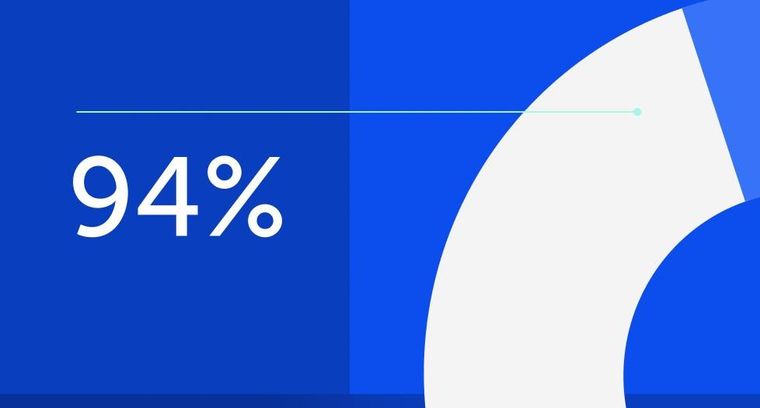
94% of researchers rate our articles as excellent or good
Learn more about the work of our research integrity team to safeguard the quality of each article we publish.
Find out more
REVIEW article
Front. Earth Sci., 12 January 2023
Sec. Structural Geology and Tectonics
Volume 10 - 2022 | https://doi.org/10.3389/feart.2022.896629
This article is part of the Research TopicEffects of Deep Fluids in Hydrocarbon Accumulations in Sedimentary BasinsView all 26 articles
Deep fluid activity is widespread in large oil-gas basins around the world. Deep fluids, as the links between internal and external factors of a basin, run in the way of organic-inorganic interactions through the oil-gas formation and aggregation. Herein, the identification characteristics of deep fluids in sedimentary basins as well as their influence on oil-gas reservoir formation and geothermal resource are summarized. The deep fluids of sedimentary basins are identified from three aspects, including mineral composition, fluid inclusions, and geochemical characteristics. The effects of deep fluid activities on oil-gas reservoir formation are manifested in two key aspects of matter and energy. As for the matter effects, deep fluids can improve the primary productivity of sedimentary basins and carry abundant inorganic hydrogen, which contributes to improving the hydrocarbon productivity through hydrogenation. As for the energy effects, the heat energy of deep fluids can promote the mature evolution from organic matter to oil and gas. During this process, the heating of deep fluids will cause the oil-generation window depth of the hydrocarbon source rocks to become thinner, and it will also generate very high pressure, which will promote the discharge of abundant hydrocarbons formed by the hydrocarbon source rocks. Furthermore, deep fluids can directly form volcanic rock oil-gas reservoirs. And another manifestation of deep fluid energy is geothermal. And the thermal energy of deep fluids can directly form hot dry rocks, which is the most important existing form of geothermal resources. The geological exploration of hot dry rocks should be supported by further geochemical and geophysical research.
The deep fluids in sedimentary basins refer to the fluids preserved in the deep crust and mantle below the basement of sedimentary basins (Andersen et al., 1984; Hauri et al., 1994; Izraeli et al., 2001). The activity of deep fluids is the carrier of energy and matter in the deep earth, and it is widespread in the major sedimentary basins worldwide (Andersen et al., 1995; Izraeli et al., 2001; Scambelluri et al., 2008). Sedimentary basins are just located in the intersection between the inner and outer layers of the earth, and this intersection is covered by the hydrosphere, biosphere and atmosphere, and underlaid by the lithosphere and mantle asthenosphere (Hitchon, 1969; Garven, 1985). During the formation of sedimentary strata, many oil-gas basins developed intrusive rocks, volcanic rocks and other endogenetic geological products. In particular, many Mesozoic-Neozoic oil-gas basins belong to volcanic sedimentary basins to some extent, in which the accumulation thickness of volcanic rock layers is up to hundreds of meters or even above one thousand meters as well as intrusive bodies in different sizes (Vogt, 1974; Mazzarini et al., 2010). Recent research on diagenesis, reservoir characterization and oil-gas systems implies that deep fluid activities affect oil-gas reservoir formation in many ways, and almost involve throughout the entire formation, migration, accumulation, and preservation of oil and gas. Hence, the oil/gas-controlling effects of deep fluids have attracted wide attention. Beides the energy and matter effects on oil-gas reservoir formation, volcanic rock oil-gas reservoirs will also be formed after the condensed crystallization of deep magmatic fluids (Luo et al., 2005; Feng, 2008).
Deep fluids will promote the formation of hydrocarbon source rocks and improve the hydrocarbon generation and expulsion efficiency (Dupuy et al., 1982; Mendelzon and Toksoz, 1985). During the evolution of sedimentary basins, the fierce active period of magma is generally the intense cracking and sinking stage of basins and the critical depositional stage of hydrocarbon source rocks. The existing research suggests that deep fluids significantly affect the formation and mature evolution of hydrocarbon source rocks. Firstly, deep fluids are favorable for the formation and preservation of original biological matter of hydrocarbons. The deep hydrothermal fluids usually carry abundant mineral elements and heat that can warm up the water bodies inside basins, while the abundant nitrogen, phosphorus and other nutrients lead to abnormal organism development in the volcano eruption environment, which contribute to the formation of parent materials (Liu et al., 2019). The temperature and salinity of active fluids rise sharply, forming anoxic conditions that are favorable for the rapid enrichment and storage of organic matter. Secondly, deep fluids can accelerate the hydrocarbon generation and expulsion of hydrocarbon source rocks. During the burying of hydrocarbon source rocks, the heat brought by magma and thermal fluids will bake and heat the hydrocarbon source rocks, which greatly promotes the hydrocarbon production quantity and speed of organic matter. Furthermore, hydrothermal or magmatic activity will bring abundant transitional metal elements (e. g. Ni, Co., V, Mn) to the surrounding hydrocarbon source rocks. Thermal simulation tests show that these transition metals can catalyze the degradation of oxygen-containing groups in organic matter and the decomposition of carbonates (Brother et al., 1991; Kawanaka et al., 1991; Larter et al., 2003; Liu et al., 2019). Hence, at the same pressure and temperature, the organic matter of hydrocarbon source rocks in active deep fluid areas will rapidly form hydrocarbons in duplicate amounts and allow the organic matter to enter the maturity threshold earlier in the case of low temperature and pressure.
Deep fluids can provide the necessary H source for oil-gas production. Thermal degradation of organic matter to form hydrocarbons is an H-consuming process, but H is a critical reducing component of deep fluids. The hydrogen of the deep source can enter sedimentary basins through two channels. Firstly, H enters sedimentary basins directly through deep degassing, and the channels are the deep and large fractures or accompanying volcanic activities at the basin basements. Secondly, secondary corrosion of ultrabasic rocks (e. g. the serpentinization of peridotite) will release H. The infusion of H-rich fluids will compensate for the H needed by the hydrocarbon production of hydrocarbon source rocks, thereby improving the hydrocarbon production efficiency of hydrocarbon source rocks. As a result, the hydrocarbon source rocks with low organic carbon concentration in the basins can also become valid for the production of abundant oil-gas (Larter et al., 2003; Tchanche et al., 2009; Dernaika et al., 20152015). According to research, due to the effect of deep fluids on the hydrogenation of hydrocarbon source rocks, the concentrations of alkanes, aromatic hydrocarbons, heterogeneous components and bitumen in hydrocarbon source rocks are somehow different from those in unaffected areas (Whelan et al., 1994).
Deep fluids can directly form volcanic rock reservoirs (Feng, 2008). The existing research on oil and gas reservoirs is mostly focused on sedimentary rocks, but in fact, igneous rocks can also act as oil and gas reservoirs under certain conditions. The intercrystal pores and gaps formed from the cooling crystallization of igneous rocks, the microfractures formed from tectonic movement, and the secondary pores resulting from the dissolution of oil-gas water media are all favorable reservoir spaces for igneous rock reservoirs. Normally, igneous rock reservoir systems are featured by the complex types and structures of reservoir space, diverse geometrical shapes, different causes, uneven distribution of pores, low connectivity, and heterogeneity among horizontal reservoirs and longitudinal reservoirs. Whether igneous rocks can become valid reservoirs depends on the lithology, lithofacies, tectonics, tectonic fluctuation, and diagenesis of igneous rocks (Zou et al., 2008; Chang et al., 2019). Furthermore, the formation of oil gas reservoirs with industrial values is decided by specific oil geological conditions, including sufficient oil-gas sources, favorable reservoir-caprock assemblages, the reservoir space formed by fracture-pore systems, valid trapping (matching between trapping time and oil-gas migration time, the spatial relationship between trapping position and oil source area), and necessary preservation conditions.
Terrestrial heat is stored inside the earth. Geothermal resources are the geothermal energy, geothermal fluids and their valuable parts inside the earth that can be economically used by humans. Geothermal resources can be divided by the causes and occurring conditions into superficial geothermal energy, hydrothermal resources and hot-dry-rock geothermal resources. Hot dry rocks are the rock mass under high underground temperature and lacking fluids owing to low porosity and penetrability. The heat stored in hot dry rocks should be exploited by artificially fracturing into the enhanced geothermal systems (Kohl et al., 1995). As for the formation mechanism, hot dry rocks are mainly formed when deep magmatic fluids invading the strata of sedimentary basins are not erupted out of the ground. The common hot dry rocks include biotite gneiss, granite and granodiorite. Hot dry rocks are nearly distributed throughout the world, and along with the geothermal temperature rise into the deep part of the earth, any place can be found with hot dry rocks at a certain depth (Zou et al., 2008). So far, the most potential areas for exploitation and utilization of hot dry rocks include the new volcanic active areas or the crust thinning areas, which are mainly located at the margins of global plates or tectonic terrains.
Herein, we statistically analyzed and summarized the deep fluid activities of oil-gas basins in China as well as their recognition marks, and elucidated the effects of deep fluids on oil-gas reservoir formation. Moreover, we preliminarily summarized and analyzed the relationship between deep fluid activity and geothermal resources in sedimentary basins, and finally clarified how the deep fluid activities of sedimentary basins affected oil-gas and geothermal resources.
It is quite difficult to research the causes and sources of deep fluids in basin. Though the sources of some fluids are clear, the views about the causes and sources of fluids are conflicting, which is mainly because the recognition marks are unknown. Hu, (2016) divided deep fluids into three types. Firstly, the fluids formed in the deep parts of basins and originating from crystallization basements are generally within the range of rigid crust and are called crust-derived fluids. Secondly, the hydrothermal fluids related to hydrocarbon formation are formed from the crude oil splitting under deep burial and thermal sulfate reduction (TSR) and are called hydrocarbon-derived hydrothermal fluids. Thirdly, the fluids originating from the mantle are called mantle-derived fluids. In previous studies, deep fluids are recognized mainly according to the mineralogical properties of rocks, the characteristics of fluid inclusions, and geochemical characteristics (Hu, 2016).
During the corrosive reconstruction by hydrothermal fluids, along with the temperature and pressure changes and the spatial migration of fluids, minerals often fill in the existing reservoir space or fissures, including calcite, dolomite, quartz, fluorite, barite, blende, chlorite and pyrite (Jin et al., 2006; Zhu et al., 2013; Hu, 2016). The major types of filling minerals are decided by the composition of the fluid-carried materials and the fluid-adjacent rock interaction.
When deep fluids invade the superficial part of basins, the temperature is usually 5 to tens of degrees Celsius above the adjacent rocks (Davies and Smith, 2006). The calcite, dolomite and quartz in deep carbonate strata possess higher homogenization temperature and higher salinity than the fluid inclusions in the strata.
The calcite cement inclusions in the tectonic fractures of the Lucaogou formation, in the Jimsar Sag, Junggar Basin possess very variable homogenization temperatures, and thereby can be divided into three intervals (Figure 1). The homogenization temperatures of inclusions within the same interval are very stable, indicating tectonic fractures are well coupled with fluid activities. Accordingly, three fluid activity phases can be determined, there are 60–109°C, 109–157°C and 157.9–211°C respectively (Figure 2). Based on the multiphase characteristics of homogenization temperatures, the tight reservoirs of the Lucaogou Formation in the Jimsar Sag experienced multiphase fracturing and fluid filling activities. Particularly, the homogenization temperatures of inclusions in the third phase are within 157.9–211°C, and these inclusions originated from deep fluid activities. In the Ordovician carbonate rocks from wells T737 and T740 in Tabei of Tarim Basin, calcite samples from several parts were found with fluid inclusions at high homogenization temperatures, as the average temperatures were 158.1 and 144.2°C respectively, which were higher than the burying temperatures of strata (Zhu et al., 2013). These two samples also have high salinity, and the average salinity of the sample from well T740 is 16.8%, indicating the cause of deep hydrothermal fluids. Compared with Tabei, the Ordovician calcite samples from Tazhong were found with fluid inclusions at higher homogenization temperatures and salinity, as the homogenization temperatures (139.2–180.8°C) were higher than the burying temperatures of strata, indicating the cause of being deep hydrothermal fluids (Figure 3). In conclusion, theoretically, the homogenization temperatures of fluid inclusions from deep fluid active areas are higher than the stratum temperatures.
FIGURE 1. Frequency histogram of the homogenization temperature (Th) for tectonic fracture calcite cements of the Lucaogou Formation in the Jimsar Sag, Junggar Basin.
FIGURE 2. Fluid inclusions from calcite fillings in tectonic fractures of the Lucaogou Formation in the Jimsar Sag, Junggar Basin, (A) homogenization temperature (Th) V.S. fluid inclusion size plot, (B) homogenization temperature (Th) V.S. ice point temperature (Tm) plot.
The common hydrothermal minerals formed under deep hydrothermal activities normally possess the geochemical characteristics of deep hydrothermal fluids (Simmons and Browne, 2000). In the Ordovician carbonate rocks in Tazhong of Tarim Basin, the calcite veins have δ18OSMOW of −7.9‰–∼14.3‰, which is an important basis for discriminating the deep fluid activities (Figure 3). The C-O isotopic distributions of calcite cements in tectonic fractures of the Lucaogou Formation in the Jimsar Sag are basically consistent with the homogenization temperatures of fluid inclusions, these can be divided into three intervals, which reflect the differences in the C-O isotopes of filling fluids at the three phases. The calcite cements in group A and B are mostly projected onto “sedimentary carbonate domain” and “fluid process”, respectively. Relatively, calcite cements in group C with δ18OSMOW of 4.51‰–17.49‰ are mostly projected onto “original mantle carbonates”, indicating calcite in group C is correlated with mantle fluid action (Figure 4).
FIGURE 4. C-O isotope diagram for calcite cements in the tectonic fractures of the Lucaogou Formation in the Jimsar Sag, Junggar Basin. Data of original mantle carbonate were from Taylor et al. (1967) and Hoefs and Hoefs, (2009); data of sedimentary carbonate were from Veizer and Hoefs, (1976) and Toyoda et al. (1994) data of mantle carbonate of South India were from Wickham et al. (1994); data of calcite cements, limestone and sandstone of Dongying Sag were from Jin and Wang, (2007).
Generally, Sr isotopes are used to trace fluid activities (Foden et al., 2001; Stewart et al., 2015). However, Sr is highly mobile in fluids and is susceptible to assimilation and contamination (Woodhead et al., 2001; Spandler et al., 2007). Accordingly, the tracing ability of Sr over the provenances of fluids is uncertain. Nd isotopes can effectively qualify the properties of calcite cements in fractures, and are unsusceptible to geological influence during fluid migration. The 143Nd/144Nd, 147Sm/144Nd, and εNd(t) of group A of calcite cements in the Jimsar Sag are 0.512767–0.512986, 0.067292–0.19731, and 6.44–9.98 respectively. These data are similar to the εNd(t) of Permian mafic-ultramafic complexes in north Xinjiang, the peridotites of Tianshan Mountains, the basalts of Beishan-Tianshan, and the surrounding basalts of Junggar Basin. Thus, it can be deduced that calcite cements of group A resulted from the hydrothermal fluid activities induced by post-collisional mantle magmatism. The Eu positive anomaly of calcite largely and directly reflects the Eu2+/Eu3+ ratio of fluids. The Eu2+/Eu3+ ratio of basin fluids is controlled by temperature, because Eu3+ is reduced to Eu2+ under a high-temperature reducing environment and the reduction is balanced at 250°C (Sverjensky, 1984; Bau and Moeller, 1992). Since Eu2+ is larger than Eu3+ in radius (0.117 vs. 0.095 nm), Eu2+ is more difficult than Eu3+ to enter rock-forming minerals. Thus, the Eu of fluids in a high-temperature environment mainly exists as Eu2+ (Figure 5). During the condensation and crystallization of hydrothermal fluids, Eu2+ is converted to Eu3+. Since Eu3+ is similar to Ca2+ in radius (0.1 nm), Eu3+ can replace Ca2+ to invade into carbonate rocks (Dasgupta et al., 2003), leading to the Eu positive anomaly in calcite of hydrothermal origin. The positive anomaly of Eu in the calcite veins and recrystallized limestone in Tazhong of Tarim Basin, and the Lucaogou Formation calcite veins in Jimsar Sag of Junggar Basin indicates the relevant fluids experienced very high temperatures and must come from the deep hydrothermal fluids. The homogenization temperatures of fluid inclusions surpassing stratum temperatures suggests that relevant fluids are hydrothermal fluids.
FIGURE 5. Rare earth element distributions of (A) Ordovician calcite in Tazhong of Tarim Basin, and (B) Lucaogou Formation Permian calcite of Jimsar Sag.
The effects of deep fluids on oil-gas reservoir formation have always been the focus of petroleum geology research. In addition to abundant magmatic melted masses, the deep earth also contains C-O-S-H fluids, which mainly exist in the form of CO, CO2, N2, H2, CH4, SO2, and H2O, and contains all the hydrocarbon-forming elements. These fluids can be considered hydrocarbon or hydrocarbon-derived materials. During the formation of hydrocarbon source rocks, deep fluids can improve the primary productivity of sedimentary basins, and can form favorable organic preservation environments. As for improving the ocean productivity, the deep fluids provide the organisms in sedimentary basins with bio-derived elements (N, P, C, Si, Fe, Zn, and Mn) and offer the sedimentary basins with archaea, thermophilic bacteria and other primary producers. The abundant CO2 and CH4 gases carried by deep fluids offer abundant carbon sources to ground surfaces and oceans. These carbon sources facilitate the synthesis of glucose, amino acids, nucleotides and other life elements, and provide sufficient CO2 for photosynthesis by terrestrial plants and the inorganic oxidation by deep-sea thermophilic bacteria (Figure 6). The carbons in deep fluids mainly originate from the carbon release in the subduction belts and deep mantle during subduction, and the deep carbon cycles include the capture of CO2 (subduction-belt sediment carbonates subducted along with oceanic crust into the internal earth), and the eruption of CO2, hydrocarbons and carbonate melts along with ocean ridges, island-arcs, mantle plume and other tectonic zone magmatic activities into ground surfaces and the atmosphere (Figure 6). The deep carbon circulation and ground surface carbon circulation jointly form the carbon circulation system on the earth, of which internal carbon content accounts for 90% of total carbon on the earth.
FIGURE 6. Phanerozoic deep carbon circulation modes (modified from Dasgupta (Dasgupta, 2013) (2013)).
Predecessors have studied the oceanic crustal subduction system and carbonate ophiolite suites and found the total carbon content from subduction belts to deep earth every year was 5.4–5.8 × 1013 g (Philp and Gilbert, 1986), and the annual average net carbon content entering the mantle was up to 3 1 × 1013 g. Other parts of captured carbon that did not reach the deep mantle mainly disengaged from subduction plates or deep mantle through the oceanic-crust metamorphosis and decarburization, the dissolution of plate dehydrated fluids, the fusion of carbonate subduction plates and deep carbonate mantle, and the redox of carbonates. Moreover, the escaping carbon entered the hydrothermal fluids in the form of CO2 or carbonate melts, and ascended with the fluids to the ground surface and atmosphere (Figure 7). Notably, carbon-bearing rocks in subduction zones produce carbon-bearing aqueous fluids (e.g., CO2-rich fluids and C-O-H fluids), graphite or diamond hydrocarbon-rich supercritical fluids and carbonatite melts by metamorphic reactions during the subduction process (Figure 7). The abundant carbon carried by deep fluids provided the carbon sources needed for the life activities of oceanic and terrestrial organisms, and promoted the thriving and primary productivity of oceanic and terrestrial organisms. This part of carbon also became the critical material basis for the formation of CO2 gas reservoirs from the inorganic origin. Due to the high temperature and flow rate of mantle source CO2, it has a strong extraction effect on surrounding rock during the flowing process, which can improve the hydrocarbon expulsion efficiency of source rock to a certain extent. The distribution of saturated n-alkanes from the source rock samples in the mantle source CO2 affected area shows a post peak pattern, indicating that the easily migrated low carbon number hydrocarbons have been discharged. However, the distribution of n-alkanes from the source rock samples in the areas not affected by the mantle source CO2 presents a stable pre peak type, which indicates that the emission of low carbon number hydrocarbons that are easy to migrate is relatively small. In addition, the neutral gas (CO2, Co, and N2) carried by the deep fluids is dissolved in the crude oil, reducing the viscosity, density and freezing point of the crude oil, which is conducive to the discharge of hydrocarbons from the source rocks (Bau and Moeller, 1992; Stewart et al., 2015). At the same time, a large amount of CO2 brought by deep fluids enters into the formation, which immensely enhances the acidity of formation water after being dissolved in water, thus forming acidic fluid, dissolving carbonate minerals in the reservoir, and effectively improving the physical properties of the reservoir. There are a lot of transitional metal elements in the deep fluids. The 3D electron layer of these transition metals is not filled, which has strong adsorption on gas and organic matter, and can break the C-C bond, C-H bond and C-O bond in organic matter to form hydrocarbons. Among them, Ni has the strongest catalytic performance among transition metal elements, even with content of 1×10−6 Ni shows strong catalytic ability (Jin and Wang, 2007).
The deep fluids are favorable for the organic matter preservation in marine and terrestrial sedimentary environments. Firstly, large-scale volcanic activities can cause massive biologic deracination and create conditions for organic matter embedding. The CO2, CH4, SO2 and other gases ejected from magma isolated air, forming a reducing environment for organic matter preservation. Secondly, the hydrothermal CO2 bound with the aqueous Ca2+ or Mg2+ to form carbonates, which increased aqueous salinity and promoted the water stratification and the stop of seawater circulation. These consequences created favorable aqueous dynamic conditions and redox status for organic enrichment (Jin et al., 2002). Furthermore, the hydrocarbon generation and expulsion of hydrocarbon source rocks in deep fluids also will be hydrogenated (Cadle, 1980; Neal and Stanger, 1983). Globally, the mantle is rich in H resources (Jin, 1998). The H-containing deep fluids can migrate, along deep and large fractures, to the superficial part of the earth and even will reach ground surfaces. Hydrogen is also a major component of volcanic gas erection (Cadle, 1980) and can be also formed in the serpentinization of mantle-derived rocks and other sources (Cadle, 1980; Neal and Stanger, 1983). Jin, (1998) designed a comparative simulation experiment on the catalytic hydrogenation of oil source rocks by using volcanic minerals, adopting the fluorspar from magmatic rocks as the catalyst, and olivine as the hydrogen source (Jin, 1998). It was found that when the organic matter reached a certain temperature, the methane yield rose by 2–3 times after adding the olivine and fluorspar (Figure 7).
Many studies have confirmed that deep fluids are very important for the formation and development of hydrocarbon source rocks, the efficiency of hydrocarbon generation and expulsion, the physical properties of reservoirs, and oil-gas migration and aggregation. Case studies indicate deep fluids can enrich the sources of hydrocarbon source rocks, carry abundant chemical components and heat, and bring some nutrients to basins, promoting the thriving and breakout of organisms in basins (Philp and Gilbert, 1986). Ocean geological surveys reveal abundant “hydrothermal organisms” were developed in the extreme deep-sea hydrothermal environments. Volcanic ash cultivation experiments indicate that, compared with the absence of volcanic ashes, the presence of volcanic ashes increased the photosynthesis efficiency and chlorophyll concentrations (Figure 8A, B). Furthermore, as the hydrothermal fluid activity stopped, due to the lack of energy and matter needed to maintain life activities, the biotic communities died in very short time, resulting in the enrichment of organic matter. Another view holds that excessive deep fluids may “inhibit the development of hydrocarbon source rocks”, and the single-layer thickness of tuff reflects the amount of nutrients carried by volcanic ashes at one time. A larger thickness reflects the larger amount of carried nutrients. In Ordos Basin Yanchang Formation, when the tuff thickness is smaller than 2 m, with the single-layer tuff thickness increases, the total organic carbon (TOC) of shale close to tuff layers gradually rises. However, when the tuff thickness is larger than 2 m, the TOC decreases with the increase of single-layer tuff thickness (Figure 9). These results suggest that when the volcanic ashes from a single sedimentation are excessive, it is unfavorable for organic matter formation.
FIGURE 8. Comparison of photosynthesis efficiency and chlorophyll contents with or without volcano ash cultivation.
The critical effects of deep fluid activities on hydrocarbon source rocks are also reflected by promoting the mature evolution of organic matter to oil and gas. The hydrocarbon formation of organic matter is also supported by the internal heat of the earth, and the hydrocarbon yield under certain conditions is also a function of temperature (Tissot and Welte, 1984). On the one hand, the heating action of deep fluids will cause the oil-generating window depth of hydrocarbon source rocks to narrower (Othman et al., 2001) and accelerate the mature evolution of peripheral hydrocarbon source rocks, forming premature oil reservoirs and increasing the hydrocarbon yields (Uysal et al., 2000; Othman et al., 2001; Jin et al., 2013). On the other hand, deep fluids also generate extremely high pressures and promote the discharge of abundant hydrocarbons formed by hydrocarbon source rocks, entering the reservoir aggregation to form reservoir formation (Wan and Jin, 2003; Jin and Wang, 2007). Based on the calculation of heat energy, Jin and Wang, (2007) found the heat brought by magmatic invasion in sedimentary basins will allow the surrounding hydrocarbon source rocks at 5 times its volume to rise in temperature by tens or even 200°C (Wan and Jin, 2003; Jin and Wang, 2007; Chang et al., 2019). Though the magmatic invasion will bring abundant heat, causing a significant temperature rise in the surrounding hydrocarbon source rocks, the acting time is relatively short and is generally only a few thousand to ten thousand years. But the cooling time of large-scale bedrocks is up to tens of thousands of years, and the influence range is directly proportional to the thickness of the intrusion, which is generally 1–2.72 times of the thickness of the intrusion (Jin, 1998). Hence, as for the medium and small-sized intrusive bodies in sedimentary basins, the magnitude of effects on hydrocarbon forming fluids should be further explored.
To sum up, the intrusive body has a significant impact on the thermal evolution of the organic matter of the surrounding rock, which makes the organic matter in it mature abnormally, but the impact time is very short. The influence range is about twice the thickness of the intrusive body in the vertical direction, and the distance from the center of the igneous rock in the horizontal direction is about the same as the diameter of the igneous rock. The huge heat brought by igneous rocks even makes the source rocks metamorphic, and even loses the ability of hydrocarbon generation. However, there is still a lack of research on the influence mechanism and influence range of the energy effect of extrusive rocks on the source rocks, especially the source rocks overlying the extrusive rocks.
The crystallized diagenesis of deep fluids will form magmatic rock reservoirs, and volcanic rock oil-gas reservoirs are a special type of oil-gas reservoirs. For a long time, it is believed that artificial volcanic activities will destroy oil-gas formation and aggregation, which is unfavorable for the formation of oil-gas reservoirs (Zerong et al., 1989). Recent research on volcanic rocks suggests that volcanic activities and volcanic rocks under certain conditions will contribute to oil-gas reservoir formation or namely the enrichment and hydrocarbon conversion of organic matter, and can act as oil-gas reservoirs and overlays, and ancient-uplift or synsedimentary anticline. East China extends from Heilongjiang and Inner Mongolia in the north to Guangdong in the south. Many faulted basins formed from North China ground cracking movements were found with volcanic rock oil-gas reservoirs that possessed high reserving and oil-producing activities. The magma, melted in the heat sources at above 1,000°C carried in large and deep fractures, will be condensed into volcanic rocks, which can provided the heat energy during heat loss needed by organic thermal metamorphism and evolution to form hydrocarbons, and act as a heat source focusing bodies. Moreover, during condensation, due to the thermal expansion, the condensed volcanic rocks will develop fissures, and some rocks contain pores after the escape of melt-out gases. From the aspect of oil geology, volcanic rocks with oil-gas reservoir abilities and oil-gas seepage abilities can form oil-gas reservoir strata, which usually include basalt, andesite, trachyte, rhyolite, and volcanic agglomerate, volcanic breccia, tuff, and other volcanic clastic rocks.
Why volcanic rocks can become oil-gas reservoir strata with commercial values can be explained by five reasons. 1) Volcanic lava is often developed with pores and abundant contraction fractures. 2) Volcanic clastic rocks are developed with abundant inter-gravel (inter-grain) pores. 3) After the volcanic lava spewed out of the surface, the physiochemical conditions will severely change, leading to extreme instability of rock structures and mineral composition, so the rocks can be easily weathered, corroded and metasomatic, forming abundant corroded pores, recrystallization pores, weathering/corroded fractures and other types of seepage spaces. 4) The Young’s modulus is larger in volcanic rocks than glutenite, and particularly large in acidic volcanic rocks than neutral or basic volcanic rocks, as manifested as high brittleness, so the rocks under tectonic forces can be easily fragmented into tectonic fissures. 5) Many volcanic rocks, due to crust activities and the long-term weathering/corrosion of the outcropped parts, are extensively developed with weathering and poured pore fractures, which fundamentally improve the reservoir performances. For this reason, the superficial intrusive rocks (also called secondary volcanic rocks, latent volcanic rocks) and metamorphic rocks that are related to the formation of volcanic rocks or accompany volcanic rocks (Jin et al., 2006; Chang et al., 2019), due to the weathering and corrosion of outcropped parts, also become oil-gas reservoir strata.
In short, in the deep horizons of basins, the physical properties of sandstone and glutenite are worsened, due to thermal metamorphism and compaction. Therefore, the sedimentary rock reservoirs in deep horizons are even worse than volcanic clastic rocks and volcanic rocks. From the aspect of oil-gas reservoirs, volcanic rocks are of favorable lithology. Thus, the unique and favorable geological conditions are the geological foundation for the formation of volcanic rock oil-gas reservoirs in the rift valley basins of East China.
The heat energy of hot dry rocks is stored in various metamorphic rocks or crystallized rocks, and some common hot dry rocks include biotite gneiss, granide, and granodiorite (Kumari et al., 2018; Ma et al., 2020; Wang et al., 2021). Hot dry rocks are featured by enormous heat energy storage, high utilization efficiency, and systematic stability. The hot dry rocks at 3–10 km account for 90% of geothermal resources in the world, so hot dry rocks are the major existing form of geothermal resources. The exploitation of hot dry rocks will open a huge thermal pool in the world. The exploitation and utilization of hot dry rocks depend on artificially fabricated heat storage, and high-pressure infusion of low-temperature water into injection wells (recharge wells) will cause fractures in rock masses. During the continual injection of low-temperature water, the fractures are gradually enlarged, expanded and connected, forming a roughly plane-shaped artificial hot dry rock heat storage structure (Kohl et al., 1995).
After crystallized diagenesis, deep fluids will become magmatic rocks or high-temperature granitoid rocks, further forming geothermal resources. In China, the Tengchong Volcanic Group is characterized by intense hydrothermal activities, such as boiling springs, intermittent fountains, hydrothermal explosions, high-temperature jet holes and steam emitting surfaces. It is a favorable area for geothermal research. The abundant geothermal resources coincide with the Tengchong volcano, indicating that there is a heat source in the crust in this area. Through three-dimensional resistivity inversion, three independent small-scale shallow magmatic chambers are found in the upper crust (10–20 km) of the Tengchong Volcanic Area, with an average temperature of 850–950°C (Tao et al., 2018). Three-dimensional resistivity inversion of the lower crust and deeper in the Tengchong Volcanic Area shows that there is a large basaltic magma reservoir with a volume of about 7,000 km3 in the lower crust (20–35 km depth). The deep crust magma reservoir is supplied by the partial melting of the uppermost mantle, and the shallow magma chamber is supplied by the lower crust magma reservoir (Zhao et al., 2021). The existence of shallow magma chamber not only provides heat source for high-temperature geothermal resources in the Tengchong area, but also provides a possibility for the existence of supercritical geothermal resources in the Tengchong area (Wang et al., 2022).
So far, research on hot dry rocks exploration is still at the starting stage. The geological exploration of hot dry rocks must be assisted by further geochemical and geophysical studies.
The deep fluids of sedimentary basins can be identified from three aspects of mineral composition, fluid inclusions, and geochemical characteristics. The relationship between deep fluid activity and basin oil-gas reservoir formation is all-dimensional, as deep fluid activity is involved in the diagenesis of hydrocarbon source rocks, reservoirs and overlays, and affects the whole formation, migration, aggregation, storage and destruction of oil-gas. Generally, the effects of deep fluid activities on oil-gas reservoir formation are manifested in two key aspects of matter and energy. As for the matter effects, deep fluids can improve the primary productivity of sedimentary basins, and can form favorable organic preservation environments. Deep fluids normally carry abundant inorganic hydrogen, so hydrocarbon production efficiency can be improved by hydrogenation. The energy effects are reflected by promoting the mature evolution of organic matter to oil and gas. The heating by deep fluids will narrow the oil-generating window depth of hydrocarbon source rocks. Deep fluids also generate extremely high pressures and promote the discharge of abundant hydrocarbons formed by hydrocarbon source rocks, entering the reservoir aggregation to form reservoir formation. Furthermore, the deep fluids can directly form volcanic rock oil-gas reservoirs. As for geothermal, deep fluids can directly form hot dry rocks. The geological exploration of hot dry rocks is at the starting stage, and must be assisted by further geochemical and geophysical studies.
JL: Conceptualization, Writing—review and editing, Supervision. CL—review and editing. MW: Conceptualization, Methodology, Writing. CZ: Revise and Polish the article.
This work is financially supported by the grants from the National Natural Science Foundation of China (No. 42002050), and the China Postdoctoral Science Foundation Funded Project (No. 2020M680815).
Author JL was employed by China Non-ferrous Metals Resource Geological Survey, Beijing, China.
The remaining authors declare that the research was conducted in the absence of any commercial or financial relationships that could be construed as a potential conflict of interest.
All claims expressed in this article are solely those of the authors and do not necessarily represent those of their affiliated organizations, or those of the publisher, the editors and the reviewers. Any product that may be evaluated in this article, or claim that may be made by its manufacturer, is not guaranteed or endorsed by the publisher.
Andersen, T., Burke, E., and Neumann, E.-R. (1995). Nitrogen-rich fluid in the upper mantle: Fluid inclusions in spinel dunite from lanzarote, canary islands. Contr. Mineral. Pet. 120 (1), 20–28. doi:10.1007/bf00311005
Andersen, T., O'Reilly, S. Y., and Griffin, W. (1984). The trapped fluid phase in upper mantle xenoliths from victoria, Australia: Implications for mantle metasomatism. Contr. Mineral. Pet. 88 (1-2), 72–85. doi:10.1007/bf00371413
Bau, M., and Moeller, P. (1992). Rare Earth element fractionation in metamorphogenic hydrothermal calcite, magnesite and siderite. Mineralogy Petrology 45 (3-4), 231–246. doi:10.1007/bf01163114
Brother, L., Engel, M. H., and Krooss, B. M. (1991). The effects of fluid flow through porous media on the distribution of organic compounds in a synthetic crude oil. Org. Geochem. 17 (1), 11–24. doi:10.1016/0146-6380(91)90036-j
Cadle, R. D. (1980). A comparison of volcanic with other fluxes of atmospheric trace gas constituents. Rev. Geophys. 18 (4), 746–752. doi:10.1029/rg018i004p00746
Chang, X., Wang, Y., Shi, B., and Xu, Y. (2019). Charging of Carboniferous volcanic reservoirs in the eastern Chepaizi uplift, Junggar Basin (northwestern China) constrained by oil geochemistry and fluid inclusion. Am. Assoc. Pet. Geol. Bull. 103 (7), 1625–1652. doi:10.1306/12171818041
Dasgupta, R. (2013). Ingassing, storage, and outgassing of terrestrial carbon through geologic time. Rev. Mineralogy Geochem. 75 (1), 183–229. doi:10.2138/rmg.2013.75.7
Dasgupta, S., Mukhopadhyay, M., Bhattacharya, A., and Jana, T. K. (2003). The geometry of the Burmese-Andaman subducting lithosphere. J. Seismol. 7 (2), 155–174. doi:10.1023/a:1023520105384
Davies, G. R., and Smith, L. B. (2006). Structurally controlled hydrothermal dolomite reservoir facies: An overview. Am. Assoc. Pet. Geol. Bull. 90 (11), 1641–1690. doi:10.1306/05220605164
Dernaika, M., Jallad, O. A., Koronfol, S., Suhrer, M., Teh, W. J., Walls, J., et al. (20152015). “Petrophysical and fluid flow properties of a tight carbonate source rock using digital rock physics,” in Proceedings unconventional resources Technology conference (San Antonio, Texas: Society of Exploration Geophysicists, American Association of Petroleum), 2550–2564.
Dupuy, C., Dostal, J., Marcelot, G., Bougault, H., Joron, J., and Treuil, M. (1982). Geochemistry of basalts from central and southern new hebrides arc: Implication for their source rock composition. Earth Planet. Sci. Lett. 60 (2), 207–225. doi:10.1016/0012-821x(82)90004-8
Feng, Z.-q. (2008). Volcanic rocks as prolific gas reservoir: A case study from the qingshen gas field in the songliao basin, ne China. Mar. Petroleum Geol. 25 (4-5), 416–432. doi:10.1016/j.marpetgeo.2008.01.008
Foden, J., Barovich, K., Jane, M., and O'Halloran, G. (2001). Sr-Isotopic evidence for late neoproterozoic rifting in the adelaide geosyncline at 586 Ma: Implications for a Cu ore forming fluid flux. Precambrian Res. 106 (3-4), 291–308. doi:10.1016/s0301-9268(00)00132-7
Garven, G. (1985). The role of regional fluid flow in the Genesis of the Pine Point deposit, Western Canada sedimentary basin. Econ. Geol. 80 (2), 307–324. doi:10.2113/gsecongeo.80.2.307
Hauri, E. H., Whitehead, J. A., and Hart, S. R. (1994). Fluid dynamic and geochemical aspects of entrainment in mantle plumes. J. Geophys. Res. 99 (B12), 24275–24300. doi:10.1029/94jb01257
Hitchon, B. (1969). Fluid flow in the western Canada sedimentary basin: 2. Effect of geology. Water Resour. Res. 5 (2), 460–469. doi:10.1029/wr005i002p00460
Hu, W. (2016). Origin and indicators of deep-seated fluids in sedimentary basins. Bull. Mineralogy , Petrology Geochem. 35 (5), 817–826.
Izraeli, E. S., Harris, J. W., and Navon, O. (2001). Brine inclusions in diamonds: A new upper mantle fluid. Earth Planet. Sci. Lett. 187 (3-4), 323–332. doi:10.1016/s0012-821x(01)00291-6
Jin, Q. (1998). Hydrocarbon generation in rift basins, Eastern China: Catalysis and hydrogenation-interaction between vlcanic minerals and organic matter. Adv. Earth Sci. 13 (6), 136–142.
Jin, Z., Zhang, L., Yang, L., and Hu, W. (2002). Primary study of geochemical features of deep fluids and their effectiveness on oil/gas reservoir formation in sedimental basins. Earth Sci. - J. China Univ. Geoscience 27 (6), 659–665. doi:10.3321/j.issn:1000-2383.2002.06.001
Jin, Z., and Wang, X. (2005). Petroleum accumulation and distribution prediction in typical superimposed basins in China. Oil Gas Geol. (03), 3–4. SCIENCE PRESS
Jin, Z., Zhu, D., Hu, W., Zhang, X., Wang, Y., and Yan, X. (2006). Geological and geochemical signatures of hydrothermal activity and their influence on carbonate reservoir beds in the Tarim Basin. Acta Geol. Sin. 80 (2), 245–254. (in Chinese with English abstract). doi:10.3321/j.issn:0001-5717.2006.02.009
Jin, Z., Zhu, D., Meng, Q., and Hu, W. (2013). Hydrothermal activites and influences on migration of oil and gas in Tarim Basin. Acta Petrol. Sin. 29 (3), 1048–1058.
Kawanaka, S., Park, S. J., and Mansoori, G. A. (1991). Organic deposition from reservoir fluids: A thermodynamic predictive technique. SPE Reserv. Eng. 6 (02), 185–192. doi:10.2118/17376-pa
Kohl, T., Evansi, K., Hopkirk, R., and Rybach, L. (1995). Coupled hydraulic, thermal and mechanical considerations for the simulation of hot dry rock reservoirs. Geothermics 24 (3), 345–359. doi:10.1016/0375-6505(95)00013-g
Kumari, W. G. P., Ranjith, P. G., Perera, M. S. A., Li, X., Li, L. H., Chen, B. K., et al. (2018). Hydraulic fracturing under high temperature and pressure conditions with micro CT applications: Geothermal energy from hot dry rocks. Fuel 230, 138–154. doi:10.1016/j.fuel.2018.05.040
Larter, S., Huang, H., Adams, J., Bennett, B., Jokanola, O., Oldenburg, T., et al. (2003). The controls on the composition of biodegraded oils in the deep subsurface: Part II—geological controls on subsurface biodegradation fluxes and constraints on reservoir-fluid property prediction. Am. Assoc. Pet. Geol. Bull. 90 (6), 921–938. doi:10.1306/01270605130
Liu, Q., Zhu, D., Meng, Q., Liu, J., Wu, X., Zhou, B., et al. (2019). The scientific connotation of oil and gas formations under deep fluids and organic-inorganic interaction. Sci. China Earth Sci. 62 (3), 507–528. doi:10.1007/s11430-018-9281-2
Luo, J., Morad, S., Liang, Z., and Zhu, Y. (2005). Controls on the quality of Archean metamorphic and Jurassic volcanic reservoir rocks from the Xinglongtai buried hill, Western depression of Liaohe basin, China. Am. Assoc. Pet. Geol. Bull. 89 (10), 1319–1346. doi:10.1306/05230503113
Ma, W., Wang, Y., Wu, X., and Liu, G. (2020). Hot dry rock (HDR) hydraulic fracturing propagation and impact factors assessment via sensitivity indicator. Renew. Energy 146, 2716–2723. doi:10.1016/j.renene.2019.08.097
Mazzarini, F., Ferrari, L., and Isola, I. (2010). Self-similar clustering of cinder cones and crust thickness in the Michoacan–Guanajuato and Sierra de Chichinautzin volcanic fields, Trans-Mexican Volcanic Belt. Tectonophysics 486 (1-4), 55–64. doi:10.1016/j.tecto.2010.02.009
Mendelzon, J., and Toksoz, M. N. “Source rock characterization using multivariate analysis of log data,” in Proceedings SPWLA 26th annual logging Symposium1985 (Society of Petrophysicists and Well-Log Analysts).
Neal, C., and Stanger, G. (1983). Hydrogen generation from mantle source rocks in Oman. Earth Planet. Sci. Lett. 66, 315–320. doi:10.1016/0012-821x(83)90144-9
Othman, R., Arouri, K. R., Ward, C. R., and McKirdy, D. M. (2001). Oil generation by igneous intrusions in the northern Gunnedah Basin, Australia. Org. Geochem. 32 (10), 1219–1232. doi:10.1016/s0146-6380(01)00089-4
Philp, R. t., and Gilbert, T. (1986). Biomarker distributions in Australian oils predominantly derived from terrigenous source material. Org. Geochem. 10 (1-3), 73–84. doi:10.1016/0146-6380(86)90010-0
Scambelluri, M., Pettke, T., and Van Roermund, H. (2008). Majoritic garnets monitor deep subduction fluid flow and mantle dynamics. Geol. 36 (1), 59–62. doi:10.1130/g24056a.1
Simmons, S. F., and Browne, P. R. (2000). Hydrothermal minerals and precious metals in the Broadlands-Ohaaki geothermal system: Implications for understanding low-sulfidation epithermal environments. Econ. Geol. 95 (5), 971–999. doi:10.2113/95.5.971
Spandler, C., Mavrogenes, J., and Hermann, J. (2007). Experimental constraints on element mobility from subducted sediments using high-P synthetic fluid/melt inclusions. Chem. Geol. 239 (3-4), 228–249. doi:10.1016/j.chemgeo.2006.10.005
Stewart, B. W., Chapman, E. C., Capo, R. C., Johnson, J. D., Graney, J. R., Kirby, C. S., et al. (2015). Origin of brines, salts and carbonate from shales of the Marcellus Formation: Evidence from geochemical and Sr isotope study of sequentially extracted fluids. Appl. Geochem. 60, 78–88. doi:10.1016/j.apgeochem.2015.01.004
Sverjensky, D. A. (1984). Oil field brines as ore-forming solutions. Econ. Geol. 79 (1), 23–37. doi:10.2113/gsecongeo.79.1.23
Tao, Y. E., Huang, Qinghua, Chen, Xiaobin, Zhang, H., Chen, Y. J., Zhao, L., et al. (2018). Magma chamber and crustal channel flow structures in the Tengchong volcano area from 3-D MT inversion at the intracontinental block boundary southeast of the Tibetan plateauow structures in the Tengchong volcano area from 3-D MT inversion at the intracontinental block boundary southeast of the Tibetan plateau[J]. J. Geophys. Res. Solid Earth 123 (12), 11112–11126. doi:10.1029/2018jb015936
Taylor, H. P., Frechen, J., and Degens, E. T. (1967). Oxygen and carbon isotope studies of carbonatites from the laacher see district, west Germany and the alnö district, Sweden. Geochimica Cosmochimica Acta 31 (3), 407–430. doi:10.1016/0016-7037(67)90051-8
Tchanche, B. F., Papadakis, G., Lambrinos, G., and Frangoudakis, A. (2009). Fluid selection for a low-temperature solar organic Rankine cycle. Appl. Therm. Eng. 29 (11- 12), 2468–2476. doi:10.1016/j.applthermaleng.2008.12.025
Tissot, B. P., and Welte, D. H. (1984). From kerogen to petroleum, Petroleum formation and occurrence. Springer, 160–198.
Toyoda, K., Horiuchi, H., and Tokonami, M. (1994). Dupal anomaly of Brazilian carbonatites: Geochemical correlations with hotspots in the South atlantic and implications for the mantle source. Earth Planet. Sci. Lett. 126 (4), 315–331. doi:10.1016/0012-821x(94)90115-5
Uysal, I., Golding, S., and Glikson, M. (2000). Petrographic and isotope constraints on the origin of authigenic carbonate minerals and the associated fluid evolution in Late Permian coal measures, Bowen Basin (Queensland), Australia. Sediment. Geol. 136 (3-4), 189–206. doi:10.1016/s0037-0738(00)00097-x
Veizer, J., and Hoefs, J. (1976). The nature of O18/O16 and C13/C12 secular trends in sedimentary carbonate rocks. Geochimica Cosmochimica Acta 40 (11), 1387–1395. doi:10.1016/0016-7037(76)90129-0
Vogt, P. R. (1974). Volcano spacing, fractures, and thickness of the lithosphere. Earth Planet. Sci. Lett. 21 (3), 235–252. doi:10.1016/0012-821x(74)90159-9
Wan, C., and Jin, Q. (2003). Study on exceptional hydrocarbons generating and eliminating of gabbros to source rocks in Chunxi area of Dongying Depression. J. Chang'An Univ. (Earth Sci. Ed. 25 (1), 20–26.
Wang, D., Bian, X., Qin, H., Sun, D., and Yu, B. (2021). Experimental investigation of mechanical properties and failure behavior of fluid-saturated hot dry rocks. Nat. Resour. Res. 30 (1), 289–305. doi:10.1007/s11053-020-09760-x
Wang, Y., Zhou, J., Li, L., Wen, H., Song, R., Jiang, G., et al. (2022). Geothermal geological conditions in the Yangbajing geothermal field and its enlightenment to the exploration of supercritical geothermal resources[J]. Nat. Gas. Ind. 42 (4), 35–45.
Whelan, J. K., Kennicutt, M. C., Brooks, J. M., Schumacher, D., and Eglington, L. B. (1994). Organic geochemical indicators of dynamic fluid flow processes in petroleum basins. Org. Geochem. 22 (3-5), 587–615. doi:10.1016/0146-6380(94)90127-9
Wickham, S., Janardhan, A., and Stern, R. (1994). Regional carbonate alteration of the crust by mantle-derived magmatic fluids, Tamil Nadu, South India. J. Geol. 102 (4), 379–398. doi:10.1086/629681
Woodhead, J., Hergt, J., Davidson, J., and Eggins, S. (2001). Hafnium isotope evidence for ‘conservative’element mobility during subduction zone processes. Earth Planet. Sci. Lett. 192 (3), 331–346. doi:10.1016/s0012-821x(01)00453-8
Zerong, L., Quanlin, X., Yongjie, W., Peiqin, X., and Xiaofeng, Z. (1989). Formation conditions and distribution regularities of oil-gas pools in Tertiary volcanic rocks in Western Huimin Depression, Shandong Province. Chin. J. Geochem. 6638 (2), 146–154. doi:10.1007/bf02840438
Zhao, Y., Guo, Z., Wang, K., and Yang, Y. J. (2021). A large magma reservoir beneath the Tengchong volcano revealed by ambient noise adjoint tomography. JGR. Solid Earth 126 (7), e2021JB022116. doi:10.1029/2021jb022116
Zhu, D. Y., Meng, Q. Q., Hu, W. X., and Jin, Z. J. (2013). Differences between fluid activities in the central and north Tarim Basin. Geochimica 42 (2), 82–94. (in Chinese with English abstract).
Keywords: deep fluids, primary productivity of sedimentary basins, hydrocarbon source rocks, geothermal resources, Oil-gas reservoir
Citation: Lu J, Li C, Wang M and Zhang C (2023) Review of deep fluids in sedimentary basins and their influence on resources, with a focus on oil and geothermal exploitation. Front. Earth Sci. 10:896629. doi: 10.3389/feart.2022.896629
Received: 15 March 2022; Accepted: 31 October 2022;
Published: 12 January 2023.
Edited by:
Dongya Zhu, Sinopec Petroleum Exploration and Production Research Institute, ChinaReviewed by:
Tao Chuanqi, Liaoning Shihua University, ChinaCopyright © 2023 Lu, Li, Wang and Zhang. This is an open-access article distributed under the terms of the Creative Commons Attribution License (CC BY). The use, distribution or reproduction in other forums is permitted, provided the original author(s) and the copyright owner(s) are credited and that the original publication in this journal is cited, in accordance with accepted academic practice. No use, distribution or reproduction is permitted which does not comply with these terms.
*Correspondence: Chuanming Li, bGljaHVhbm1pbmcwMzIyQDE2My5jb20=
†These authors have contributed equally to this work
Disclaimer: All claims expressed in this article are solely those of the authors and do not necessarily represent those of their affiliated organizations, or those of the publisher, the editors and the reviewers. Any product that may be evaluated in this article or claim that may be made by its manufacturer is not guaranteed or endorsed by the publisher.
Research integrity at Frontiers
Learn more about the work of our research integrity team to safeguard the quality of each article we publish.