceous-Paleogene boundary. Whereas the Paleozoic marine fauna had been dominated by brachiopods, rugose corals, tabulate corals, cephalopods, trilobites, graptolites, and crinoids, and lower actinopterygian fishes (e.g., “paleonisciform” or “paleopterygian” fishes; Romano et al., 2016) and non-neoselachian sharks, the Modern fauna, commencing in the Triassic, was characterized by bivalves, gastropods, malacostracan crustaceans (crabs, lobsters), echinoids, neoselachian sharks, neopterygian bony fishes (including basal holosteans and teleosts), and marine tetrapods (reptiles in the Mesozoic, whales in the Cenozoic). These changes also reflect sharp rises from Paleozoic to Mesozoic in the proportions of motile (free-swimming) animals to non-motile (bottom-dwelling) and in the proportions of predators to non-predators (Bambach et al., 2002). The major changes were set in train following the devastating effects of the Permian-Triassic mass extinction (PTME).
In considering the timing of major events in the Triassic, the Carnian Pluvial Episode (CPE), dated from 233 to 232 Ma, has also emerged as potentially very important in punctuating the rise of modern ecosystems on land and in the oceans (Simms and Ruffell, 1989; Roghi et al., 2010; Dal Corso et al., 2020). This episode was triggered by repeated eruptions of the Wrangellia Large Igneous Province, which led to a series of consequences similar to those that occurred during the PTME, including sharp global warming and especially in tropical latitudes, acid rain and mass wasting on land, and ocean acidification and seabed anoxia in the oceans. Through a million years, repeated eruptions drove temperatures up and generated excess rainfall on land, but after the eruptions ceased some 232 Ma, the CPE humid phase reverted to aridity, and it was the humid-to-arid switch that led to substantial extinctions and major changes in ecosystems (Roghi et al., 2010; Dal Corso et al., 2020). In the sea, the first scleractinian reefs and rock-forming calcareous nannofossils diversified, both reflecting and enabling substantial changes in ocean chemistry (Dal Corso et al., 2020). Some new predatory clades that had originated in the aftermath of the PTME (e.g., decapod crustaceans, coleoid cephalopods, asteroid and cidaroid echinoderms, neopterygian bony fishes, neoselachian sharks) diversified substantially at this point, marking a step-change in predatory attack (e.g., durophagy, hole-drilling) and defensive and escape strategies (e.g. cementation, deep burrowing, fast escape swimming). On land, there were major diversifications and originations of conifers, insects, dinosaurs, crocodiles, lepidosaurs, turtles, and mammals; most of these groups had originated much earlier, in the Early and Middle Triassic, but diversified and became ecologically significant after the CPE (Dal Corso et al., 2020). Thus emerged the roots of modern ecosystems.
In related, but parallel work, Vermeij (1977) identified the Mesozoic Marine Revolution as a key episode in the history of marine animals. He noted that typical Mesozoic and modern marine predators, such as malacostracan arthropods, gastropods, echinoids, and vertebrates (including neoselachian sharks, teleost fishes, and marine reptiles) were all faster and nastier than their Paleozoic precursors. As predators became faster to catch their prey, the prey evolved means of escape and defense, including a thicker shell or the ability to burrow deeper. Predatory modes also became more deadly, with many fishes and reptiles becoming durophages, capable of crushing thick-shelled oysters, and with gastropods perfecting their hole-piercing equipment to penetrate through shell to flesh, and sharks and teleosts with mobile mouth parts to enlarge their gapes. Vermeij pointed to the origins of many of these adaptations in the Cretaceous and suggested the MMR began in the Jurassic. Based on new evidence, Vermeij (2008) revised his original view, and noted an early burst of the MMR in the Late Triassic and Early Jurassic, and since then additional evidence has revealed origins of many key MMR predators in the Early and Middle Triassic (Hu et al., 2011; Benton et al., 2013; Tackett, 2016; Tackett and Bottjer, 2016; Brayard et al., 2017; Waller, 2006; Hautmann et al., 2017), and if this is true, it links the initial driver of the MMR to the recovery of life following the PTME and the origin of modern-style ecosystems in the oceans.
Among vertebrates in particular, the nature of their recovery also seems to mark something unusual. Certain clades such as fishes and tetrapods showed very rapid diversifications in the sea (Benton et al., 2013; Scheyer et al., 2014; Motani et al., 2015) and on land (Ezcurra, 2016; Ezcurra et al., 2020a, b; Benton, 2021). Indeed, the changes among ecologically dominant tetrapods on land, cutting across the major clades of archosaurs and synapsids, seem to mark a quickening of life in general. Both archosaurs (including the ancestors of dinosaurs and birds) and synapsids (including the ancestors of mammals) show evidence of a major shift in posture (sprawling to upright) and in thermal physiology (ectothermy to endothermy). These changes affected the nature of energy transfer through ecosystems, so they share more in common with modern than Paleozoic ecosystems. Importantly, changes initiated by the PTME appear to have been accelerated by the CPE, which triggered remarkable changes in plants, insects, and tetrapods, including the origins of dinosaurs and of mammals.
Here, we explore critical points about the Triassic, and with a key focus on the CPE and whether the combined “double hit” of the hugely devastating PTME, and then the smaller, but hugely influential, CPE 20 Myr later marked the origins of modern ecosystems in the sea and on land.
Early Start of the Mesozoic Marine Revolution
Definition of the Mesozoic Marine Revolution
In considering the recovery of life after the PTME, and when the MMR started, it is important to recall that there had been a long history of increasingly effective predatory and defensive strategies among marine animals throughout the Paleozoic, and especially with some escalation in the Devonian and Carboniferous (Brett and Walker, 2002), and with renewal of Paleozoic predatory modes in the new world of the Triassic as well as further escalation (Walker and Brett, 2002). A key question is when the MMR began, and whether a case can be made plausibly that the MMR is a part of, or equivalent with, the Triassic recovery of life from the rigors of the PTME.
In his original works, Vermeij (1977) and Vermeij et al. (1981) made the case that the MMR began in the Cretaceous, based on several lines of evidence: 1) an increase in snail-shell sturdiness in response to the evolution of new shell-destroying predators such as teleosts, stomatopods, and decapod crustaceans, 2) an intensification of grazing, and 3) deeper burrowing than ever seen before in certain taxa, a classic means of escape from intense predation. He noted that these new behaviors coincided with the origin of several clades that dominate oceans today, including mesogastropods, neogastropods, stomatopod crustaceans, and shell-crushing rays and teleosts. Vermeij et al. (1981) tested the timing of the MMR by focusing on shell damage in gastropods through the Phanerozoic, and they identified a step-change in such damage at some point between the Late Triassic and Late Cretaceous but did not have sufficient sampling to say when this had occurred other than at some point in the Jurassic.
In his review of 46 innovations among marine animals throughout the Mesozoic, Vermeij (2008) noted a two-step model for the MMR, with bursts of innovation in the Late Triassic–Early Jurassic interval (0.42 innovations per million years) and in the mid–Late Cretaceous (0.60) separated by a time of only modest innovation from Middle Jurassic–Early Cretaceous (0.12). Marine innovations in the Carnian included origins of calcified phytoplankton (dinoflagellates, calcified nannoplankton), compound plates in echinoids, giant marine tetrapods, turtle form in tetrapods, articulating teeth in hinges of cementing bivalves, suckered arms in squid, diverse zooxanthellate reef-building corals, bioerosive herbivory, and obligately deep-boring bivalves.
Re-Dating the MMR
Vermeij’s (2008) analysis accepted the idea of a Late Triassic start to the MMR, which we could now indicate was part of the aftermath of the CPE, but there is still a question around whether the MMR kicked off even earlier, in the Early and Middle Triassic. Vermeij (2008) enumerated a list of 46 predatory and defensive-escape innovations seen in Mesozoic organisms, including 31 marine and 15 terrestrial examples. We repeat this analysis in the sense of listing innovations relevant to the predator-prey escalation in the Triassic but excluding his terrestrial examples.
The numerical counts presented by Vermeij (2008) are arbitrary, and different authors might produce different listings, which makes the estimates of rates of change only indicative, as he acknowledged. We do not present any such statistical metrics. In any case, there would be more appropriate approaches in ecological terms, estimating for example the proportions of predatory interactions of the “new” type versus others; this would allow discrimination between the maximal point of origin of an innovation and the point at which it became prevalent in ecosystems.
In many examples noted below, a related issue is to separate origins based on phylogenetic inference from origins based on abundant fossils. For example, some fiendish predatory adaptations of Mesozoic hunters, such as the muscular tentacles of hunting cephalopods armed with suckers and hooklets, are largely soft-tissue and so hard to fossilize, and yet phylogenetic evidence indicates likely points of origin before fossils became abundant. Does this mean the adaptation was widespread but poorly fossilized, or in fact only rarely present and so of negligible ecological importance? In the summary to follow, we will keep these concerns about data and methods in mind, and look at the carbonate factory first, then the new predators, and finally the new defenses against these predators.
Carbonate Factory-Plankton Revolution
Carbonate factories are the combination of sedimentary environment, organisms and precipitation processes that lead to the formation of a carbonate platform, and they can be divided into multiple systems, including tropical, cool-water, and mud-mound types (Schlager, 2005; Reijmer, 2021). Key controlling factors on these systems include light, water temperature, nutrients, salinity, substrate, and carbonate saturation; local controls are also ocean currents, upwelling and non-upwelling systems, ocean-atmosphere systems, atmospheric systems, shallow-water dynamics, and terrestrial sediment and water inputs (Reijmer, 2021).
The nature and location of the dominant carbonate factories through time have been critical in the regulation of ocean chemistry and cycling of carbon. When calcifying organisms arose in the Cambrian, the products of weathering were captured, and this affected the carbon cycle permanently. Then there was a dramatic shift in the main locus of the carbonate factory from continental shelves to deep seas and this followed the rise of calcifying plankton in the Late Triassic and Jurassic (Figure 1). The changes in ocean chemistry and function were profound (Ridgwell, 2005). Before the switch, in Paleozoic seas, this kind of “neritic ocean” was subject to extreme fluctuations in carbonate content and pH according to topography and climate, reflecting variable weathering on land and halving and doubling of the area of the continental shelf. The consequences were that the ocean could become extremely saturated with carbonates washed in from weathering on land at times of low sea level when areas for deposition of carbonates were restricted. After the ocean revolution when carbonate factories moved towards deeper waters, as today, such variations in saturation do not occur; the deep-sea carbonate sink enables the oceanic saturation state to self-regulate. This also had impacts on the concentration of carbon dioxide in the atmosphere and the degree of acidification (pH level) of the ocean itself.
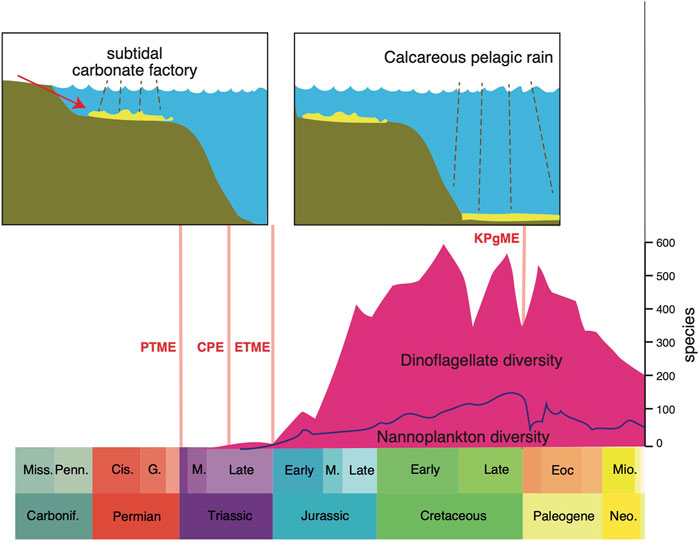
FIGURE 1. The major shift in the marine carbonate factory from the shelf-based model through the Palaeozoic and most of the Triassic, and the modern-style dep-water carbonate factory of today, which began in the latest Triassic. Abbreviations: Carbonif, Carboniferous; Cis, Cisuralian; CPE, Carnian Pluvial Episode; Eoc, Eocene; ETME, end-Triassic mass extinction; KPgME, Cretaceous-Paleogene mass extinction; M, Middle; Mio, Miocene; Miss, Mississippian; Neo, Neogene; Penn, Pennsylvanian; PTME, Permian-Triassic mass extinction. Dinoflagellate diversity through time from Fensome et al. (1996), Nannoplankton diversity from Bown et al. (2005), and sketch carbonate factory models based on James (1984).
In their review of 1.5 billion years of eukaryotic planktonic evolution, Falkowski et al. (2004) identified a major ecological reset in the Triassic that marked a comprehensive overhaul in how the oceanic carbon cycle worked. Before the reset, Paleozoic marine phytoplankton were perhaps less diverse and abundant, and less important in nutrient cycling. The extinction of many groups in the PTME marked the beginning of a new marine world dominated by the modern groups of phytoplankton (e.g., dinoflagellates, coccolithophores), which originated about the same time in the Middle and Late Triassic (Figure 1). The new eukaryotic plankton of the Triassic possessed plastids derived from an ancestral red alga that enabled them to develop a “dual fuel” metabolism, whereby they could retain fixed nitrogen within the cell, while simultaneously obtaining organic carbon via photosynthesis, and even assimilating dissolved and particulate organic matter (Falkowski et al., 2004). Initially, dinoflagellates and coccolithophores flourished in the relatively warm, quiescent Mesozoic seas, when wind speeds and oceanic thermohaline circulation were relatively sluggish. When the major polar icecaps emerged about 33 Ma in the early Oligocene (Zachos et al., 2001; Norris et al., 2013; Westerhold et al., 2020), climates cooled, winds freshened and ocean circulation speeded up, and diatoms began to replace dinoflagellates and coccolithophores as dominant eukaryotic phytoplankton in the oceans.
The transition from the neritic to deep-sea dominant ocean pattern was dated at the Triassic-Jurassic boundary by Ridgwell. (2005), and to the aftermath of the CPE by Dal Corso et al. (2020). Carbonate fluxes and nannoplankton diversity remained low through the Late Triassic and Early Jurassic, and production was restricted to epi-continental areas. The true colonization of the oceans occurred through a very long time, reaching an advanced stage in the Early Cretaceous, based on analysis of species diversity through time (Figure 1), but importantly also estimated accumulation rates on the ocean floor: rates of accumulation rose one-hundredfold, from 109 m2yr−1 in the Early Jurassic to 1011 m2yr−1 in the Early Cretaceous (Suchéras-Marx et al., 2019).
The concurrent rise of calcifying plankton groups in the oceans as well as new reef-building corals (scleractinians) and other metazoan reef-builders, contributed to a major shift in the global carbon cycle. Today, the three eukaryotic phytoplankton groups are responsible for the great majority of the export of organic matter to the ocean interior and into deep-ocean sediments (Falkowski et al., 2004). Their huge contribution to carbon cycling is documented in the Italian Dolomites where the rise of coccolithophores and dinoflagellates in the Carnian is marked by a huge increase in calcispheres in the carbonate rocks; their frequency rose from essentially 0% in the Middle Triassic to 10% after the CPE, and >50% of rock volume in the Rhaetian. As Dal Corso et al. (2020, p. 6) note, “The rise of these calcispheres may represent a milestone in Earth history that could have fundamentally changed the global carbon cycle and certainly deserves more attention.” At the same time, the “carbonate factory,” the processes of conversion of carbon into calcium carbonate within organisms, had occurred mainly in microbial reefs in earlier parts of the Triassic, and switched to metazoan reefs (i.e., corals, sponges, bryozoans) during and immediately after the CPE (Dal Corso et al., 2020). These together shifted the main sites of carbonate accumulation from the continental shelf to offshore locations, as reef debris was washed offshore, but more importantly, calcareous nannoplankton lived over deep waters as well as shelf waters, and shed their skeletons into deeper waters. These deep-water carbonates helped stabilize the global carbon cycle by improving the buffering capacity of the oceans (Ridgwell., 2005). This was a key switch in global ocean chemistry, marked by a shift of major carbonate reservoirs offshore and into deeper waters, which were less susceptible to fluctuating sea levels than the earlier carbonate accumulations on continental shelves.
New Predators
As noted earlier, the Triassic revolution in the oceans is marked not only by the beginning of the shift in the carbonate factory, but also by a great number of new predators and predatory modes, matched by many new defensive and escape strategies by the prey organisms (Figure 2).
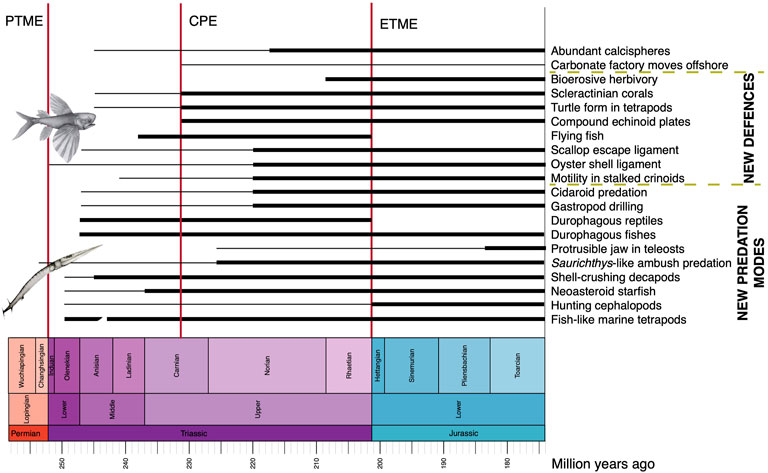
FIGURE 2. Novel predatory and defensive modes in Triassic seas, showing approximate timings of known fossil records, or times when a phenomenon became established (thick line) and earlier occurrences, either inferred from phylogeny or based on rare fossils (thin line). The flying fish Potanichthys and the ambush-predatory Saurichthys are by Fei-xiang Wu.
Predatory crustaceans, primarily shrimp- and lobster-like decapods, were widespread in the Triassic, and these include stem penaeoid and caridean shrimps and polychelidan and astacidean lobsters (Hegna et al., 2020). It is likely that crown families, known from fossils in the Jurassic, such as the Anomura (hermit crabs, squat lobsters, false crabs, stone crabs) and Brachyura (true crabs), will also yield fossils back into the Triassic, as indicated by molecular estimates (e.g., Bai et al., 2018) that substantial diversifications followed the PTME, in the Early to Middle Triassic. Modern families identified so far in the Triassic include Penaeidae (prawns and shrimps), Palinuridae and Glypheidae (rock and glypheid lobsters), and Upogebiidae (mud shrimps). New fossil finds from the Middle Triassic (Anisian) of China show the Luoping fauna included five decapod species, capable of variously hunting and scavenging their prey (Feldmann et al., 2012). In their review of decapod hunting modes, Schweitzer and Feldmann (2010) noted that decapods had adaptations for shell-crushing since the Early Triassic.
Coleoid cephalopods, squid, cuttlefish, octopuses, and belemnoids, diversified substantially through the Mesozoic, and their fossils, especially those of the hunting forms with tentacles bearing suckers and hooks, such as phragmoteuthids and belemnites to a lesser extent, became common in the Late Triassic. These forms, modern and ancient, hunted by grasping immobile or slow-moving prey in their tentacles and snipping and crushing the shell with their beak. Combined molecular and fossil evidence (Tanner et al., 2017) confirms massive diversification of such hunting coleoids in the Early Jurassic, but there was slow diversification through the Triassic, with a reported squid-like coleoid from the Early Jurassic of Idaho, United States (Doguzhaeva et al., 2018).
New echinoderm predators emerged. The PTME witnessed the extinction of most echinoderm clades, and Early Triassic fossil records are poor, but they show a rapid re-diversification of ophiuroids and crinoids in the Middle Triassic, and significant expansions of all clades in the Late Triassic (Twitchett and Oji, 2005). Asteroidea (starfishes) are rare as fossils in the Triassic, but these voracious predators likely arose early in the Triassic according to phylogenomic analyses, with the PTME marking the trigger for origin of the most diverse living clade, Neoasteroidea, at that point (Mah and Blake, 2012). Their rarity as fossils may reflect the fact that they require special conditions to fossilize. Cidaroid echinoids diversified substantially from the Carnian onwards, and perhaps drove the evolution of motile crinoids (see below).
Marine predatory vertebrates show spectacular and rapid diversifications in the Early and Middle Triassic, and new discoveries from China have confirmed their early start in the Triassic, but not in the Late Permian (Benton et al., 2013; Scheyer et al., 2014; Motani et al., 2015). Among osteichthyans, the clade Neopterygii expanded enormously in this time, comprising holosteans plus teleosts, and today representing 99% of living bony fishes (Benton et al., 2013; Romano et al., 2016; Xu, 2020; Romano, 2021). Romano (2021) makes a case that there is a hiatus in preservation during the crucial time between Olenekian and early Anisian, from 250 to 246 Ma, so perhaps artificially pushing apparent origins of some clades from Early to Middle Triassic. Similarly, the modern shark clade, Neoselachii, also diversified from the Early Triassic onwards, diversifying into several lineages, but apparently slowly (Cuny and Benton, 1999).
Ambush predation had evolved among fishes in the mid-Paleozoic, but the hugely abundant, elongate osteichthyan Saurichthys apparently adopted new, super sneaky strategies. Computational fluid dynamics studies of 3D models show that Saurichthys had adaptations to make its approach and final lunge undetectable by minimizing flow disturbance, as with modern needlefish and gars (Kogan et al., 2015). According to these authors, pike, which are also ambush hunters, generate more disturbance as they lunge for the kill, but they compensate for this by faster attack speed and their protrusible jaws. Saurichthyid fishes exploded on the scene in the Middle Triassic (Figure 3). They originated in the latest Permian (Eosaurichthys from Changxing, Zhejiang, China) and diversified to dozens of species in the Middle Triassic (Wu et al., 2011, Wu et al., 2015, Wu et al., 2018) and continued until the Middle Jurassic (Maxwell, 2016), hence surviving two major biotic crises. In addition, a lineage of saurichthyiform fishes from the Anisian of China had evolved a distinct crushing feeding mechanism, comprising massive jaws but small and blunt teeth (Wu et al., 2013). These Triassic fishes, however, did not have protrusible jaws, and this important feeding adaptation was absent in early teleosts such as Pholidophorus from the Late Triassic (Arratia, 2017), and is characteristic of the Acanthomorpha (Schaeffer and Rosen, 1961), originating in the Late Jurassic or Early Cretaceous (Chen et al., 2014).
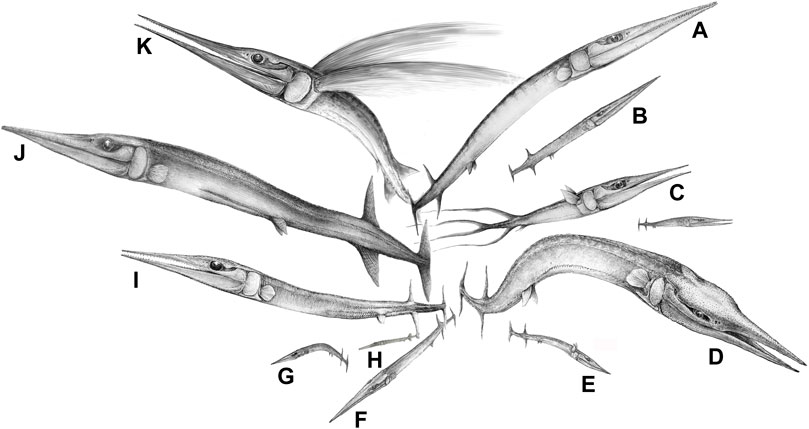
FIGURE 3. The diversification of the saurichthyiform fishes (‘lizard fish’) in the Middle Triassic of South China (eastern paleo-Tethys), reflecting the establishment of a complexly tiered marine ecosystem (or marine fish communities) with intensive predator-prey interactions along the food chains. Species list: (A), Saurichthys dawaziensis; (B), Saurichthys sp. 1 (under description); (C), Sinosaurichthys longimedialis; (D), Yelangichthys macrocephalus; (E), Sinosaurichthys sp. (under description); (F), Saurichthys sp. 2; (G), Sinosaurichthys minuta; (H), Saurichthys spinosa; (I), Saurichthys yunnanensis; (J), Saurichthys yangjuanensis, which preyed on the coexisting Sinosaurichthys longipectoralis (see, Wu et al., 2015); (K), Sinosaurichthys longipectoralis. A,C,E–I from the Anisian of the Luoping Biota; D,J,K from the Anisian of the Panxian Biota; B from the Ladinian of the Xingyi Biota. Artwork by Fei-xiang Wu.
Among marine reptiles, there was substantial diversification in the Early and Middle Triassic, documented especially well in the south of China (Chaohu, Panxian, Luoping, Xingyi, Guanling faunas) and in central Europe (Benton et al., 2013; Scheyer et al., 2014; Motani et al., 2015), including groups such as ichthyosaurs, sauropterygians (pachypleurosaurs, nothosaurs, placodonts), thalattosaurs, saurosphargids, hupehsuchians, archosauromorphs, and chelonians. The recent analysis of a giant ichthyosaur, Cymbospondylus youngorum, from the Anisian-aged Fossil Hill Member of the Favret Formation in Nevada, gives impressive evidence of the rapid diversification of these marine reptiles (Sander et al., 2021). This animal is estimated as 17.6 m long and weighing 45 tons, and the authors carry out detailed macroevolutionary analysis which shows enormously rapid achievement of huge diversity and great body size by ichthyosaurs in the Olenekian and Anisian, a prime example of an ‘early burst’ radiation. They identify 30 species of ichthyosaurs in the Olenekian–Ladinian interval, and explosive evolution of body size from initial sizes of <1 m to a broad range in the Anisian from 0.5 to 17 m, some 5 Myr later. The speed of diversification far outstrips that experienced by whales as they evolved towards large size in the Cenozoic.
The huge body sizes achieved by some ichthyosaur lineages in the Triassic is matched by the fact that many or most of the marine reptiles were probably warm-blooded (endothermic) to some extent, generating internal body heat as mammals and birds do today, in contrast to the largely ectothermic (cold-blooded) modern reptiles. For example, study of bone histology shows that ichthyosaurs were likely homeothermic by virtue of large size and insulation or even regionally endothermic, enabling fast and sustained activity from their origin in the Early Triassic (Nakajima et al., 2014; Lindgren et al., 2018). On the other hand, whereas plesiosaurs from their origination in the Late Triassic may have been endothermic, the Triassic sauropterygians such as nothosaurs, pachypleurosaurs and placodonts, were almost certainly still ectothermic, as indicated by bone histology (Wintrich et al., 2017).
These ichthyosaurs, the other marine reptiles, as well as the sharks and some of the neopterygian bony fishes, were predators, and the larger ichthyosaurs, nothosaurs, thalattosaurs, and hupehsuchians were apex predators, themselves feeding on other marine reptiles. These all represent new predatory levels in the trophic systems of the Olenekian and Anisian, substantially different from the Permian when sharks were the sole top predators (Benton et al., 2013; Scheyer et al., 2014; Sander et al., 2021). The new groups of marine fishes and reptiles occupied other predatory niches too.
Durophagy, the predatory adaptation to crush hard shells, arose several times among Paleozoic predators, for example in placoderms and some sharks (Brett and Walker, 2002), but the habit had disappeared, before its re-emergence among different groups of fishes and newly in reptiles in the Early and Middle Triassic (Tackett, 2016; Tackett and Bottjer, 2016). Shell-crushing vertebrates emerged at the start of the Middle Triassic. Several groups of fishes (hybodontid sharks, colobodontids) and reptiles (placodonts, some ichthyosaurs, some thalattosaurs) had specialized shell-crushing dentitions, and some were relatively large such as 30-cm-long colobodontids (Xu, 2020). Note, however, that although colobontid fishes had blunt teeth on the buccal surface, they had pencil-like teeth on the jaws, which might indicate a predatory habit. Therefore, they were probably not obligate durophages, and may also have preyed on thick-scaled holostean fishes, using the sharp teeth to snatch and manipulate their prey, and the blunt buccal teeth to crush their scales. Importantly also, durophagous fishes and reptiles made up a rather small proportion of the taxic diversity of the Panxian and Luoping (Anisian) marine communities. Linked evidence that shell-crushing occurred in the Middle Triassic vertebrates are regurgitated masses of shell debris and crinoid remains (‘bromalites’) reported by Salamon et al. (2012) from the Middle Triassic of Poland. Several authors have interpreted such evidence as evidence that the MMR began explicitly as a part of the Early–Middle Triassic recovery from the PTME (e.g., Salamon et al., 2012; Benton et al., 2013; Xu, 2020).
Adaptations for durophagy occur also in Triassic hybodont sharks, with typical crushing teeth and body lengths over 2 m in some cases. There may have been some shell-crushing hybodonts in the Permian (Vermeij, 1977), but the Lonchidiidae, which originated in the Middle Triassic, were the first of several Mesozoic families of hybodonts to adopt durophagy. In Jurassic species, there are also cases of stomach contents and regurgitations of belemnites (Klug et al., 2021).
Durophagy in ichthyosaurs evolved 3–5 times during the Triassic, and indeed more than half the species of Early and Middle Triassic ichthyosaurs had at least some molariform teeth (Huang et al., 2020). Indeed, Huang et al. (2020) suggest that the prevalence of durophagy among these marine reptiles indicates that their specific adaptations to consuming hard-shelled prey and hard-scaled fishes explains much of their early success. Further, the nature of the crushing teeth varies, suggesting that different ichthyosaurs specialized on different kinds of hard-shelled prey. All the durophagous reptiles (placodonts, ichthyosaurs, thalattosaurs) died out at the end of the Triassic, or earlier, and the habit did not re-emerge in marine reptiles until the Late Jurassic, in plesiochelyid turtles, and Late Cretaceous, in some mosasauroids and turtles (Stubbs and Benton, 2016).
New Defenses and Evasions
The new predators and their cunning modes of attack in Triassic oceans stimulated new defensive adaptations in their prey (Figure 2). In an earlier review, McRoberts (2001) found that Mesozoic predatory adaptations such as durophagy, shell snipping, and shell boring were present, but not prevalent in the Triassic. He noted steadily rising diversity among bivalves through the Triassic but explained the series of innovations just noted driven by environmental changes associated with the recovery of life following the PTME, rather than evidence for an escalation of predator-prey interactions, a key element of the MMR, mainly because of the apparent rarity of the new predatory groups and evidence for their activities such as boreholes and snipped shell edges. This view was strengthened by reports of predatory attack on mollusks in the Early Jurassic and Late Triassic. Harper et al. (1998) suggested a Triassic start for the MMR based on evidence for high levels of boring predation marks on bivalve shells from the Lower Jurassic.
Shell drilling was not a Mesozoic innovation, having been reported through the Paleozoic, and attributed to platyceratid gastropods (Brett and Walker, 2002). However, the drilling predation habit was not continuous through geological time, with a gap from Late Carboniferous to Permian, and a gradual increase from the Middle Triassic to the present day (Walker and Brett, 2002; Klompmaker et al., 2016; Tackett and Tintori, 2019, Figure 5). Fürsich and Jablonski (1984) reported extensive findings of boreholes from the Triassic, evidence of the new gastropod predation modes. Strong external shell sculptures, interpreted as an antipredatory feature, and typical of the MMR (Vermeij, 1977), were also reported in Triassic gastropods and bivalves (Nützel, 2002). The relatively low levels of boreholes in the Triassic are indicative of the re-invention of this feeding strategy in the Early to Middle Triassic, and its slow expansion through the Jurassic and Early Cretaceous (0–5%), until it rose to modern levels (c. 20%) in the Late Cretaceous when there was a great increase in drilling by naticid and muricid gastropods (Harper, 2003).
Trace fossils provide additional evidence for an escalation in predator-prey behavior in the Triassic. Previous authors noted an increase in infaunalization in the Early Triassic (Stanley, 1968, 1977; Foster and Twitchett, 2014). What we cannot demonstrate is whether this behavior was new and was not already happening in the Late Permian. Buatois et al. (2016) report that trace fossils show evidence that seabed ecosystems had fully recovered by the Middle Triassic in equatorial carbonate settings, although these ichnoassemblages show limited infaunalization and simple tiering structures. These authors note major changes in Jurassic ichnoassemblages that mark the rise to dominance of the Modern Evolutionary Fauna. In general, the intensity of bioturbation, trace fossil diversity, complexity of tiering, and maximum burrow depths, increased through the Early and Middle Triassic, indicating stepwise recovery of benthic biodiversity and range of feeding modes (Luo et al., 2020). Bioerosive herbivory, whereby mollusks, echinoids and other taxa feed actively across sea-shore services, consuming green algae, began in the Rhaetian and became common in the Jurassic (Radley, 2010), and it is unclear whether older examples are confidently known in the Triassic.
Cementing to rocks and clamping tight shut are mechanisms that oysters and other mollusks use to avoid the attentions of predators. Hautmann (2004) reported the origin of a major antipredatory adaptation in Triassic bivalves coupled with substantial diversification of the major clade Pteriomorpha, which includes mussels, scallops, and oysters. Pteriomorph bivalves are characterized by modified alivincular ligaments that substantially strengthen the hinge line and enable them to adopt one of two new defense strategies; oysters and mussels can clamp down and keep the shell shut against attempts to prize it open by starfishes, and scallops and other swimmers can close the shell fast under attack and escape by snapping shut and squirting water out. Hautmann (2004) noted that three modified alivincular ligament types emerged in parallel in different pteriomorph clades in the Late Triassic and Early Jurassic. Hautmann et al. (2017) reported Early and Middle Triassic cementing oysters, and their origin and diversification early in the Triassic has been explained as paralleling the diversification of durophagous crustaceans, fishes and reptiles.
The alivincular ligament, as noted, enables scallops and relatives to snap the valves shut and escape by rocket propulsion. Hautmann (2010) reported the first scallop from the early Ladinian of Europe. Further, phylogenetic analyses of the pecten families Pectinidae and Entolioididae show they originated in the Early Triassic, likely with their shell-clapping habit and strengthened alivincular ligament from the start (Waller, 2006).
Escape by fast swimming has also been suggested as evidence of new predation pressures on crinoids. Motility in stalked crinoids perhaps evolved for similar reasons. Baumiller et al. (2010) noted evidence for active predation by cidaroid echinoids on stalked crinoids from the Middle Triassic of Poland, as well as in later stratigraphic units, and suggested that this new mode of hunting promoted the evolution of motile crinoids as a means of escape from their fellow echinoderms. Cidaroid echinoids diversified through the Triassic and Early Jurassic (Kier, 1974), and their predation on crinoids perhaps drove this new mode of life.
An unexpected discovery was the first flying fish, from the late Ladinian of China (Figure 2) (Xu et al., 2013). This was a locomotory mode that had been identified in the Late Triassic thoracopterid bony fishes (Tintori and Sassi, 1992), and it is a convergent precursor of the Exocetidae (Beloniformes), the flying fishes of today. Fish fly, by launching themselves out of the water, as an escape strategy, but so far as we know, this mode of escape from predation did not exist in the Jurassic or Early Cretaceous, until Exocoetoides (?Aulopiformes, Chirothricidae) evolved in the Late Cretaceous (Davis, 1887).
Two Phases in the Triassic MMR
These studies of evolution among potential predators tend to show earlier-than-expected origins of many clades, and some of them, such as ichthyosaurs, apparently diversified explosively in the first 5–10 Myr of the Triassic. Others show an early origin of the clade or the innovation, but a slow expansion, and sometimes an uptick in the Carnian or Norian, in many cases following the CPE (Figure 2). There is a similar divergence of evidence for patterns of new modes of predation, as well as predation avoidance and escape strategies, with some modes occurring very early in the Triassic, and others expanding slowly and spreading more rapidly in the Late Triassic to Early Jurassic.
There are two reasons for a coincidence between the processes of recovery of life from the extreme levels of devastation wrought by the PTME, and the beginning of the MMR and origin of modern marine ecosystems. First is that the extinction cleared ecospace and allowed new taxa to dominate earliest Triassic ecosystems, and these new taxa established new, faster life modes and arms races than seen in the Paleozoic. Second are the more immediate aspects of the turmoil of post-PTME seas, when harsh environmental conditions interfered with the recovery and forced some strong ecological interactions. During the Early and Middle Triassic, new clades with their new adaptations emerged, both new antipredatory strategies such as thickened shells and cementation in oysters and mussels, snap escape swimming by scallops, motile crinoids, prominent sculpture by gastropods and bivalves, and deep burrowing by many taxa, as well as the new hunting modes, including shell snipping by malacostracans, hole boring using chemical and mechanical means by gastropods, and durophagy by hybodont sharks and reptiles.
The strong evidence for substantial expansion among invertebrate predatory clades, as well as neoselachian sharks, durophagous fishes and reptiles, and evidence of predatory activity and anti-predatory adaptations (motile crinoids; heavily ridged shells; cemented oysters; shell-clapping scallops) from the Carnian onwards could reflect bias in the fossil record, namely the availability of numerous extensively fossiliferous marine geological formations in Europe, North America, China and elsewhere that preserve more fossils and to higher quality. However, some of the apparent explosion of new clades and innovations, especially for example the diversification of scleractinian corals and coral reefs, and the plankton revolution, are likely real, and consequences of the impact of the CPE (Dal Corso et al., 2020). The plankton revolution of the Late Triassic (Falkowski et al., 2004) was the time when all modern marine plankton (calcareous nannoplankton, planktonic foraminifera, dinoflagellates, radiolaria) originated or diversified. These provided the basis for new trophic structures in the oceans and could have triggered the explosive diversification of consumers at all levels.
In summary, new evidence from marine ecosystems has shown that the initiation of the MMR has moved back in steps through the last decades. At first, Vermeij (1977) suggested that the major escalation in predator-prey interaction began in the Late Jurassic, but then marshalled evidence that the first phase actually dated back to the Late Triassic-Early Jurassic (Vermeij, 2008). New evidence, as noted by many authors and summarized here, is that the initiation of the MMR coincides with the building of the ‘Modern’ marine ecosystem from the Early Triassic onwards (Sepkoski, 1984), and that there was a second escalation following the CPE some 232 Ma, marked by major revolutions in plankton, carbonate factory, predatory modes, and anti-predator defenses (Dal Corso et al., 2020).
Further evidence for escalation through the Triassic is that some of the new predatory and defensive adaptations disappeared at the end of the Triassic, including widespread durophagy in marine reptiles and flying adaptations in fishes; these adaptations re-emerged in other groups later in the Mesozoic.
The Triassic Terrestrial Revolution
Physiological Escalation
Biologists, and the general public, readily divide vertebrates into ‘cold-blooded’ fishes, amphibians and reptiles on the one hand, and ‘warm-blooded’ mammals and birds on the other. It is broadly assumed that these represent respectively low-energy and high-energy modes of life, or ‘slow’ and ‘fast’ organisms in terms of the speed with which they live their lives, but also the amount of energy, oxygen and carbon they consume. Mammals and birds are fast-moving, capable of sustained locomotion and nocturnality, whereas amphibians and reptiles are generally slower moving, not capable of sustained fast movement, and apparently motionless for much of the time. In fact, there may be a twenty-fold difference in energy consumption between the two physiological groups. Metabolic rate, measured as the rate of consumption of oxygen, depends on the size of the animal (it is relatively higher in small animals), but as an example a small bird may use up 10 ml of oxygen in 1.3 h, whereas a lizard of similar body mass would take 23.8 h to use the same amount of oxygen, a 20-fold difference (Bennett and Nagy, 1977; Gillooly et al., 2001).
This reflects fundamental differences between endothermy (‘warm-bloodedness’; production of heat by physiological means) and ectothermy (‘cold-bloodedness’; acquisition of heat from external environmental sources): endotherms consume oxygen and food in considerable quantities to fuel their inner furnaces, whereas ectotherms rely on sunlight directly or indirectly. At larger body sizes, differences in metabolic rate may become less because bulky ectotherms achieve insulation by being large and so retain heat passively when the external environment cools down (McNab, 1978; Paladino et al., 1990). This enables some large living reptiles such as sea turtles and some snakes to achieve a kind of warm-bloodedness, termed gigantothermy or inertial homeothermy, possibly also true for dinosaurs and other extinct reptiles including ichthyosaurs and plesiosaurs (Paladino et al., 1990; Seymour, 2013; Lindgren et al., 2018).
However, it is not clear that inertial endothermy is necessarily equivalent to endothermy in terms of metabolic rate and outputs: Seymour (2013) found that whereas a small crocodilian (1 kg) can produce up to 57% of the power output of a mammal of similar body mass, a large crocodile (200 kg) with relatively inertially constant body temperature nonetheless produced only 14% of the power of a mammal of similar body mass. The deficit relates to the relative aerobic capacity of endotherms and ectotherms, their ability to take in and use oxygen in a continuous manner.
This difference is made more marked by posture. Lizards and most ectothermic tetrapods are sprawlers that move by swinging the vertebral column from side to side as they take strides. As the right arm moves forward, the torso twists left and air is expelled from the left to the right lung, and vice versa as the left arm moves forward. Mammals and birds have erect, or parasagittal, posture with the limbs held beneath the body and moving back and forwards parallel to the backbone. Mammal backbones flex up and down and air is pumped in and out of the lungs by compression and relaxation of the diaphragm; birds have a one-way respiration system involving air sacs connected to the lungs. Both groups show high endurance when compared to ectothermic tetrapods; salamanders and lizards can either run or breathe, but not both (Carrier, 1987).
Benton (2021) argued that the Triassic was the crucial time in the independent origins of endothermy in the bird and mammal lineages. He pointed to the origin of parasagittal gait and insulating dermal coverings in the Early and Middle Triassic, 250 Ma, and speculated that this happened during the time when life was recovering from the PTME. Then all aspects of a higher-paced, endothermic lifestyle became established in archosaurs and synapsids as they engaged in competitive and predator-prey arms races throughout the Triassic. Can this case be sustained in the face of very different models for the origins of endothermy?
Origins of Endothermy
The origins of endothermy in mammals and birds have long been discussed, and current estimates of timing range widely, from Carboniferous to Jurassic. Grigg et al. (2022) argued that endothermy in both clades should be traced back to the origin of amniotes in the mid-Carboniferous, some 310 Ma, based on a combination of the close similarities of the fundamentals of avian and mammalian endothermy, as well as paleontological evidence. On the other hand, Rezende et al. (2020) argued that endothermy emerged in birds only with the extreme miniaturization that took place in the paravian lineage in the Middle and Late Jurassic, 175 Ma. Similarly, Newham et al. (2022) identify a late origin of endothermy in mammals also in the Middle Jurassic.
Grigg et al. (2022) argue for a single origin of endothermy in early amniotes from physiological and paleontological evidence. First, they note revisions to the standard assumption that non-shivering thermogenesis (NST), the fundamental source of tetrapod endothermy, originates from skeletal muscle in birds and brown adipose tissue in mammals; in fact, they note that brown adipose tissue is absent in monotremes, marsupials and many eutherian mammals, and that muscle-based NST in birds and mammals shares the same processes of calcium transfer at cellular level. Second, they marshal paleontological evidence for an early, shared origin of whole-body endothermy in all amniotes, and as seen today in birds and mammals: 1) evidence from bone histology for fibrolamellar bone and endothermy in Carboniferous and Permian amniotes including ophiacodontids, edaphosaurids, sphenacodontians, dinocephalians, gorgonopsians, dicynodonts, and pareiasaurs; 2) erect or semi-erect posture and large body size in some Permian synapsids (Dimetrodon, Inostrancevia, Moschops) and sauropsids (Scutosaurus, Moradisaurus); 3) large nutrient foramina in long bones of caseids, edaphosaurids, sphenacodontids, dicynodonts, gorgonopsids, and cynodonts suggesting rapid growth and repair associated with high-speed locomotion; and 4) obligate bipedality in Triassic archosaurs. If whole-body endothermy arose soon after the origin of amniotes in the mid-Carboniferous and persisted in all clades including the bird and mammal lineages, the ancestors of turtles, lizards, snakes, and crocodilians must have reverted from endothermy to ectothermy independently at certain points in their histories. Indeed, it has often been suggested that crocodilians were ancestrally endothermic based on their 4-chambered hearts, bird-like lungs, and fibrolamellar bone in juveniles (Seymour et al., 2004). However, the proposal (Grigg et al., 2022) of reversion from endothermy to ectothermy in the ancestors of turtles and lepidosaurs (lizard snakes and relatives) is novel.
At the other end of the scale, as noted, some recent papers propose that avian and mammalian endothermy arose 135 Myr later, in the Middle Jurassic. Rezende et al. (2020) argued that endothermy arose in the bird lineage at the same time as miniaturization brought their body size down to a point where the costs of elevated energy requirements were mitigated. They argue that the cost of maintaining body temperature higher than ambient (= environmental) temperature is high in a large animal, so miniaturization brings the cost down. Their calculations suggest that the transition from ectothermy to endothermy happened between body masses of 100 and 1 kg, and especially at about 10 kg, so they suggest that many maniraptorans, and all paravians were endothermic. This transition occurred in the Early to Middle Jurassic, about 180–170 Ma, well before the origin of birds, and associated with the acquisition of diverse avian-like feather types by numerous maniraptoran dinosaurian lineages.
Similarly, Newham et al. (2022) point to the Middle Jurassic as the time of origin of endothermy in mammals, long after the emergence of indicators accepted by other authors (e.g., Botha-Brink et al., 2018; Benton, 2021) as evidence for earlier origins, whether in the Middle or Late Permian (separation of lumbar and thoracic regions and origin of diaphragm; differentiation of dentition; bony secondary palate; enlargement of brain), Early Triassic (parasagittal gait; nutrient foramina on snout suggesting muscular lips and hair; parental care; fibrolamellar bone; reduced red blood cell size), or Late Triassic (neurosensory evolution and hair; miniaturization on mammalian lineage; ossified nasal turbinates). Newham et al. (2022) acknowledge all these features and their relative timings of origin but argue that they indicate modifications to locomotory mode and enhanced aerobic activity, but not endothermy. They emphasize the importance of the acquisition of both a high basal metabolic rate (BMR) and a high maximum metabolic rate (MaMR), both of which are features of modern mammals. Their studies of the Early Jurassic mammal Morganucodon show that, although it had all these features, as well as the mammalian jaw joint, diphyodonty (tooth replacement limited to milk and adult sets), and determinate growth, growth increments in the cement that fixed the teeth in their sockets indicated a life span of 14 years, and 9 years for another Early Jurassic mammal, Kuehneotherium. Such long life spans are more in line with ectotherms than endotherms of such small body sizes. Further, the sizes of nutrient foramina in the limb bones of Morganucodon indicate a MaMR value closer to that of modern reptiles than mammals.
In his overview of the evolution of endothermy, Lovegrove (2017) suggested a three-phase model in both birds and mammals, which each followed independently, through stages of 1) parental care and locomotion; 2) thermoregulation and miniaturization; and 3) locomotion and climate adaptation. In the bird lineage (Archosauria), these phases ran respectively from Late Permian–Triassic; Jurassic–Early Cretaceous; and Early Cretaceous–Modern, and in the mammal lineage (Synapsida) from Middle Permian–Middle Triassic; Late Triassic–Paleogene; and Paleogene–Modern. Newham et al. (2022) present a similar sequence for mammals, with initial stages of increasing aerobic capacity (MMR) and improving thermoregulatory ability (BMR) through the Permian and Triassic, and the establishment of endothermy (acquisition of mammalian BMR and MaMR) in the Late Jurassic and Cretaceous, coincident with the origin of modern mammals.
Triassic Arms Races
Whether we accept origins of endothermy in the Carboniferous, Permian, Triassic or Jurassic, it is worth considering steps along the way and when they happened. In fact, much of the debate outlined above depends on definitions of endothermy, but despite quandaries around that issue, we can observe many definite changes in the fossils, including origins of erect posture, dermal insulation, and elevated metabolic rates as inferred from bone histology from the Early Triassic onwards. Importantly, the changes appear to occur in parallel in the two major clades of terrestrial tetrapods of the time, the archosauromorphs (including ancestors of crocodilians, dinosaurs and birds) and synapsids (including ancestors of mammals).
These two great clades passed at low diversity into the Triassic. Archosauromorphs had existed only at low diversity in the Late Permian, and synapsids were hit hard by the PTME, and perhaps only ten or twenty species survived (Benton et al., 2004; Irmis and Whiteside, 2011). The earliest Triassic archosauromorphs and synapsids diversified rapidly in the new world, and they appear to have embedded a series of new adaptations that spread through all Triassic survivors and their descendants.
First is Posture
It had long been noted that Late Permian synapsids showed substantial modifications in their posture with the limbs tucked beneath the body and modified rib cages suggesting the presence of a diaphragm, and a vertebral column that flexed up and down rather than side to side (e.g., Jones et al., 2019). Among archosauromorphs, or archosaurs in particular, a different pattern of postural evolution had been suggested, passing stepwise from a sprawling posture to semi-erect, and then fully erect by Middle and Late Triassic (Charig, 1972). This idea of a gradual postural shift was challenged by Kubo and Benton (2009) who showed an apparently instant switch in the posture of medium-sized tetrapods that coincided with the Permian-Triassic boundary (Figure 4). Using a large sample of fossil trackways, they showed that all the Middle and Late Permian tracks suggested sprawling locomotion, whereas from the Early Triassic onwards, the postures were parasagittal. This can be determined readily from fossil trackways by measuring the pace angulation (the angle between successive right-left-right footprint sets): values were 88o in the Middle and Late Permian, but 140o, 149o, and 156o in the Early, Middle and Late Triassic. This documents a shift from very widely sprawling reptiles in the Permian to purely parasagittal forms in the Triassic, and showing some, but not much, evolution of parasagittality.
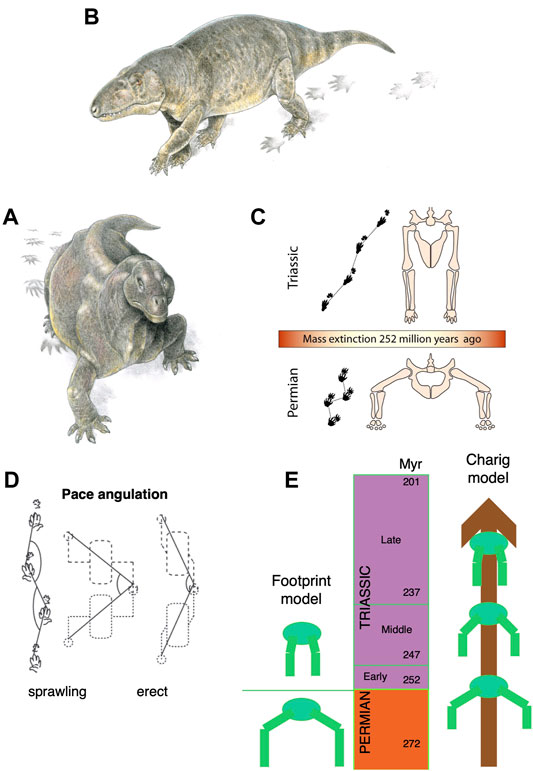
FIGURE 4. Posture shift from sprawling to parasagittal, among synapsids and archosauromorphs. (A) A sprawling synapsid, Moschops from the Late Permian. (B) An erect-postured archosauriform, Vjushkovia from the Early and Middle Triassic, both with tracks. (C) The transition from sprawling to erect posture across the Permian-Triassic boundary. (D) Measurement of pace angulation of tracks, showing sprawling and erect tracks, with sketch body and limbs (values of pace angulation 138o indicate an erect posture). (E) Contrast of the instant switch from sprawling to erect posture across the Permian-Triassic boundary, 252 Ma (left) with the classic Charig (1972) model of a more prolonged postural shift, occurring through much of the Triassic. Panels A and B are by Jim Robins; C by Simon Powell; D is from Kubo and Benton (2009), and E is original; all from Benton (2021).
This simple switch from sprawling to erect posture across the PTB has been challenged by Sullivan (2015) who notes that the skeletons of Early Triassic archosaurs such as proterosuchids and erythrosuchids show they were almost certainly sprawlers. This apparent mismatch of footprint and skeletal evidence requires more study, but either way an erect posture was the norm for archosaurs and synapsids by the Middle Triassic.
Second is Dermal Insulation
A common assumption by physiologists is that the feathers of birds and hair of mammals are correlated with their endothermy and that they act as insulation. Therefore, if such insulating pelage were identified in the fossils, this might also suggest warm-bloodedness, if not endothermy of a modern kind. Such conditions had been suggested by Watson (1913, 1931) based on abundant pits around the snout in cynodonts and therocephalians, carnivorous synapsids: he argued that the pits (Figure 5A) were presumably for nerves that invested the sensory whiskers, or vibrissae, and that these early meat-eaters also had muscular mammalian-like lips, as well as upright posture and enlarged brains, all indicative of endothermy. Fossil hairs have been identified in Late Permian coprolites from South Africa (Smith and Botha-Brink, 2011) and Russia (Bajdek et al., 2016). Nevertheless, Botha-Brink et al. (2018) suggest that hair evolved, or at least formed an insulating pelage, in synapsids in the early Late Triassic. It now seems evident that archosauromorphs also had feathers, especially short, fluffy insulating-type feathers, since the Early Triassic. The evidence is that theropod dinosaurs have very clearly avian-like feathers (Xu et al., 2014), as do some ornithischian dinosaurs such as Kulindadromeus. If dinosaurs all have feathers or the propensity for feathers, then feathers would date to the origin of dinosaurs (Benton et al., 2019; Yang et al., 2019). Pterosaurs, the sister group of dinosaurs, also have dermal insulating structures commonly called pycnofibres. New evidence (Yang et al., 2019; Cincotta et al., 2022) shows these pycnofibres take a variety of forms, including branching and tufting structures as seen among dinosaurs and birds (Figures 5B,C), so these are presumably also feathers. However, even if researchers balk at calling pycnofibres feathers, it does not change the fact that insulating dermal structures appeared in the first dinosaurs and the first pterosaurs, and the shared ancestry of these two clades is dated to the Early or early Middle Triassic. This realization of such early origins is a new discovery based on the occurrence of sister-group taxa such as silesaurids and aphanosaurians in the Anisian (Benton et al., 2014; Nesbitt et al., 2017; Benton, 2021).
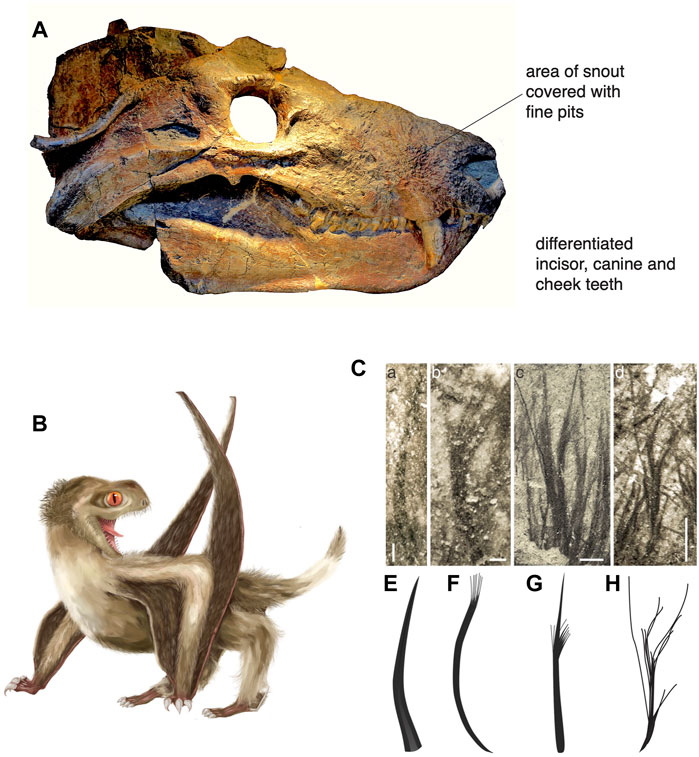
FIGURE 5. Evidence for insulating pelage in synapsids (A) and archosauromorphs (B,C). (A) Skull of the Middle Triassic cynodont Cynognathus in lateral view, showing pits on the snout suggesting vibrissae. (B) An anurognathid pterosaur from the Middle Jurassic of China, from which the four feather types were identified. (C) The four pterosaurian feather types: monofilaments (a, e), tufted monofilaments (b, f), bunched fibres (c, g), and down feathers (d, h). (A) Photo by Emöke Dénes, Wikimedia; (B) artwork by Yuan Zhang; (C) Image based on Yang et al. (2019).
According to an alternative view (Ezcurra et al., 2020a), the key Manda Formation of Tanzania is redated as late Ladinian to early Carnian rather than mid to late Anisian, so moving the dates of origin of some key early avemetatarsalians forward in time. However, even if the ages of these early avemetatarsalians are much younger than generally assumed, there are definitive members of the sister clade of Avemetatarsalia, the Pseudosuchia, in the Olenekian (Butler et al., 2011), so dating the origin of both clades as minimally Early Triassic (Olenekian). According to current phylogenies (e.g., Nesbitt et al., 2017; Ezcurra et al., 2020b), Pterosauria and sister clades and Dinosauria and sister clades each form unique lineages that diverged at the origin of Avemetatarsalia, so we assume an Early Triassic origin for both. One aphanosaurian, Dongusuchus from the Donguz Svita of Russia, is almost certainly Anisian in age, and if correctly identified as an aphanosaurian (Nesbitt et al., 2017), this brings the origins of the dinosaur and pterosaur lineages down to middle Anisian, if not late Olenekian, whatever the age of the Manda Formation.
Third is evidence of high metabolic rate in the bone histology of Triassic archosauromorphs and synapsids. Investigators have noted the widespread presence of fibrolamellar bone, often with extensive secondary remodeling through Haversian canals, both of which are features of the bone of living endotherms such as birds and mammals that exhibit fast growth (e.g., Ricqlès et al., 2008; Botha-Brink et al., 2018). Importantly, the sizes of canals through bone can indicate metabolic rate: birds and mammals today have small red blood cells (RBC) to enable the transport of increased amounts of oxygen when compared to ectotherms such as amphibians or reptiles which have larger red blood cells. RBC size then is a measure of endothermy vs. ectothermy and, although the red blood cells are not preserved, the scaling of bone microvascularization provides a good measure. Huttenlocker and Farmer (2017) showed that Triassic synapsids and archosauromorphs generally had RBC sizes <10 μm and many were <7 μm, suggesting coincident evolution of endothermy in both lineages from the Early Triassic onwards (Figure 6). Microvessel size is only one direct anatomical indicator of thermal physiology in the bone of fossil tetrapods; other indicators include vascular density, osteocyte density, osteocyte shape, and osteocyte area, and these were used in combination by Legendre et al. (2016) to estimate resting metabolic rate (RMR) of a wide variety of Permian and Mesozoic animals (Figure 6). Their multi-factor histological metric is calibrated from modern reptiles, birds and mammals for which the RMR is known, and values are then determined for fossil forms. Their calibrated ancestral states reconstruction (Figure 7) indicates widespread endothermy (yellow-green, yellow, red colours) in both Triassic archosauromorphs and synapsids. A similar phylogenetic study, showing more detail of Synapsida and a broad array of traits (Figure 8) confirms stepwise acquisition of derived features through the Triassic (Botha-Brink et al., 2018).
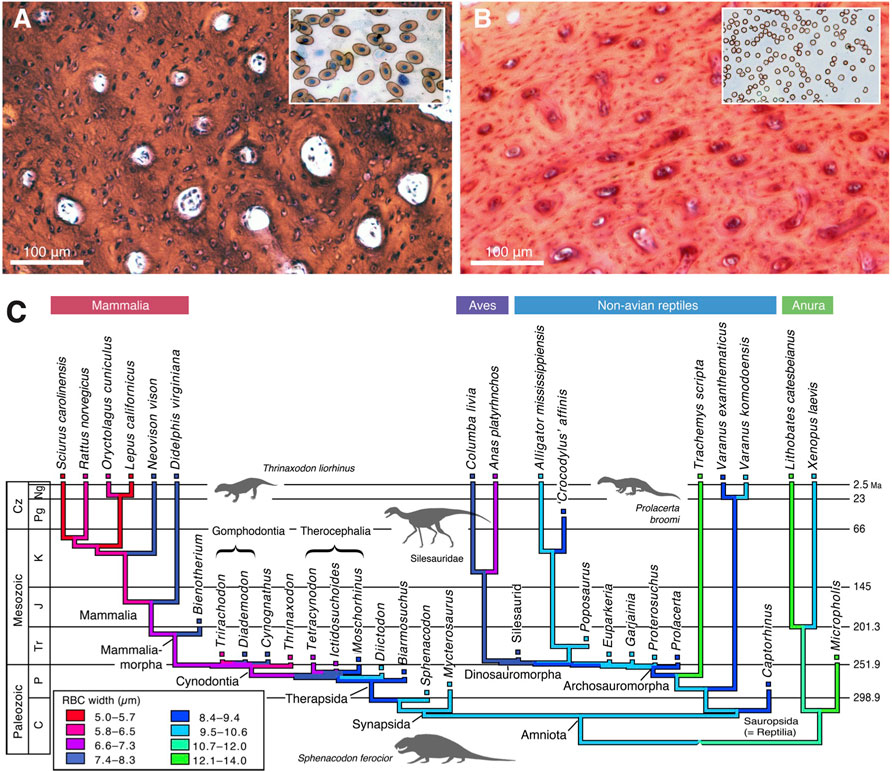
FIGURE 6. Bone microvascularization and red blood diameter. (A,B) Microphotographs of histological sections of limb bones of American bullfrog (A) and rabbit (VB), with diagrammatic version to show larger vessels and fewer of them in the ectotherm (A) than in the endotherm (B). (C) Phylogenetic tree showing optimisation of ancestral states reconstruction of red blood cell size (based on bone microvascularization) for tetrapods; among synapsids, therocephalians and cynodonts show mammal-like red blood cell sizes by the Permian-Triassic transition, whereas Triassic archosaurs show a mix of modern bird and crocodilian values in the Triassic. All images courtesy of Adam Huttenlocker.
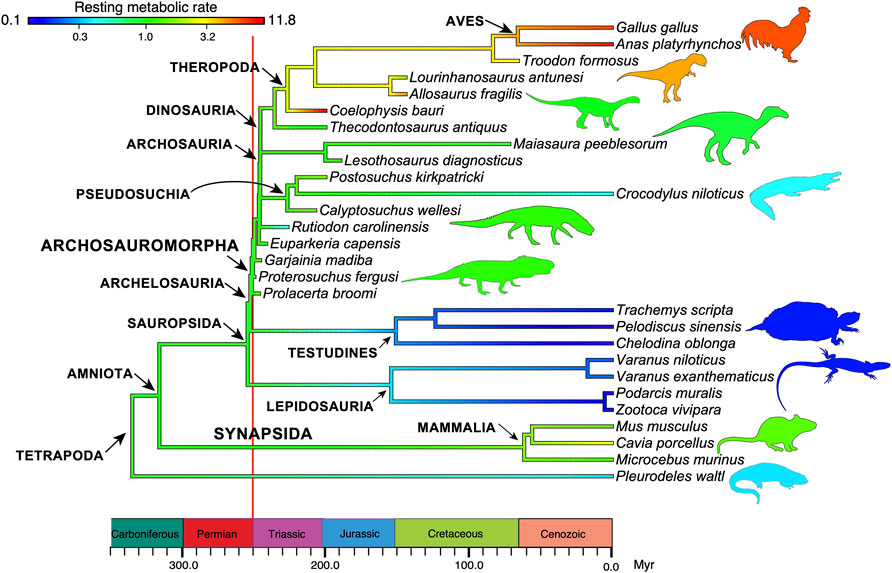
FIGURE 7. The evolution of endothermy in archosauromorphs and synapsids from the Permian to the present day. In the analysis, ancestral states of resting metabolic rates, measured in mLO2h−1 g−0.67, are estimated at each branching point in the phylogeny and color-coded to indicate the level (note the logarithmic scale). Values for ectotherms are typically 1.0, with modern mammals having RMR values in the range 1.5–3.5, and modern birds, 8–12 mLO2h−1 g−0.67. Based on data in Legendre et al. (2016), with thanks to Lucas Legendre for the base image.
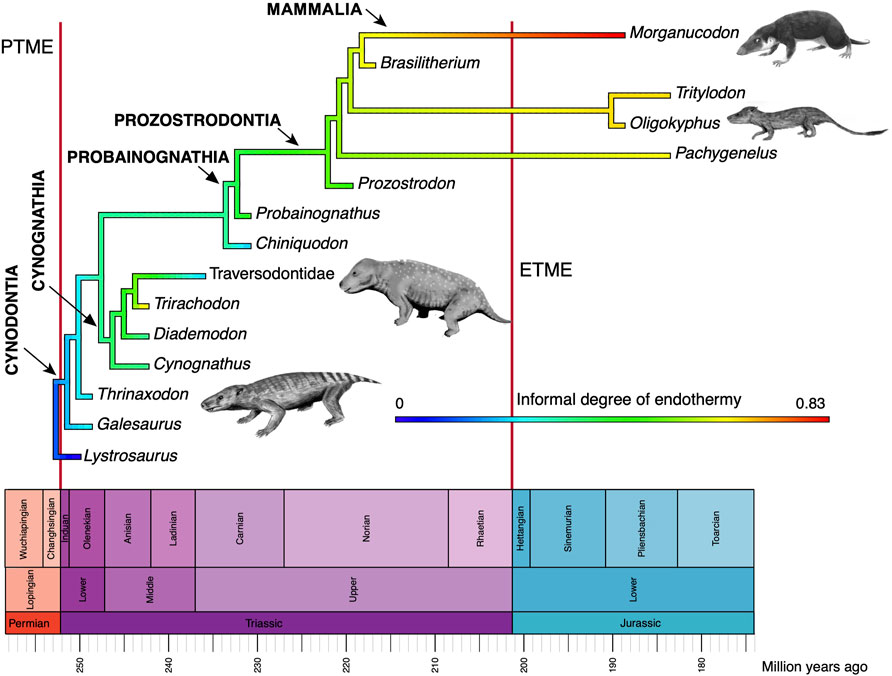
FIGURE 8. Evolution of endothermy among synapsids, showing a range of values of an informal metric of endothermy (data in Benton, 2021, table 1). The small animal images are by Nobu Tamura (Thrinaxodon, Oligokyphus, Exaeretodon) and FunkMonk (Morganucodon), all Creative Commons (Wikimedia).
The conclusion from studies of posture, insulatory structures and bone histology is that both archosauromorphs and synapsids were evolving a broad array of structures indicative of more active lifestyles, even endothermy throughout the Triassic (Figure 9). In their ecosystems, both lineages included a broad diversity of herbivores and carnivores of various sizes, and they were living side by side, presumably competing for food and engaging in predator-prey relationships, where sometimes the top predators were archosauromorphs, sometimes synapsids. Overall then these are the lines of evidence that led Benton (2021) to argue for side-by-side evolution of indicators of enhanced metabolic rates in both lineages and interpreted these ecologically as arms races through the Triassic that culminated in dinosaurs and pterosaurs on one hand, mammals on the other.
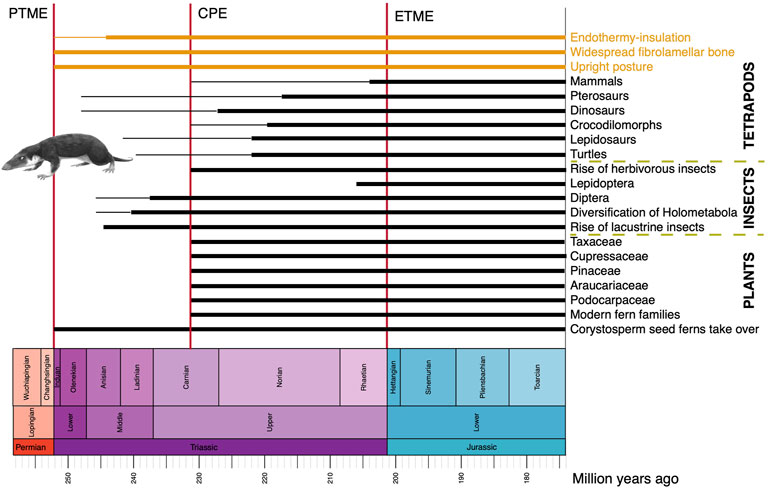
FIGURE 9. Novel physiological and functional characteristics, new tetrapod, insect and plant groups in the Triassic on land. As in Figure 2, the image shows approximate timings of known fossil records, or times when a phenomenon became established (thick line) and earlier occurrences, either inferred from phylogeny or based on rare fossils (thin line). The early mammal Morganucodon is by FunkMunk (Wikimedia).
In all cases for terrestrial tetrapods, steps in their evolution from Paleozoic holdover taxa in the Early Triassic to modern-style animals by the end of the Triassic, were mediated by major events, most notably the CPE (Bernardi et al., 2018; Dal Corso et al., 2020). For dinosaurs and pterosaurs, it seems that both clades originated in the late Early or early Middle Triassic, but fossils of unequivocal examples are not known until the Late Triassic, after the CPE. By this time, with major changes in climates and floras, other ‘modern’ tetrapods are encountered at this time, including lizards, rhynchocephalians, turtles, crocodylomorphs, and mammals. Perhaps then the combination of global clear-out and reset by the PTME, followed by the CPE, triggered the birth of modern-style ecosystems on land as well as in the oceans (Dal Corso et al., 2020).
Plants and Insects
The fossil record of other terrestrial organisms has not been explored as thoroughly as that of tetrapods, but plants and insects also appear to show major steps towards modernization of ecosystems through the Triassic, and especially following the CPE (Figure 9). Plants suffered a major setback at the beginning of the Triassic because the PTME wiped out large sectors of the plant communities, most notably trees. The famous Early to Middle Triassic ‘coal gap’ marks a time of 10–20 Myr when trees and forests were absent. In Gondwana, corystosperm seed ferns dominated floras, and forests became diverse and productive during the humid conditions of the CPE, marking the return of substantial coal seams. Numerous plant groups that were to be important components of Mesozoic floras emerged and diversified at or after the CPE (Dal Corso et al., 2020; Lu et al., 2021), including the Bennettitales and several modern fern families (Hymenophyllaceae, Matoniaceae, Dipteridaceae). Probably more significant in the landscape were the diversifying new conifer groups, adapted to post-CPE arid conditions, including Podocarpaceae (podocarps), Araucariaceae (monkey puzzle trees), Pinaceae (pines, cedars, firs), Cupressaceae (cypresses), Taxaceae (yews), and the extinct Cheirolepidiaceae. The importance of this phase is also that these represent by far the majority of modern conifers, all of which trace their origins to this time in the Late Triassic.
The impact of the PTME on insects has been hard to determine because of patchy occurrences, but there were high rates of extinction at the end of the Permian and high rates of origination in the Early Triassic (Nicholson et al., 2014). Family-level richness of insects began to increase steadily towards the present from the Triassic onwards, and apparently with a marked increase in rates in the Carnian (Nicholson et al., 2015). New evidence from China (Zheng et al., 2018) confirms that lacustrine insects diversified substantially in the Middle Triassic, part of the ‘Mesozoic Lacustrine Revolution’, with the earliest caddisfly cases (Trichoptera), water boatmen (Hemiptera), diverse polyphagan beetles (Coleoptera), and scorpionflies (Mecoptera). The clade Holometabola, comprising 95% modern insect species, diversified substantially in the Middle to Late Triassic, perhaps because of their resilience and buffering from environmental variability through developmental and ecological adaptations, coupled with the diversification of new plant groups as life recovered from the impacts of the PTME (Wang et al., 2022). Among major new insect groups originating in the Triassic are Diptera (flies), first known from the early Middle Triassic (Lukashevich, 2021) and Lepidoptera (moths and butterflies), first known from the Late Triassic (van Eldijk et al., 2018). Modern herbivorous insects diversified substantially in the Late Triassic after the CPE, including aquatic insects, hydraphagans (water beetles), and staphylinids (rove beetles), and many modern feeding modes (piercing and sucking, galling, leaf mining, seed predation) seem to have diversified in the Carnian (Dal Corso et al., 2020).
Conclusion
When Van Valen (1984) identified the PTME as the pivotal turning point in the history of Phanerozoic life, and when Sepkoski (1984) noted the origin of the ‘Modern’ marine evolutionary fauna in the Triassic, both authors stressed the revolutionary nature of the Triassic for the evolution of life in the sea. New work has confirmed their insights, in particular moving the MMR back in time to coincide with recovery from catastrophe through the Early and Middle Triassic. New work on Triassic dinosaurs and other terrestrial tetrapods has confirmed the critical role of the Triassic also in the origins of modern terrestrial ecosystems. Most notably, several lines of evidence (posture, insulation, bone histology) point to widespread elevated metabolic rates in both main tetrapod lineages, the archosauromorphs and synapsids. The endothermy of modern birds and mammals began to evolve in the Early to Middle Triassic. Likewise, plants and insects show dramatic changes. In many cases, we see a two-step process, with the initial stages occurring in the turmoil of perturbed ecosystems through the first 5–8 Myr of the Early and early Middle Triassic (252–246 Ma), followed by substantial reorganisations of marine and terrestrial ecosystems after the CPE (233–232 Ma). Thus were born the fundamentals of modern ecosystems.
Author Contributions
All authors listed have made a substantial, direct, and intellectual contribution to the work and approved it for publication.
Funding
This work was supported by a Natural Environment Research Council (NERC) UK (grant NE/P013724/1) and European Research Council (ERC) Advanced Grant (number 788203) and the Strategic Priority Research Program of the Chinese Academy of Sciences (CAS) (grant XDA20070203).
Conflict of Interest
The authors declare that the research was conducted in the absence of any commercial or financial relationships that could be construed as a potential conflict of interest.
Publisher’s Note
All claims expressed in this article are solely those of the authors and do not necessarily represent those of their affiliated organizations, or those of the publisher, the editors and the reviewers. Any product that may be evaluated in this article, or claim that may be made by its manufacturer, is not guaranteed or endorsed by the publisher.
Acknowledgments
We thank ME and YS for their very helpful comments.
References
Arratia, G. (2017). New Triassic Teleosts (Actinopterygii, Teleosteomorpha) from Northern Italy and Their Phylogenetic Relationships Among the Most Basal Teleosts. J. Vert. Paleontol. 37 (2), e1312690. doi:10.1080/02724634.2017.1312690
Bai, J., Xu, S., Nie, Z., Wang, Y., Zhu, C., Wang, Y., et al. (2018). The Complete Mitochondrial Genome of Huananpotamon Lichuanense (Decapoda: Brachyura) with Phylogenetic Implications for Freshwater Crabs. Gene 646, 217–226. doi:10.1016/j.gene.2018.01.015
Bajdek, P., Qvarnström, M., Owocki, K., Sulej, T., Sennikov, A. G., Golubev, V. K., et al. (2016). Microbiota and Food Residues Including Possible Evidence of Pre-mammalian Hair in Upper Permian Coprolites from Russia. Lethaia 49, 455–477. doi:10.1111/let.12156
Bambach, R. K., Knoll, A. H., and Sepkoski, J. J. (2002). Anatomical and Ecological Constraints on Phanerozoic Animal Diversity in the Marine Realm. Proc. Natl. Acad. Sci. U.S.A. 99, 6854–6859. doi:10.1073/pnas.092150999
Baumiller, T. K., Salamon, M. A., Gorzelak, P., Mooi, R., Messing, C. G., and Gahn, F. J. (2010). Post-paleozoic Crinoid Radiation in Response to Benthic Predation Preceded the Mesozoic Marine Revolution. Proc. Natl. Acad. Sci. U.S.A. 107, 5893–5896. doi:10.1073/pnas.0914199107
Bennett, A. F., and Nagy, K. A. (1977). Energy Expenditure in Free-Ranging Lizards. Ecology 58, 697–700. doi:10.2307/1939022
Benton, M. J., Dhouailly, D., Jiang, B., and McNamara, M. (2019). The Early Origin of Feathers. Trends Ecol. Evol. 34, 856–869. doi:10.1016/j.tree.2019.04.018
Benton, M. J., Forth, J., and Langer, M. C. (2014). Models for the Rise of the Dinosaurs. Curr. Biol. 24, R87–R95. doi:10.1016/j.cub.2013.11.063
Benton, M. J. (2021). The Origin of Endothermy in Synapsids and Archosaurs and Arms Races in the Triassic. Gondwana Res. 100, 261–289. doi:10.1016/j.gr.2020.08.003
Benton, M. J., Tverdokhlebov, V. P., and Surkov, M. V. (2004). Ecosystem Remodelling Among Vertebrates at the Permian-Triassic Boundary in Russia. Nature 432, 97–100. doi:10.1038/nature02950
Benton, M. J., Zhang, Q., Hu, S., Chen, Z.-Q., Wen, W., Liu, J., et al. (2013). Exceptional Vertebrate Biotas from the Triassic of China, and the Expansion of Marine Ecosystems after the Permo-Triassic Mass Extinction. Earth-Science Rev. 125, 199–243. doi:10.1016/j.earscirev.2013.05.014
Bernardi, M., Gianolla, P., Petti, F. M., Mietto, P., and Benton, M. J. (2018). Dinosaur Diversification Linked with the Carnian Pluvial Episode. Nat. Commun. 9, 1499. doi:10.1038/s41467-018-03996-1
Botha-Brink, J., Bento Soares, M., and Martinelli, A. G. (2018). Osteohistology of Late Triassic Prozostrodontian Cynodonts from Brazil. PeerJ 6, e5029. doi:10.7717/peerj.5029
Bown, P. R. (2005). Calcareous Nannoplankton Evolution: A Tale of Two Oceans. Micropaleontology 51, 299. doi:10.2113/gsmicropal.51.4.299
Brayard, A., Krumenacker, L. J., Botting, J. P., Jenks, J. F., Bylund, K. G., Fara, E., et al. (2017). Calcareous Nannoplankton Evolution: a Tale of Two Oceans. Micropaleontology 51, e1602159. doi:10.2113/gsmicropal.51.4.299
Brett, C. E., and Walker, S. E. (2002). Predators and Predation in Paleozoic Marine Environments. Paleontol. Soc. Pap. 8, 93–118. doi:10.1017/s1089332600001078
Buatois, L. A., Carmona, N. B., Curran, H. A., Netto, R. G., Mángano, N. G., and Wetzel, A. (2016). “The Mesozoic Marine Revolution,” in The Trace Fossil Record of Major Evolutionary Events, Volume 2. Editors M. G. Mángano, and L. A. Buatois (Dordrecht: Topics in Geobiology), 40, 18–134. doi:10.1007/978-94-017-9597-5_2
Butler, R. J., Brusatte, S. L., Reich, M., Nesbitt, S. J., Schoch, R. R., and Hornung, J. J. (2011). The Sail-Backed Reptile Ctenosauriscus from the Latest Early Triassic of Germany and the Timing and Biogeography of the Early Archosaur Radiation. PLoS ONE 6, e25693. doi:10.1371/journal.pone.0025693
Carrier, D. R. (1987). The Evolution of Locomotor Stamina in Tetrapods: Circumventing a Mechanical Constraint. Paleobiology 13, 326–341. doi:10.1017/s0094837300008903
Charig, A. J. (1972). “The Evolution of the Archosaur Pelvis and Hind-Limb: an Explanation in Functional Terms,” in Studies in Vertebrate Evolution. Editors K. A. Joysey, and T. S. Kemp (Edinburgh: Oliver & Boyd), 121–155.
Chen, W.-J., Santini, F., Carnevale, G., Chen, J.-N., Liu, S.-H., Lavoué, S. b., et al. (2014). New Insights on Early Evolution of Spiny-Rayed Fishes (Teleostei: Acanthomorpha). Front. Mar. Sci. 1, 53. doi:10.3389/fmars.2014.00053
Cincotta, A., Nicolaï, M., Campos, H. B. N., McNamara, M., D’Alba, L., Shawkey, M. D., et al. (2022). Pterosaur Melanosomes Support Signalling Functions for Early Feathers. Nature 604, 684–688. doi:10.1038/s41586-022-04622-3
Cuny, G., and Benton, M. J. (1999). Early Radiation of the Neoselachian Sharks in Western Europe. Geobios 32, 193–204. doi:10.1016/s0016-6995(99)80032-1
Dal Corso, J., Bernardi, M., Sun, Y., Song, H., Seyfullah, L. J., Preto, N., et al. (2020). Extinction and Dawn of the Modern World in the Carnian (Late Triassic). Sci. Adv. 6, eaba0099. doi:10.1126/sciadv.aba0099
Davis, J. W. (1887). The Fossil Fishes of the Chalk of Mount Lebanon in Syria. Sci. Trans. R. Dublin Soc. 3, 457
Doguzhaeva, L. A., Brayard, A., Goudemand, N., Krumenacker, L. J., Jenks, J. F., Bylund, K. G., et al. (2018). An Early Triassic Gladius Associated with Soft Tissue Remains from Idaho, USA—a Squid-like Coleoid Cephalopod at the Onset of Mesozoic Era. Acta Palaeontol. Pol. 63, 341–355. doi:10.4202/app.00393.2017
Ezcurra, M. D., Fiorelli, L. E., Trotteyn, M. J., Martinelli, A. G., and Desojo, J. B. (2020a). The Rhynchosaur Record, Including a New Stenaulorhynchine Taxon, from the Chañares Formation (Upper Ladinian–?lowermost Carnian Levels) of La Rioja Province, North-Western Argentina. J. Syst. Palaeontol. 18, 205. doi:10.1080/14772019.2020.1856205
Ezcurra, M. D., Nesbitt, S. J., Bronzati, M., Dalla Vecchia, F. M., Agnolin, F. L., Benson, R. B. J., et al. (2020b). Enigmatic Dinosaur Precursors Bridge the Gap to the Origin of Pterosauria. Nature 588, 445–449. doi:10.1038/s41586-020-3011-4
Ezcurra, M. D. (2016). The Phylogenetic Relationships of Basal Archosauromorphs, with an Emphasis on the Systematics of Proterosuchian Archosauriforms. PeerJ 4, e1778. doi:10.7717/peerj.1778
Falkowski, P. G., Katz, M. E., Knoll, A. H., Quigg, A., Raven, J. A., Schofield, O., et al. (2004). The Evolution of Modern Eukaryotic Phytoplankton. Science 305, 354–360. doi:10.1126/science.1095964
Feldmann, R. M., Schweitzer, C. E., Hu, S., Zhang, Q., Zhou, C., Xie, T., et al. (2012). Macrurous Decapoda from the Luoping Biota (Middle Triassic) of China. J. Paleontol. 86, 425–441. doi:10.1666/11-113.1
Fensome, R. A., MacRae, R. A., Moldowan, J. M., Taylor, F. J. R., and Williams, G. L. (1996). The Early Mesozoic Radiation of Dinoflagellates. Paleobiology 22, 329–338. doi:10.1017/s0094837300016316
Foster, W. J., and Twitchett, R. J. (2014). Functional Diversity of Marine Ecosystems after the Late Permian Mass Extinction Event. Nat. Geosci. 7, 233–238. doi:10.1038/ngeo2079
Fürsich, F. T., and Jablonski, D. (1984). Late Triassic Naticid Drillholes: Carnivorous Gastropods Gain a Major Adaptation but Fail to Radiate. Science 224, 78
Gillooly, J. F., Brown, J. H., West, G. B., Savage, V. M., and Charnov, E. L. (2001). Effects of Size and Temperature on Metabolic Rate. Science 293, 2248–2251. doi:10.1126/science.1061967
Grigg, G., Nowack, J., Bicudo, J. E. P. W., Bal, N. C., Woodward, H. N., and Seymour, R. S. (2022). Whole‐body Endothermy: Ancient, Homologous and Widespread Among the Ancestors of Mammals, Birds and Crocodylians. Biol. Rev. 97, 766–801. doi:10.1111/brv.12822
Harper, E. M. (2003). Assessing the Importance of Drilling Predation over the Palaeozoic and Mesozoic. Palaeogeogr. Palaeoclimatol. Palaeoecol. 201, 185–198. doi:10.1016/s0031-0182(03)00624-2
Harper, E. M., Forsythe, G. T. W., and Palmer, T. (1998). Taphonomy and the Mesozoic Marine Revolution: Preservation State Masks the Importance of Boring Predators. Palaios 13, 352–360. doi:10.2307/3515323
Hautmann, M. (2004). Early Mesozoic Evolution of Alivincular Bivalve Ligaments and its Implications for the Timing of the 'Mesozoic Marine Revolution'. Lethaia 37, 165–172. doi:10.1080/00241160410005835
Hautmann, M., Ware, D., and Bucher, H. (2017). Geologically Oldest Oysters Were Epizoans on Early Triassic Ammonoids. J. Molluscan Stud. 83, 253–260. doi:10.1093/mollus/eyx018
Hegna, T. A., Luque, J., and Wolfe, J. M. (2020). “The Fossil Record of the Pancrustacea. 21–52,” in The Natural History of the Crustacea. Vol. 8. Evolution and Biogeography of the Crustacea. Editors M. Thiel, and G. C. B. Poore (New York: Oxford University Press).
Hu, S.-x., Zhang, Q.-y., Chen, Z.-Q., Zhou, C.-y., Lü, T., Xie, T., et al. (2011). The Luoping Biota: Exceptional Preservation, and New Evidence on the Triassic Recovery from End-Permian Mass Extinction. Proc. R. Soc. B 278, 2274–2282. doi:10.1098/rspb.2010.2235
Huang, J.-d., Motani, R., Jiang, D.-y., Ren, X.-x., Tintori, A., Rieppel, O., et al. (2020). Repeated Evolution of Durophagy during Ichthyosaur Radiation after Mass Extinction Indicated by Hidden Dentition. Sci. Rep. 10, 7798. doi:10.1038/s41598-020-64854-z
Huttenlocker, A. K., and Farmer, C. G. (2017). Bone Microvasculature Tracks Red Blood Cell Size Diminution in Triassic Mammal and Dinosaur Forerunners. Curr. Biol. 27, 48–54. doi:10.1016/j.cub.2016.10.012
Irmis, R. B., and Whiteside, J. H. (2011). Delayed Recovery of Non-marine Tetrapods after the End-Permian Mass Extinction Tracks Global Carbon Cycle. Proc. R. Soc. B 279, 1310–1318. doi:10.1098/rspb.2011.1895
James, N. P. (1984). “Shallowing-upward Sequences in Carbonates,” in Faciees Models. Editor R. G. Walker. second edition (St John’s, Nova Scotia: Geoscience Canada Reprint Series), 1, 213
Jones, K. E., Angielczyk, K. D., and Pierce, S. E. (2019). Stepwise Shifts Underlie Evolutionary Trends in Morphological Complexity of the Mammalian Vertebral Column. Nat. Commun. 10, 5071. doi:10.1038/s41467-019-13026-3
Kier, P. M. (1974). Evolutionary Trends and Their Functional Significance in the Post-Paleozoic Echinoids. J. Paleontol. 48, 1–95. doi:10.1017/s0022336000061321
Klompmaker, A. A., Nützel, A., and Kaim, A. (2016). Drill Hole Convergence and a Quantitative Analysis of Drill Holes in Mollusks and Brachiopods from the Triassic of Italy and Poland. Palaeogeogr. Palaeoclimatol. Palaeoecol. 457, 342–359. doi:10.1016/j.palaeo.2016.06.017
Klug, C., Schweigert, G., Hoffmann, R., Weis, R., and De Baets, K. (2021). Fossilized Leftover Falls as Sources of Palaeoecological Data: a 'pabulite' Comprising a Crustacean, a Belemnite and a Vertebrate from the Early Jurassic Posidonia Shale. Swiss J. Palaeontol. 140, 10. doi:10.1186/s13358-021-00225-z
Kogan, I., Pacholak, S., Licht, M., Schneider, J. W., Brücker, C., and Brandt, S. (2015). The Invisible Fish: Hydrodynamic Constraints for Predator-Prey Interaction in Fossil Fish Saurichthys Compared to Recent Actinopterygians. Biol. Open 4, 1715–1726. doi:10.1242/bio.014720
Kubo, T., and Benton, M. J. (2009). Tetrapod Postural Shift Estimated from Permian and Triassic Trackways. Palaeontology 52, 1029–1037. doi:10.1111/j.1475-4983.2009.00897.x
Legendre, L. J., Guénard, G., Botha-Brink, J., and Cubo, J. (2016). Palaeohistological Evidence for Ancestral High Metabolic Rate in Archosaurs. Syst. Biol. 65, 989–996. doi:10.1093/sysbio/syw033
Lindgren, J., Sjövall, P., Thiel, V., Zheng, W., Ito, S., Wakamatsu, K., et al. (2018). Soft-tissue Evidence for Homeothermy and Crypsis in a Jurassic Ichthyosaur. Nature 564, 359–365. doi:10.1038/s41586-018-0775-x
Lovegrove, B. G. (2017). A Phenology of the Evolution of Endothermy in Birds and Mammals. Biol. Rev. 92, 1213–1240. doi:10.1111/brv.12280
Lu, J., Zhang, P. X., Dal Corso, J., Yang, M. F., Wignall, P. B., Greene, S. E., et al. (2021). Volcanically Driven Lacustrine Ecosystem Changes during the Carnian Pluvial Episode (Late Triassic). Proc. Natn. Acad. Sci. U. S. A. 118, e2109895118 doi:10.1073/pnas.2109895118
Lukashevich, E. D. (2021). The Oldest Diptera (Insecta) from the Upper Buntsandstein (Early Middle Triassic) of Europe. Zootaxa 5067, 135–143. doi:10.11646/zootaxa.5067.1.10
Luo, M., Shi, G. R., Buatois, L. A., and Chen, Z.-Q. (2020). Trace Fossils as Proxy for Biotic Recovery after the End-Permian Mass Extinction: a Critical Review. Earth-Science Rev. 203, 103059. doi:10.1016/j.earscirev.2019.103059
Mah, C. L., and Blake, D. B. (2012). Global Diversity and Phylogeny of the Asteroidea (Echinodermata). PLoS ONE 7 (4), e35644. doi:10.1371/journal.pone.0035644
Maxwell, E. E. (2016). First Middle Jurassic Record of Saurichthyidae (Actinopterygii). PalZ 90, 287–291. doi:10.1007/s12542-015-0281-5
McNab, B. K. (1978). The Evolution of Endothermy in the Phylogeny of Mammals. Am. Nat. 112, 1–21. doi:10.1086/283249
McRoberts, C. A. (2001). Triassic Bivalves and the Initial Marine Mesozoic Revolution: A Role for Predators? Geol. 29, 359–362. doi:10.1130/0091-7613(2001)029<0359:tbatim>2.0.co;2
Motani, R., Jiang, D.-Y., Chen, G.-B., Tintori, A., Rieppel, O., Ji, C., et al. (2015). A Basal Ichthyosauriform with a Short Snout from the Lower Triassic of China. Nature 517, 485–488. doi:10.1038/nature13866
Nakajima, Y., Houssaye, A., and Endo, H. (2014). Osteohistology of the Early Triassic Ichthyopterygian Reptile Utatsusaurus Hataii: Implications for Early Ichthyosaur Biology. Acta Palaeontol. Pol. 59, 343. doi:10.4202/app.2012.0045
Nesbitt, S. J., Butler, R. J., Ezcurra, M. D., Barrett, P. M., Stocker, M. R., Angielczyk, K. D., et al. (2017). The Earliest Bird-Line Archosaurs and the Assembly of the Dinosaur Body Plan. Nature 544, 484–487. doi:10.1038/nature22037
Newham, E., Gill, P. G., and Corfe, I. J. (2022). New Tools Suggest a Middle Jurassic Origin for Mammalian Endothermy. BioEssays 44, 2100060. doi:10.1002/bies.202100060
Nicholson, D. B., Mayhew, P. J., and Ross, A. J. (2015). Changes to the Fossil Record of Insects through Fifteen Years of Discovery. PLoS ONE 10 (7), e0128554. doi:10.1371/journal.pone.0128554
Nicholson, D. B., Ross, A. J., and Mayhew, P. J. (2014). Fossil Evidence for Key Innovations in the Evolution of Insect Diversity. Proc. R. Soc. B 281, 20141823. doi:10.1098/rspb.2014.1823
Norris, R. D., Turner, S. K., Hull, P. M., and Ridgwell, A. (2013). Marine Ecosystem Responses to Cenozoic Global Change. Science 341, 492–498. doi:10.1126/science.1240543
Nützel, A. (2002). The Late Triassic Species Cryptaulax? Bittneri (Mollusca: Gastropoda: Procerithiidae) and Remarks on Early Aspects of the Mesozoic Marine Revolution. Paläontol. Z. 76, 57. doi:10.1007/BF02988185
Paladino, F. V., O'Connor, M. P., and Spotila, J. R. (1990). Metabolism of Leatherback Turtles, Gigantothermy, and Thermoregulation of Dinosaurs. Nature 344, 858–860. doi:10.1038/344858a0
Radley, J. D. (2010). Grazing Bioerosion in Jurassic Seas: a Neglected Factor in the Mesozoic Marine Revolution? Hist. Biol. 22, 387–393. doi:10.1080/08912961003673079
Reijmer, J. J. G. (2021). Marine Carbonate Factories: Review and Update. Sedimentology 68, 1729–1796. doi:10.1111/sed.12878
Rezende, E. L., Bacigalupe, L. D., Nespolo, R. F., and Bozinovic, F. (2020). Shrinking Dinosaurs and the Evolution of Endothermy in Birds. Sci. Adv. 6, eaaw4486. doi:10.1126/sciadv.aaw4486
Ricqlès, A., Padian, K., Knoll, F., and Horner, J. R. (2008). On the Origin of High Growth Rates in Archosaurs and Their Ancient Relatives: Complementary Histological Studies on Triassic Archosauriforms and the Problem of a ‘phylogenetic Signal’ in Bone Histology. Ann. Paléontol. 94, 57. doi:10.1016/j.annpal.2008.03.002
Ridgwell, A. (2005). A Mid Mesozoic Revolution in the Regulation of Ocean Chemistry. Mar. Geol. 217, 339–357. doi:10.1016/j.margeo.2004.10.036
Roghi, G., Gianolla, P., Minarelli, L., Pilati, C., and Preto, N. (2010). Palynological Correlation of Carnian Humid Pulses throughout Western Tethys. Palaeogeogr. Palaeoclimatol. Palaeoecol. 290, 89–106. doi:10.1016/j.palaeo.2009.11.006
Romano, C. (2021). A Hiatus Obscures the Early Evolution of Modern Lineages of Bony Fishes. Front. Earth Sci. 8, 618853. doi:10.3389/feart.2020.618853
Romano, C., Koot, M. B., Kogan, I., Brayard, A., Minikh, A. V., Brinkmann, W., et al. (2016). Permian-Triassic Osteichthyes (Bony Fishes): Diversity Dynamics and Body Size Evolution. Biol. Rev. 91, 106–147. doi:10.1111/brv.12161
Salamon, M. A., Niedźwiedzki, R., Gorzelak, P., Lach, R., and Surmik, D. (2012). Bromalites from the Middle Triassic of Poland and the Rise of the Mesozoic Marine Revolution. Palaeogeogr. Palaeoclimatol. Palaeoecol. 321-322, 142–150. doi:10.1016/j.palaeo.2012.01.029
Sander, P. M., Griebeler, E. M., Klein, N., Juarbe, J. V., Wintrich, T., Revell, L. J., et al. (2021). Early Giant Reveals Faster Evolution of Large Body Size in Ichthyosaurs Than in Cetaceans. Science 374, eabf5787. doi:10.1126/science.abf5787
Schaeffer, B., and Rosen, D. E. (1961). Major Adaptive Levels in the Evolution of the Actinopterygian Feeding Mechanism. Am. Zool. 1, 187–204. doi:10.1093/icb/1.2.187
Scheyer, T. M., Romano, C., Jenks, J., and Bucher, H. (2014). Early Triassic Marine Biotic Recovery: The Predators' Perspective. PLoS ONE 9 (3), e88987. doi:10.1371/journal.pone.0088987
Schlager, W. (2005). Carbonate Sedimentology and Sequence Stratigraphy. Tulsa, Oklahome: Society for Sedimentary Geology, 200.
Schweitzer, C. E., and Feldmann, R. M. (2010). The Decapoda (Crustacea) as Predators on Mollusca through Geologic Time. Palaios 25, 167–182. doi:10.2110/palo.2009.p09-054r
Seymour, R. S., Bennett‐Stamper, C. L., Johnston, S. D., Carrier, D. R., and Grigg, G. C. (2004). Evidence for Endothermic Ancestors of Crocodiles at the Stem of Archosaur Evolution. Physiological Biochem. Zoology 77, 1051–1067. doi:10.1086/422766
Seymour, R. S. (2013). Maximal Aerobic and Anaerobic Power Generation in Large Crocodiles versus Mammals: Implications for Dinosaur Gigantothermy. PLoS ONE 8 (7), e69361. doi:10.1371/journal.pone.0069361
Simms, M. J., and Ruffell, A. H. (1989). Synchroneity of Climatic Change and Extinctions in the Late Triassic. Geol. 17, 265–268. doi:10.1130/0091-7613(1989)017<0265:soccae>2.3.co;2
Smith, R. M. H., and Botha-Brink, J. (2011). Morphology and Composition of Bone-Bearing Coprolites from the Late Permian Beaufort Group, Karoo Basin, South Africa. Palaeogeogr. Palaeoclimatol. Palaeoecol. 312, 40–53. doi:10.1016/j.palaeo.2011.09.006
Stanley, S. M. (1968). Post-paleozoic Adaptive Radiation of Infaunal Bivalve Molluscs: A Consequence of Mantle Fusion and Siphon Formation. J. Paleontol. 42, 214
Stanley, S. M. (1977). “Chapter 7 Trends, Rates, and Patterns of Evolution in the Bivalvia,” in Patterns of Evolution as Illustrated by the Fossil Record. Editor A. Hallam (Amsterdam): Elsevier), 209–250. doi:10.1016/s0920-5446(08)70327-3
Stubbs, T. L., and Benton, M. J. (2016). Ecomorphological Diversifications of Mesozoic Marine Reptiles: the Roles of Ecological Opportunity and Extinction. Paleobiology 42, 547–573. doi:10.1017/pab.2016.15
Suchéras‐Marx, B., Mattioli, E., Allemand, P., Giraud, F., Pittet, B., Plancq, J., et al. (2019). The Colonization of the Oceans by Calcifying Pelagic Algae. Biogeosciences 16, 2501. doi:10.5194/bg-16-2501-2019
Sullivan, C. (2015). “Evolution of Hind Limb Posture in Triassic Archosauriforms,” in Great Transformations in Vertebrate Evolution. Editors K. P. Dial, N. Shubin, and E. L. Brainerd (Chicago: University of Chicago Press), 107
Tackett, L. S., and Bottjer, D. J. (2016). Paleoecological Succession of Norian (Late Triassic) Benthic Fauna in Eastern Panthalassa (Luning and Gabbs Formations, West-Central Nevada). Palaios 31, 190–202. doi:10.2110/palo.2015.070
Tackett, L. S. (2016). Late Triassic Durophagy and the Origin of the Mesozoic Marine Revolution. Palaios 31, 122–124. doi:10.2110/palo.2016.003
Tackett, L. S., and Tintori, A. (2019). Low Drilling Frequency in Norian Benthic Assemblages from the Southern Italian Alps and the Role of Specialized Durophages during the Late Triassic. Palaeogeogr. Palaeoclimatol. Palaeoecol. 513, 25–34. doi:10.1016/j.palaeo.2018.06.034
Tanner, A. R., Fuchs, D., Winkelmann, I. E., Gilbert, M. T. P., Pankey, M. S., Ribeiro, Â. M., et al. (2017). Molecular Clocks Indicate Turnover and Diversification of Modern Coleoid Cephalopods during the Mesozoic Marine Revolution. Proc. R. Soc. B 284, 20162818. doi:10.1098/rspb.2016.2818
Tintori, A., and Sassi, D. (1992). ThoracopterusBronn (Osteichthyes: Actinopterygii): A Gliding Fish from the Upper Triassic of Europe. J. Vertebrate Paleontology 12, 265–283. doi:10.1080/02724634.1992.10011459
Twitchett, R. J., and Oji, T. (2005). Early Triassic Recovery of Echinoderms. Comptes Rendus Palevol 4, 531–542. doi:10.1016/j.crpv.2005.02.006
van Eldijk, T. J. B., Wappler, T., Strother, P. K., van der Weijst, C. M. H., Rajaei, H., Visscher, H., et al. (2018). A Triassic-Jurassic Window into the Evolution of Lepidoptera. Sci. Adv. 4, e1701568. doi:10.1126/sciadv.1701568
Van Valen, L. M. (1984). A Resetting of Phanerozoic Community Evolution. Nature 307, 50–52. doi:10.1038/307050a0
Vermeij, G. J. (2008). Escalation and its Role in Jurassic Biotic History. Palaeogeogr. Palaeoclimatol. Palaeoecol. 263, 3–8. doi:10.1016/j.palaeo.2008.01.023
Vermeij, G. J., Schindel, D. E., and Zipser, E. (1981). Predation through Geological Time: Evidence from Gastropod Shell Repair. Science 214, 1024–1026. doi:10.1126/science.214.4524.1024
Vermeij, G. J. (1977). The Mesozoic Marine Revolution: Evidence from Snails, Predators and Grazers. Paleobiology 3, 245–258. doi:10.1017/s0094837300005352
Walker, S. E., and Brett, C. E. (2002). Post-paleozoic Patterns in Marine Predation: Was There a Mesozoic and Cenozoic Marine Predatory Revolution? Paleontol. Soc. Pap. 8, 119–194. doi:10.1017/s108933260000108x
Waller, T. R. (2006). Phylogeny of Families in the Pectinoidea (Mollusca: Bivalvia): Importance of the Fossil Record. Zool. J. Linn. Soc. 148, 313342. doi:10.1111/j.1096-3642.2006.00258.x
Wang, B., Xu, C. P., and Jarzembowski, E. A. (2022). Ecological Radiations of Insects in the Mesozoic. Trends. Ecol. Evol. doi:10.1016/j10.1016/j.tree.2022.02.007
Watson, D. M. S. (1913). XXV.-Further Notes on the Skull, Brain, and Organs of Special Sense ofDiademodon. Ann. Mag. Nat. Hist. 12, 217–228. doi:10.1080/00222931308693389
Westerhold, T., Marwan, N., Drury, A. J., Liebrand, D., Agnini, C., Anagnostou, E., et al. (2020). An Astronomically Dated Record of Earth's Climate and its Predictability over the Last 66 Million Years. Science 369, 1383–1387. doi:10.1126/science.aba6853
Wintrich, T., Hayashi, S., Houssaye, A., Nakajima, Y., and Sander, P. M. (2017). A Triassic Plesiosaurian Skeleton and Bone Histology Inform on Evolution of a Unique Body Plan. Sci. Adv. 3 (12), e1701144. doi:10.1126/sciadv.1701144
Wu, F. X., Sun, Y. L., Hao, W. C., Jiang, D.-Y., and Sun, Z. Y. (2015). A New Species of Saurichthys (Actinopterygii; Saurichthyiformes) from the Middle Triassic of Southwestern China, with Remarks on Pattern of the Axial Skeleton of Saurichthyid Fishes. njgpa 275, 249–267. doi:10.1127/njgpa/2015/0462
Wu, F. X., Chang, M.-m., Sun, Y. L., and Xu, G. H. (2013). A New Saurichthyiform (Actinopterygii) with a Crushing Feeding Mechanism from the Middle Triassic of Guizhou (China). Plos One 8 (12), e81010. doi:10.1371/journal.pone.0081010
Wu, F. X., Sun, Y. L., Xu, G. H., Hao, W. C., Jiang, D. Y., and Sun, Z. Y. (2011). New Saurichthyid Actinopterygian Fishes from the Anisian (Middle Triassic) of Southwestern China. Acta Palaeontol. Pol. 56, 581. doi:10.4202/app.2010.0007
Wu, F. X., Sun, Y. L., and Fang, G. (2018). A New Species of Saurichthys from the Middle Triassic (Anisian) of Southwestern China. Vertebr. PalAsiat. 56, 273. doi:10.19615/j.cnki.1000-3118.171023
Xu, G.-H. (2020). Feroxichthys yunnanensis gen. et sp. nov. (Colobodontidae, Neopterygii), a large durophagous predator from the Middle Triassic (Anisian) Luoping Biota, eastern Yunnan, China. PeerJ 8, e10229. doi:10.7717/peerj.10229
Xu, G.-H., Zhao, L.-J., Gao, K.-Q., and Wu, F.-X. (2013). A New Stem-Neopterygian Fish from the Middle Triassic of China Shows the Earliest Over-water Gliding Strategy of the Vertebrates. Proc. R. Soc. B 280, 20122261. doi:10.1098/rspb.2012.2261
Xu, X., Zhou, Z., Dudley, R., Mackem, S., Chuong, C.-M., Erickson, G. M., et al. (2014). An Integrative Approach to Understanding Bird Origins. Science 346, 1253293. doi:10.1126/science.1253293
Yang, Z., Jiang, B., McNamara, M. E., Kearns, S. L., Pittman, M., Kaye, T. G., et al. (2019). Pterosaur Integumentary Structures with Complex Feather-like Branching. Nat. Ecol. Evol. 3, 24–30. doi:10.1038/s41559-018-0728-7
Zachos, J., Pagani, M., Sloan, L., Thomas, E., Billups, K., Pagani, M., et al. (2001). Trends, Rhythms, and Aberrations in Global Climate 65 Ma to Present. Science 292, 686–693. doi:10.1126/science.1059412
Keywords: Carnian Pluvial Episode, Mesozoic Marine Revolution, modern marine fauna, archosaurs, synapsids
Citation: Benton MJ and Wu F (2022) Triassic Revolution. Front. Earth Sci. 10:899541. doi: 10.3389/feart.2022.899541
Received: 18 March 2022; Accepted: 20 May 2022;
Published: 17 June 2022.
Edited by:
Sara Callegaro, University of Oslo, NorwayReviewed by:
Yadong Sun, University of Erlangen Nuremberg, GermanyMartin Daniel Ezcurra, Museo Argentino de Ciencias Naturales Bernardino Rivadavia, Argentina
Copyright © 2022 Benton and Wu. This is an open-access article distributed under the terms of the Creative Commons Attribution License (CC BY). The use, distribution or reproduction in other forums is permitted, provided the original author(s) and the copyright owner(s) are credited and that the original publication in this journal is cited, in accordance with accepted academic practice. No use, distribution or reproduction is permitted which does not comply with these terms.
*Correspondence: Michael J. Benton, bWlrZS5iZW50b25AYnJpc3RvbC5hYy51aw==