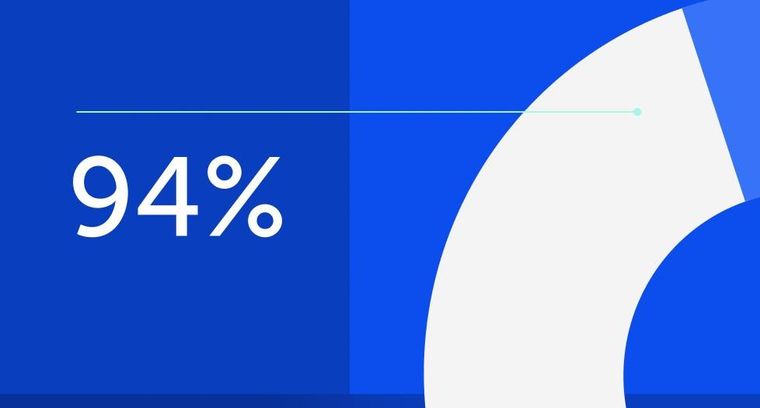
94% of researchers rate our articles as excellent or good
Learn more about the work of our research integrity team to safeguard the quality of each article we publish.
Find out more
HYPOTHESIS AND THEORY article
Front. Earth Sci., 09 May 2022
Sec. Biogeoscience
Volume 10 - 2022 | https://doi.org/10.3389/feart.2022.886395
This article is part of the Research TopicSedimentation on the Continental Margins: From Modern Processes to Deep-Time RecordsView all 28 articles
Continental margin sediments represent a major global sink of organic carbon (OC), and as such exert a key control on Earth’s climate. Today, OC burial in marine sediments mainly takes place under oxygen-rich water columns, where most OC is stabilized through intimate association with sediment grains and biogenic minerals. In prior episodes of Earth’s past, when large parts of the oceans were anoxic, the mode of sedimentary OC burial must have been very different, however. Present-day analogues indicate that surface sediments accumulating under low-oxygen water columns are often “soupy” in texture. Moreover, most OC occurs in large (100–2,000 μm diameter) organic and organo-mineral aggregates which, due to their low density, are prone to wave- and current-induced resuspension. Upon mobilization, these aggregates can undergo lateral transport within so-called nepheloid layers, and may be translocated hundreds of kilometres, and on timescales of thousands of years. Little is known about processes of formation, resuspension and hydrodynamic properties of these aggregates in oxygen-poor waters, or which factors control their eventual breakdown or burial. The goal of this study is to examine the drivers and biogeochemical consequences of this resuspension on OC cycling in modern, oxygen-depleted, “Semi-Liquid Ocean Bottom” (SLOB) regions. We argue that models of sediment and OM hydrodynamics and redistribution that describe sedimentation processes in oxygenated ocean waters of the modern ocean are a poor analogue for equivalent processes occurring under oxygen-deficient conditions. In the latter, we hypothesize that 1) the abundance of low-density organic-rich particles and aggregates leads to a greater propensity for sediment remobilization at low(er) shear stress, and 2) upon resuspension into low-oxygen bottom waters, remobilized OM may be subject to less degradation (less attenuation) during lateral transport, leading to efficient and widespread translocation to distal centres of deposition. We address specific aspects of the SLOB hypothesis utilizing a combination of literature and new data, focussing on the Benguela Upwelling Region as a model system.
Organic matter burial in marine sediments comprises an integral component of the global carbon cycle and is of fundamental importance in regulating the balance of CO2 and O2 in the atmosphere on geological timescales (Berner, 2001; Mills et al., 2019). Continental margins and other high productivity regions are among the most prolific sites of burial despite the fact that they only make up <20% of the whole ocean (Premuzic et al., 1982; Hedges and Keil, 1995; Burdige, 2007; Arndt et al., 2013). Organic matter preserved in these depositional environments on geologic timescales also provides key insights into past oceanic conditions.
Sinking particles derived from surface ocean biological productivity are the main conveyor of organic matter and nutrients to the sea floor, orchestrated by the “biological pump” (Passow and De La Rocha, 2006; Honjo et al., 2008). Collisions and interactions between organic and inorganic matter in the surface ocean promote flocculation, and interparticle attractive forces (e.g., polymer bridges), as well as biological processing, facilitate the subsequent coagulation to larger particles (e.g., faecal pellets and aggregates) (e.g., Israelachvili, 2011). These large particles promote export flux of organic carbon due to their size and excess density compared to the surrounding sea water (Armstrong et al., 2002; Klaas and Archer, 2002; Honjo et al., 2008). Still on the low-density spectrum, however, these organic matter-rich sinking particles may remain in stationary suspension in the benthic boundary layer for protracted periods of time. Strong and variable bottom currents, as well as storm-driven resuspension events, may result in the development of bottom nepheloid layers—a near-bed water body containing increased amounts of particulate material (Jahnke, 2009; McCave, 2009). These may extend several hundred meters above the sea floor (McCave, 2009) and repeated internal resuspension-deposition cycles result in widespread lateral transport and sediment redistribution. These hydrodynamic processes, along with occlusion in biominerals, close association with detrital mineral phases and the seclusion from oxic conditions within the microenvironment of the aggregate, result in preferential transport of fine-grained minerals, frequently resulting in corresponding deposits of fine-grained minerals and associated organic carbon on upper continental slopes (Keil et al., 1994b; Ransom et al., 1998; Francois et al., 2002; Ingalls et al., 2004; Iversen and Ploug, 2010).
Under oceanographic settings that are characterized by high primary productivity (e.g., upwelling regions, riverine nutrient inputs) and/or restricted water circulation (e.g., water column stratification, semi-enclosed basins), high rates of aerobic organic matter degradation in the water column may lead to pronounced oxygen depletion in bottom waters, promoting organic matter accumulation on the sea floor. Such locations often exhibit a pronounced fuzzy sediment-water interface and a soupy sediment texture owing to the accumulation of low-density and organic matter-rich aggregates in “fluff” or “flocc” layers (e.g., Beier et al., 1991). Such material has a greater propensity for resuspension, and lateral transport of these particles may promote redistribution and formation of local hotspots of sedimentary carbon (Bianchi et al., 2018). The quality and quantity of OC preserved in these hotspots is directly related to the duration of exposure to molecular oxygen during transport and deposition—the oxygen exposure time (OET) (Arnarson and Keil, 2007; e.g., Hartnett et al., 1998; Keil et al., 2004). Thus, whether particulate organic matter is exported from the surface ocean to oxygen-rich or oxygen-depleted bottom waters may influence the degradation rate and burial location of organic matter in continental margin sediments.
While organic matter preservation and dynamics under anoxic conditions have been the subject of numerous investigations, the impact of organic carbon quantity and type on sediment hydrodynamic properties has been largely unstudied. Here, we argue that these processes should be considered in order to better understand the sedimentological drivers of organic carbon burial in the ocean. Low oxygen settings are spatially restricted in the modern ocean (Moffitt et al., 2015), but such conditions were much more prevalent earlier in Earth’s history (e.g., Schlanger and Jenkyns, 1976; Jenkyns, 2010), and will assume greater significance with on-going ocean deoxygenation (Keeling et al., 2010; Breitburg et al., 2018; Oschlies et al., 2018). Hence, these questions may take on greater relevance with respect to carbon burial on continental margins and in the deep ocean in the past and future.
In this paper, we merge published insights with new observations on the Benguela Upwelling System to present a conceptual framework for understanding the fate of sedimentary organic carbon in oxygen-depleted continental margin environments. Based on this model system, we discuss the properties of soupy, “Semi-Liquid Ocean Bottom” (SLOB) sediments and their broader-scale effects on organic matter distribution and burial in the ocean. In this context, we discuss the importance of hydrodynamic factors in controlling organic carbon transport and burial today, and for the interpretation of past paleoceanographic conditions based on sedimentary records. Such conditions may affect how we understand past and future ocean carbon storage capacity, considering the projected global warming and spread in oxygen minimum zones.
The surface sediment cores measured for this study were recovered in five separate sampling trips to the Benguela Upwelling System (BUS) in 2014–2017 and 2019. Sampling focused on a series of cross-margin transects at 20, 23 and 25–26°S that included locations of high sedimentary organic carbon content (Figure 1). The BUS lies along offshore Namibia on the south-western margin of Africa and is characterized by localized seasonal cells of coastal upwelling that cause intense primary production, the spread of low-oxygen bottom waters and a high rate of OC burial (Nelson and Hutchings, 1983; Barlow et al., 2009; Junker et al., 2017). Two large oceanic currents define the BUS, namely the warm southward-directed Angola undercurrent and the cold northward-flowing Benguela surface current (Shannon et al., 1996). They roughly follow the main shelf break where an alongshore thermal front defines the extent of the upwelling cells (Summerhayes et al., 1995; Fennel, 1999; Skogen, 1999). They also play a key role in the formation of oxygen minimum zones (OMZs) depending on seasonal fluctuations in current intensity (Mohrholz et al., 2008).
FIGURE 1. Overview of study area (large right panel), including the three BUS upwelling cells that were studied boxes (A–C). Black symbols refer to MOSAIC database stations (Van Der Voort et al., 2021). Stations shown as white circles were analysed in this study. On the shelf, two large currents confine the BUS: an undercurrent from the north (nutrient-poor, oxygen-rich) and a surface current from the Angola Gyre (nutrient-rich, oxygen-poor) (modified from NCEI—National Centers for Environmental Information, 2016). Ocean colour (NPP, 4 km resolution) shows the typical distribution of high productivity inshore (NASA, 2018) and thus highlights the major upwelling zones. Total organic carbon (TOC; left panels) is focused in belts along the coast and is additionally transported to and concentrates in depositional centres (“depocenters”) offshore the major upwelling cells (D). The highest TOC values are present in muddy depocenters 20 and 50 nm offshore Walvis Bay (B) and Lüderitz (C), respectively (data from Inthorn et al., 2006b). Bottom water oxygen concentrations are low across the shelf and reach minimal values on the platform between 50 and 100 m water depth (e.g., Lavik et al., 2009).
The upwelled nutrient-rich water leads to high productivity in the coastal surface ocean and high vertical export of freshly produced, labile OM to the lower water column and underlying sediments. Remineralization by aerobic organoheterotrophs consumes the oxygen in the water column and creates an OMZ (<0.5 ml L−1 O2) that extends along and over the shelf (Lahajnar et al., 2014; Levin, 2003), across the two shelf breaks that mark the inner (50–150 m water depth) and outer shelf (300 m water depth). The inner shelf is characterized by a diatomic mud belt, with high OM preservation and burial, and sedimentary OC contents as high as 20 wt% (Inthorn et al., 2006b; Lass and Mohrholz, 2005; Mohrholz et al., 2008); Figure 1). Most effective deposition focuses on three along-shore belts on the continental margin and a large offshore depocenter at 25–26°S (800–2000 m water depth) (Inthorn et al., 2006b; Van Der Plas et al., 2007). Seasonal stratification, high sedimentation rates coupled with low water column oxygen concentrations (episodically <0.1 ml L−1 O2) (Levin, 2003; Arning et al., 2008) in the bottom water layers, and relatively low cross-shelf current velocities offshore (0.04–0.06 m s−1) support efficient OM burial (Bailey, 1991; Mollenhauer et al., 2004; Mohrholz et al., 2014), while tides, coastal currents, and upwelling influence particle resuspension and control sediment redistribution patterns (Lass and Mohrholz, 2005; Monteiro et al., 2005; Inthorn et al., 2006b; Junker et al., 2019).
Sediment cores were retrieved by multicorer (MC-400 Hedrick/Marrs) and were sectioned every 1 cm and frozen in plastic sample bags at −20°C aboard ship. Upon return to the laboratory, the top 5 cm were freeze-dried. After cleaning the utensils with distilled water and polar and apolar organic solvents, the samples were homogenized and combined to yield a 0–5 cm composite surface sediment sample for bulk measurements (TOC, 14C), grain size fractionation, and density fractionation. The 5 cm cut-off was chosen to account for the variable thickness of the floc layer (often >3 cm from the top), and to ensure sample amounts sufficient for the subsequent analyses.
Granulometric analyses were performed to assess the impact of sedimentological parameters on the geochemical distribution patterns on the Namibian shelf, as OC commonly associates with small grain size fractions and resides in low-density particulate matter (e.g., Arnarson and Keil, 2001; Wakeham et al., 2009; Ausín et al., 2021). Three separate sodium polytungstate (SPT-0, NaW; TC-Tungsten Compounds, Germany) heavy liquid solutions were produced to separate the top 5 cm composite samples into four density fraction ranges, i.e., ≤1.6, 1.6–2.0, 2.0–2.5, and >2.5 g cm−3 (Wakeham et al., 2009; Cui et al., 2016). These were obtained by weighing ∼15 g of dried, powdered sediment and vortex mixing with the lowest density solution. Centrifugation for 20 min at 1,600 rpm separated the dense particles from the floating supernatant, which was recovered. The residue was then admixed with the next (higher) density solution and the process was repeated. Each supernatant was filtered (polyethersulfone, 0.2 μm Millipore filters) with Milli-Q type 1 water to remove the remaining salts in each fraction. Finally, the sediment was frozen, freeze-dried and weighed.
Seven grain size fractions (>250, 250–200, 200–125, 125–63, 63–10, 10–2, 2–0.2 μm) were produced by a combined wet sieving-centrifuging-filtering approach on separate aliquots of the same composite sediment samples using Milli-Q type 1 water. Aliquots of ∼15 g of dried, powdered sediment were weighed and, if necessary, clumps formed during storage and preparation were mechanically broken but no ultrasonication was applied to prevent disruption of fragile aggregates. The sand fractions collected on the sieves (up to and including >63 μm) were frozen, freeze-dried, and stored for further use. The material passing through the 63 μm sieve was collected and centrifuged until the supernatant was clean [160 RCF (g), 4 min] in order to separate the clay (0–2 μm) from the silt-sized residual (63–2 μm). The coarse and fine silt fractions were then separated using settling columns at 20°C (Gibbs et al., 1971; Ausín et al., 2021). The clay fraction was filtered (polyethersulfone, 0.2 μm Millipore filters) prior to freezing and storage. All fractions were then freeze-dried and weighed for subsequent analyses.
The purity of each grain size fraction was assessed by performing grain size analysis on the fractions (pre- and post-organic matter removal) using a Malvern Mastersizer 2000 laser-diffraction instrument at the Geological Institute, ETH Zürich. If necessary, shells >2 mm were picked out prior to analysis (McCave et al., 1995; Bao et al., 2019a).
Mineral surface area measurements are used to assess the degree of organic matter-mineral associations and OC loadings on mineral surfaces. A representative aliquot of the bulk sediment sample was heated at 350°C for 12 h and degassed under vacuum at 350°C for 12 h on a Quantachrome FLOVAC degasser to remove organic matter and any bound water, respectively, prior to surface area measurements on a BET Nova4000e using nitrogen. Subject to availability, between 0.31 and 1.2 g (dry wt.) sediment was used, and 5-point N2 adsorption isotherms were measured. To ensure reproducibility and repeatability, the reported values are averages of at least three separate measurements with external standards (σ from 0.0079 to 0.6724, Supplementary Table S4). The high and low surface area standards consist of 82% Aluminium oxide and 18% Silicon (IV) oxide in white pellets (27.46 m2 g−1, 95% reproducibility ± 3.6 m2 g−1) and Alumina in white powder form (2.85 m2 g−1, 95% reproducibility ± 0.20 m2 g−1), respectively.
Prior to measurements of the content and isotopic composition of organic carbon, aliquots (10–20 mg) of bulk sediment, and corresponding grain size and density fractions from each sample were fumigated to remove inorganic carbon with concentrated HCl (37%, 72 h) and subsequently dried over NaOH pellets for 72 h at 70°C. Bulk and fraction-specific radiocarbon measurements (14C/12C) were performed using an elemental analyzer (Vario Micro Cube Elementar, Germany) interfaced to a MICADAS Mini Carbon Dating Accelerator Mass Spectrometry system at the Laboratory of Ion Beam Physics, ETH Zürich (Synal et al., 2007; Welte et al., 2018). The results are reported in F14C without rounding to avoid artificially produced age offsets and errors.
The results are reported in units of F14C following Reimer et al. (2004). The standard deviations of the reported values are better than 10‰ of relative error and the background had values of 0.005 F14C.
To supplement the local measurements from the Namibian margin and develop a global scale perspective, a query of the relevant parameters in well-established databases (Pangaea, NOAA, GSA Repository, MOSAIC) resulted in a local dataset of 17 stations containing distance from shore (Supplementary File S1), a global dataset of 176 stations with grain size-specific TOC (%) and F14C (Supplementary File S2), a global dataset of 20 stations with density fraction-specific TOC (%) (Supplementary File S3), and a global dataset of 617 stations with TOC (%) and surface area (g m−2) (Supplementary File S4). The full references to the datasets are listed with the data.
In the next sections, we briefly revisit prevailing concepts of sediment resuspension and redistribution, in the context of new observations from the Benguela margin. We then propose a new paradigm for sediment deposition, resuspension, redistribution, and burial under low oxygen conditions.
Overall, the bulk OC 14C values reveal a pronounced ageing trend (i.e., decrease in F14C values) in organic matter from nearshore, shallower stations to offshore and deeper stations (Figure 2). The oldest 14C age (F14C, 0.718) is observed 80 nautical miles (nm) offshore, while the youngest 14C age (F14C, 1.021) is 10 nm offshore at 23°S. However, there is no clear correlation between OC (%) and F14C in the bulk surface sediments as both modern and old ages are observed at locations with high concentrations (i.e., >6%) of sedimentary OC. Within grain size fractions, oldest ages (lowest F14C values) are observed at 23°S, 50 and 60 nm, just beyond the inshore belt of high OC (wt%). The age relationship between the grain size fractions changes with distance to shore as sand fractions become older than silt and clay offshore (Figure 3). Within density fractions, the oldest 14C ages are observed in offshore stations and low- and intermediate-density fractions at 20 and 23°S, while youngest ages are reported in intermediate and high-density fractions (Figure 4).
FIGURE 2. OC age (expressed as F14C) illustrates the trend of increasing organic carbon age and decreasing organic carbon content with increasing distance to shore and water depth across the shelf. The interval between 100 and 200 nm contains mostly stations from the depocenter at Lüderitz and down-slope stations (data mostly from Mollenhauer et al., 2003), while stations at the shelf depocenter (60–90 nm) report young OC ages. A clustering effect caused by sampling choice and lab procedure may not be excluded.
FIGURE 3. Grain size-specific F14C data from the Namibian Shelf from inshore to offshore at (A) 20°S and (B) 23°S. Inshore stations are generally characterised by younger ages, while sandy grain size fractions contain old organic carbon in the offshore stations. This trend mirrors the offshore ageing trend in the bulk sediment.
FIGURE 4. F14C values in density fractions, coloured according to distance from shore (nm), symbol type according to shore-perpendicular transect. Density fraction-specific radiocarbon data from the Namibian Shelf reveals older organic carbon in southern and offshore stations (23060), whereas inshore stations tend to be modern (e.g., 20002 & 23010).
A key observation is that organic matter ages in bulk sediment as well as within granulometric fractions increase from inshore to offshore (Figures 2, 3, 4). Potential causes for this increase in OC 14C ages include increased vertical mixing (bioturbation) that entrains older OC from deeper sediment layers or the addition of fossil or pre-aged carbon. Bottom waters on the Namibian inshore shelf (<200 m depth) are largely sub-oxic or anoxic (i.e., O2 <2–3 mg O2 L−1; Diaz, 2016; Mohrholz et al., 2008; Monteiro et al., 2006), which limits bioturbation by infauna and results in negligible sediment mixing (Lagostina, 2019; Sanders, 1968). Moreover, the modern sedimentation rates inshore and over the offshore depocenters are reported to range from 50–150 cm kyr−1 to 5–50 cm yr−1, respectively (Mollenhauer et al., 2002). On longer timescales, sedimentation rates at 25°S and 1992 m water depth (ODP Site 1084) are uniformly high values, reaching up to 20 cm kyr−1 during the Quaternary (Berger et al., 2002; Lazarus et al., 2006). Hence, the 5 cm composite sediment depth samples roughly correspond to 250 years of deposition and vertical mixing of these sediments is not sufficient to explain F14C values as low as 0.7 (Figure 2). Instead, these low F14C values imply inputs of old carbon that has been laterally transported to this location. The major water flow direction on the shelf is north-south, controlled by the Benguela Current and the Angola undercurrent (Figure 1), neither of which is influenced by a large proximal riverine input. In addition, the BUS is dominated by marine productivity [δ13C values −19.7–22.3‰ (Robinson et al., 2002), C:N 6.6-14 (Neumann et al., 2016)], and the adjacent arid landscape of inland Namibia and northern South Africa does not allow for significant land-based plant or soil contributions. While eolian inputs of potentially old OC are possible, these would be most important on a mineralogical level at the proximal (coastal) stations and not the offshore depocenters. Excluding the supply of pre-aged terrestrial organic matter, and consistent with prior interpretations (Inthorn, 2006; Mollenhauer et al., 2003, 2007), we attribute the cause of the observed old ages (low F14C values) of OC in BUS surficial sediments in the offshore depocenters to the advection of pre-aged marine organic carbon derived from sediment redistribution processes.
The timescale of exposure to dissolved oxygen and aerobic decomposition during transport and deposition is considered one of the key controls on sedimentary organic matter preservation (Hartnett et al., 1998; Keil et al., 2004). Given that particulate organic matter arriving at the seafloor is prone to resuspension and lateral redistribution, the degree of oxygen exposure depends not only on the amount of time OM resides in the oxygenated sedimentary (mixed) layer but also on the time it is exposed to oxygen during sediment transport (Keil et al., 1994b). The cumulative oxygen exposure is hypothesized to counterbalance physical protection on millennial timescales, and thus represents an ultimate control on organic matter burial (Hedges and Keil, 1995; Hartnett et al., 1998; Hedges et al., 1999; Keil et al., 2004; Arnarson and Keil, 2007). High OET typically results in the intense degradation of labile organic matter and an accumulation of refractory organic compounds in the residual carbon pool (Middelburg, 1989; Hedges et al., 1999; Hwang et al., 2011). Under these conditions, most of the residual organic matter is present in microaggregates and closely associated with fine-grained mineral surfaces (Hedges et al., 1999; Mayer et al., 2004; Arnarson and Keil, 2007; Saidy et al., 2013).
In contrast, a clear shift in OM-mineral associations and sediment fabric toward large, low-density organic matter-rich particulates can be observed under low oxygen concentrations or short OET (Arnarson and Keil, 2007). Their formation requires measured water movement to cause collisions between the microaggregates but these particles would rapidly disassociate in high-energy conditions or due to mineralisation under protracted O2 exposure. Still, they may persist over much longer time scales under low-oxygen conditions by continuous rebuilding in a dynamic environment characterised by constant interaction between the suspended or temporarily deposited particles. For example, in the BUS with widespread low-oxygen bottom water, we find an abundance of intact but fragile aggregates of old age in the fluffy surface sediment (e.g., F14C ∼0.7) from the slope depocenter, a tell-tale sign of short OET but protracted resuspension-deposition cycles in a weak bottom current system. Their abundance even in sediments underlying oxygenated waters suggests that they are susceptible to mobilization and remain stable during transport, likely because the excess density of organic matter relative to seawater is smaller than that of mineral grains, typically approaching between 1.6 and 2.5 g cm−3 (Richardson and Gardner, 1985; Wakeham et al., 2009). Therefore, the loadings of organic carbon and its influence on the sediment fabric, which in turn is ultimately controlled by the degree of oxygen exposure, appears to be a key controlling factor in how natural sediment responds to shear stress.
For organic matter associated with fine-grained, high-surface-area minerals, OM redistribution is controlled by the hydrodynamic properties of the sedimentary matrix (Wakeham et al., 2009; Bao et al., 2016; Cui et al., 2016), and protracted lateral transport via entrainment in repeated resuspension-deposition loops can result in preferential transport and deposition according to grain size or density (Arnarson and Keil, 2007; Wakeham et al., 2009; Zonneveld et al., 2010; Bao et al., 2016; Wakeham and Canuel, 2016). Over long time-scales, this redistribution and winnowing of fine particles will form hotspots of sorted sediment, where specific (pre-aged) organic compounds accumulate, driven by their preferential association with the mineral grains (Kusch et al., 2010; McCave et al., 2017; Ausín et al., 2022). More importantly, however, the different pre-depositional histories of the translocated organic matter will result in age offsets between the constituents of the same sedimentary layer (Ohkouchi, 2002; Mollenhauer et al., 2005; Kusch et al., 2010; Ausín et al., 2019, 2022).
Transport-induced OM age increases may be a consequence of two factors: the timescale of lateral transport, or the selective preservation of refractory, pre-aged organic matter at the expense of fresh (young) labile organic matter (Bao et al., 2016). Both factors would be expected to play a prominent role in OM transiting or traversing oxygenated water columns, and thus should be considered in the assessment of the 14C ages of sedimentary OM constituents. However, in oxygen-depleted settings such as the Namibian margin, the lower rates of remineralisation allow for a substantial quantity of OC to accumulate in underlying sediments, even after it was subjected to repeated and extensive lateral transport. These transport pathways may exceed >100 nm (>180 km), a fact which is reflected in decreasing F14C ages (increasing age) to the offshore (Figure 2) while OC concentrations remain high. Although a trend to preferential degradation of fresh organic matter is supported by observations of pre-aged biomarkers (Mollenhauer et al., 2008) and sedimentary amino acid compositions (Nagel et al., 2016), an overall larger quantity and more heterogeneous mixture of OM reaches the offshore depocenters (Burdige, 2007; Abshire et al., 2020; LaRowe et al., 2020). These depocenters provide insights into the complex ageing processes at play during transport and burial under low-oxygen conditions. Moreover, the accumulation of OC-rich sediments offshore challenges prevailing views of sedimentological processes that have been shaped by studies of the (oxygenated) modern ocean.
Bulk sediment organic carbon contents (OC%) are highest between 10 and 20 nm offshore (>8%), and generally decrease with increasing distance from shore and with increasing water depth. The 25 and 26°S transects, however, are a notable exception, where a large depocenter of OC-replete sediments lies on the upper slope, 70–90 nm offshore (Figure 2). It is therefore evident that organic matter accumulates both close to the shore and offshore, with a break at intermediate distances. The distribution of OC among grain size fractions is variable. The stations at 20°S indicate that mid-shelf sediments exhibit relatively uniform % OC values for all grain size fractions, while the % OC values at the inshore (2 nm) and offshore (60 nm) stations are lower in the fine sand to silt grain size fractions. At 23 and 25°S, values remain uniformly high (Supplementary Figure S2). OC contents of density fractions are highest in samples with high proportions of lower (≤1.6 g cm−3) and intermediate (1.6–2.0 g cm−3) density fractions. Even though these fractions are gravimetrically less abundant, their high OC concentrations control the bulk organic carbon content. It should be borne in mind, however, that loss of organic matter during the fractionation procedure cannot be precluded as an influence on observations.
The normalization of OC with surface area allows predictions about the OC preservation capacity of an environment (e.g., Blair and Aller, 2012). Corresponding organic carbon “loadings” in Namibian margin sediments expressed as OC contents per unit mineral surface area (OC/SA), are >1 mg OC m−2 (Figure 5) with the highest values in sediments between 2 and 20 nm from shore. This contrasts with values for continental shelf and slope sediments deposited under oxygenated water columns that generally fall in the range of 0.4–1 mg OC m−2 (Figure 5) (Bergamaschi et al., 1997; Bianchi et al., 2018; Keil et al., 1994a; Mayer, 1994; Ransom et al., 1998). Alternatively, high OC/SA ratios can reflect the presence of mineral-free OM particles, or OM-rich aggregates with embedded mineral grains, a fact that is supported by the presence of coarse-grained sediment fractions with high OC (%) contents, particularly in the inner-shelf locations (Figure 6). Irrespective, the high relative proportions of low-density fractions in the OC-rich Namibian margin sediments provide evidence for the abundance of sedimentary particles replete in organic matter and OC loadings exceeding that which can be supported through close mineral associations, hence forming secondary or even tertiary zones on the inner layer of organic matter (Figure 7) (Bock and Mayer, 2000; Sollins et al., 2006; Arnarson and Keil, 2007; Kleber et al., 2007). That outer-layer OM may be subject to greater exchange with the surrounding solution (Sollins et al., 2006; Kleber et al., 2007), and easier to access by microbes and their hydrolytic enzymes (Ransom et al., 1998; Mayer et al., 2004; Burdige, 2007; Bianchi et al., 2018).
FIGURE 5. Comparison of typical OC/SA of marine stations with Namibian shelf samples (this study). The high values of OC (wt%) make the Namibian shelf a prime location for studies on the effects of OC accumulation (Van Der Voort et al., 2021; primary references in Supplementary File S4).
FIGURE 6. Digital microscope and scanning electron microscopy (SEM) imagery of (A) an OC-rich aggregate, (B) organic carbon coating a mineral grain, and (C) SEM imagery of simultaneously occurring pure mineral grains and OM-rich particles from stations offshore 23°S. The organic matter visually coats the grains and associates with pores and other uneven surface structures.
FIGURE 7. Compilation of literature data along with Namibian margin density fractions (this study). (A) Stations from different continental margins plotted according to OC (wt%) per density fraction, coloured for location, with symbol size adjusted for bulk OC content (%). (B) The same stations plotted according to mass (g) per density fraction, coloured for location. Low-density fractions remain important to the organic carbon concentration in the bulk sediment—even in small quantities (Add., Arnarson and Keil, 2001, 2007; Cui et al., 2016; Schreiner et al., 2013; Wakeham et al., 2009; Wakeham and Canuel, 2016).
Taken together, the above information reveals that Namibian margin sediments are rich in organic matter, and that much of this organic matter occurs as discrete fragments or comprises organic-rich aggregates, rather than existing in close association with fine-grained mineral surfaces. Moreover, the persistence of high OC contents and OC/SA ratios in offshore depocenters suggests that these sediment properties are widespread within the BUS. These characteristics have been observed in sediments deposited in other oxygen-deficient settings on continental margins (Arnarson & Keil, 2007; Bianchi et al., 2007; Wakeham and Canuel, 2016).
The hydrodynamic properties of a sediment are not directly influenced by oxygen concentration in the water column, but indirectly, as it controls OC preservation. Organic matter coating on mineral grains destabilizes the surface sediment, as the low-density OM-rich particles remain suspended in a stationary nepheloid layer with a lower threshold of motion, thus increasing porosity (Moore and Keller, 1984) (Supplementary Figure S1). The diverse modes of association of organic matter with mineral surfaces—sorption, aggregation, and loose contact—depend on prevailing conditions (e.g., surface ocean productivity, dissolved oxygen concentrations, the availability and type of mineral surface area, current strength) and contribute directly to the propensity for resuspension of the sediment. When sedimentary organic matter is exposed to oxic degradation, low-density fractions become relatively scarce or ephemeral in nature and the loosely bound organic matter is remineralised rapidly. Hence, the residual organic matter is predominantly associated with the small, high-density mineral grains that follow well-known sedimentary motion criteria. In oxygen-poor conditions, the sediment remains enriched in the low-density aggregates that can carry the associated OC to locations of low energy (depocenters), thus contributing to a characteristic distribution—fine grain sizes offshore or in shielded locations together with low-density fractions coupled to high OC concentrations while shelf breaks and inshore stations are characterised by larger grain sizes. Consequently, organic carbon accumulation in oxygen-deficient conditions may result in different sedimentary dynamics than under oxygenated water columns.
On the Namibian margin, OC distribution follows sediment properties to form nearshore and offshore depocenters (23 and 25°S) where intermediate and low-density particles are abundant in surface sediments as well as fine grain size fractions (Figure 8). Since the depocenters are not directly linked to any of the seasonal surface productivity cells, their creation and preservation requires efficient, protracted lateral transport—made possible by nepheloid layer transport of low-density, OC-rich aggregates. Another feature illustrating the prevalence of lateral transport caused by alongshore currents and their offshore deflection are the distinct sedimentation bands parallel to the shelf break and coast (e.g., a diatomaceous mud-belt in low-energy water depths) (Bremner, 1983; Monteiro et al., 2005). The formation of differential energy regimes due to the interaction of the shelf topography, the internal tides and waves, and the alongshore trade wind stress resulting in the coastal upwelling gives rise to a lower overall grain size at 20 nm offshore and a sharp increase in grain size to the offshore stations, where the turbulent wave energy at the shelf break resuspends small grain sizes and low-density particulate matter into nepheloid layers for lateral redistribution over the slope.
FIGURE 8. (A) Grain size and (B) density fraction distribution on the Namibian shelf along three shore-perpendicular lines from inshore to offshore (in nautical miles), colour-coded for grain size fractions and density fractions, respectively. (A) The sediment is distributed evenly over the shelf with highest bulk sediment sizes offshore 23°S between 50 and 60 nm and inshore 25°S at 5 nm distance from shore. At the inshore and offshore depocenters (23°S 20 and 25°S 80 nm), mostly silty surface sediment is deposited. (B) Density fraction distribution of the same samples is variable, with a notable increase in low-density fractions offshore 20°S and 25°S, while 23°S, the lowest density fraction remains at ∼40% of the total sample.
The hydrodynamic controls acting on the Namibian Shelf may apply to other low-oxygen shelf settings dominated by organic matter input from surface ocean productivity, where grain size is limited to clay-silt fractions and only limited terrestrial input is recorded. However, settings where terrestrial organic matter represents a significant part of sedimentary OC such as in river deltas and estuaries often contain sandy, nearshore sediments with high concentrations of fresh, plant debris (e.g., lignin-rich wood, leaves; Bianchi et al., 2007; Wakeham and Canuel, 2016). On their own, these low-density particles may behave similarly to the large organic aggregates found on the Namibian shelf when subjected to shear stress, but there is very little information present about interparticle interaction of either—cohesion forces are of minor importance in this grain size spectrum (Passow and De La Rocha, 2006; e.g., Simon et al., 2002). However, since aggregates exist in a dynamic state (i.e., they break apart, are reassembled and restructured constantly) whereas individual woody particles remain more or less intact due to their coarser, more rigid structure, it remains an open question if a direct comparison is possible. The presence of the low-density woody materials near-shore raises the possibility that grain size overrides particle density when it comes to controlling particle hydrodynamic properties even at the low-density spectrum (cf., Thomsen and Gust, 2000).
Lateral sediment transport results from a sequence of resuspension and resedimentation processes, the duration and scale of which depends on the transport agent and a range of other external factors including gravity and friction effects, as well as sediment-intrinsic properties (Miller et al., 1977; Cheel, 2005; Southard, 2006; Righetti and Lucarelli, 2007). The transport of sediment in a fluid is often described as a two-phase flow under turbulent or laminar conditions, whose onset of motion depends on the properties of the fluid and the sediment, including physicochemical interactions between individual grains that result in viscous boundary layers (Thomsen and Gust, 2000; Black et al., 2002; Kleeberg and Herzog, 2014). Recent studies refine the physical concepts of sediment transport by focusing on the influence of biological activity on sediment cohesiveness and stability (Gerbersdorf et al., 2008; Tolhurst et al., 2008; Lubarsky et al., 2010; Righetti and Lucarelli, 2010; Fang et al., 2014; Chen et al., 2017c).
Several approaches exist to determine the critical shear stress for incipient motion of non-cohesive sediments. The Shields curve, which represents the probably most well-known entrainment criterion (Shields, 1936) (Figure 9), predicts the conditions under which sediment starts to move using the dimensionless Shields parameter and the particle Reynolds number. Modifications of the initial curve resulted in the extended Shields diagram, a graphical reproduction of the curve, and also considered the influence of bulk density (Mantz, 1977; Miller et al., 1977; Yalin and Karahan, 1979; Israelachvili, 2011). More recently, research has focused on empirical equations that describe the threshold shear stress of sediment movement (Dey, 1999; Miederma, 2010; le Roux, 1991; Soulsby, 1998), as well as recognized importance of biological activity on both sediment stabilization and sediment motion patterns (Tolhurst et al., 2002; Gerbersdorf et al., 2008; Fang et al., 2014; Chen et al., 2017b). Still, the parametrisation and dynamics of cohesive sediments remain a challenge (Mehta, 1988; Berlamont et al., 1993; Yang et al., 2014) and studies that quantify critical shear stress for organic-rich particles and aggregates remain rare (i.e., Beaulieu, 2003; Dzuy and Boger, 1983; Fettweis and Baeye, 2015; Fisher et al., 1983). Most approaches have thus far been based on studies of mineral-dominated sediments in shallow coastal settings or riverine and estuarine environments.
FIGURE 9. Conceptualised Shields diagram modified from Miller et al. (1977) and Thomsen and Gust (2000) showing bed shear stress (τ0) and the Shields criterion (θt) as a function of particle diameter (D50). In green, experiments are reported with low grain densities (ρS = 1.54 g cm−3), in black, Unsöld’s (1984) modification of Shields’ diagram with uncertainty limits (Miller et al., 1977). Both fluid and grain density variation results in a measurable difference in the threshold of motion, with pure quartz grains showing the onset of the particle cohesion effect at ∼30 μm. None of the experiments deal with the effects of organic matter on sediment cohesion and critical shear stress.
According to hydrodynamic principles, coarse (“sortable”) silt and fine sand grain size fractions are the most susceptible to resuspension as their threshold of motion under a given shear stress is relatively low (Shields, 1936; Yalin and Karahan, 1979; Unsöld, 1984). Above this size range, shear stresses required to mobilize coarse-grained sediments are only achieved in highly energetic environments (e.g., wave-influenced settings) (Green, 2016; de Vries et al., 2020), and at lower grain sizes (especially for clay-sized particles), cohesive forces limit the propensity for resuspension (Teisson et al., 1993; Black et al., 2002). Interacting with the grain size control is the overall particle density, a parameter often standardized by using pure mineral samples. However, particles of lower density (e.g., organic matter fragments and organic-rich aggregates) do not necessarily follow the same general rules (Ward, 1969; Fisher et al., 1979; Beheshti and Ataie-Ashtiani, 2008; Righetti and Lucarelli, 2010). High proportions of organic matter-replete particles and aggregates accumulating at the seafloor under low-oxygen bottom water conditions likely influence how the sediment reacts to shear stress given the influence of density on sediment resuspension (Stokes’ Law). Specifically, sediment enriched in organic matter may lower the sediment threshold of motion and facilitate lateral transport, implying that hydrodynamic processes in oxygen-depleted marine settings may be more active than previously considered—potentially resulting in widespread sediment dispersal and the formation of both local and distal depocenters.
Benthic biological activity also influences sediment properties, including response to changes in fluid flow (i.e., bottom shear stress). Bioturbational processes resulting from labile organic matter supply and rapid carbon turnover by benthic epifauna and infauna (e.g., feeding and burrowing by macrofauna) can promote mixing of uppermost sediment layers and the formation of a ventilated upper sediment mixed layer. In contrast, the development of microbial mats can impart cohesion to coarser sediments through excretion of extracellular polymeric substances (EPS) (Tolhurst et al., 2002; Gerbersdorf et al., 2004; Fang et al., 2015), that stabilize the sediment surface to varying degrees (Munn, 2011; Keil and Mayer, 2013). Bioturbation prevents the formation and stability of microbial mats by physically changing the environment and introducing oxygen to a system that would otherwise be devoid of it (Deng et al., 2020). Also, different microbial community structures may impart different physical properties to the sediment through their lifestyle, independent of redox conditions. For example, at locations on the Namibian shelf where bottom water O2 < 0.1 ml L-1 that are characterized by no or at most minimal bioturbation (Levin, 2003; Arning et al., 2008), extensive microbial mats comprised of the single-celled, immotile sulfur-oxidising bacteria, Thiomargarita namibiensis, exhibit relatively little cohesive structure (Schulz et al., 1999; Salman et al., 2013). In contrast, Peruvian margin sediments deposited under similarly oxygen-deficient conditions contain Thioploca, a cellular organism building large filamentous structures that reinforce sediment cohesion (Levin, 2003). These examples serve to show that surficial sediments in oxygen-deficient settings host very dynamic biological communities with different stabilizing effects (Chen X. et al., 2017), whose impact on sediment hydrodynamic behaviour is difficult to parameterize, but may be significant.
Sediment resuspension and redistribution processes are prevalent on continental margins where energetic conditions induced by wave action, tidal motion and local current systems interact to mobilize and translocate sediments. As a consequence of the interplay between different processes, water columns on continental margins often contain layers of enhanced particle abundance, so-called nepheloid layers (Ziervogel et al., 2016; Gardner et al., 2017). Nepheloid layers may be either ephemeral or perennial features and are particularly common in high-productivity or turbulent water masses. They may be relatively stagnant in a stratified water column or serve as conveyor belts for lateral displacement of particulate matter (McCave, 2009; Henrich and Hüneke, 2011). It has been shown that the water chemistry in these particle-rich layers may fluctuate laterally between oxygenated and suboxic conditions depending on the reactivity of the entrained organic matter and resulting microbial activity (Sloth et al., 1996; Blees et al., 2014; Moriarty et al., 2018; Thiele et al., 2018). As such, nepheloid layers contribute significantly to the global flux of biogenic particulate matter to deep depositional sites, augmenting the vertical supply of particulate matter as part of hemipelagic sedimentation (Inthorn et al., 2006c; Honjo et al., 2008; Levin and Sibuet, 2012; Gardner et al., 2018b).
Studies of water column suspended particulate matter and settling particles (via sediment-trap deployments) in oxygenated water columns show that nepheloid layers mostly contain fine-silt to clay-sized particles eroded from the seafloor by bottom currents (Gardner et al., 2017). The cyclic nature of associated resuspension-deposition processes, with repeated exposure to oxygenated bottom waters, leads to significant attenuation in organic matter contents and a highly degraded state of the entrained organic matter (Keil et al., 2004; Inthorn et al., 2006b; McCave, 2009; Bao et al., 2018). Moreover, the extensive time-scales of the along- and across-margin transport lead to temporally aliased organic proxy signatures (e.g., Benthien and Müller, 2000; Ohkouchi et al., 2002), of which the BUS remains an exemplary study site (Mollenhauer et al., 2005; Ausín et al., 2022). Current measurements from Namibian slope bottom waters reveal mean flow velocities high enough to maintain the fine particles in stationary or moving suspension (25 cm s−1) (Mollenhauer et al., 2003; Inthorn et al., 2006a). Although transported organic matter loads in oxygenated water columns may remain significant due to the intrinsic recalcitrance of residual organic matter and physical protection via close mineral associations (Inthorn et al., 2006b; Gardner et al., 2018a; Gardner et al., 2018c), particulate OC concentration generally decreases with transport distance (McCave, 1984; Gardner et al., 2017; Bao et al., 2018, 2019b; Bröder et al., 2018). In this context, the high OC contents (up to ∼10%) yet low corresponding F14C values (∼0.7) of surface sediments in Namibian slope offshore depocenters stand in sharp contrast, highlighting the importance of transport pathways in (partially) oxygen-deficient conditions (Figure 2).
The “Semi-Liquid (or sloppy) Ocean Bottom” (SLOB) hypothesis argues that conventional models are poor analogues for predicting sediment hydrodynamic properties under oxygen-deficient conditions, resulting in impacts on organic matter distribution and burial for two key reasons: First, the abundance of low-density organic matter-replete aggregates results in a depression of the sediment threshold of motion and a higher propensity for resuspension; Second, the resuspended organic matter may be subjected to less degradation during lateral transport under low-oxygen conditions. Both may result in a more efficient and widespread redistribution (and ageing) of organic matter and associated sedimentary matter to distal depositional loci (depocenters) than would be predicted from first principles. Figure 10 depicts the proposed interrelationships between three key sedimentary characteristics—OC loadings (OC/SA), OET, particle density and resuspension propensity—that are linked through the SLOB hypothesis.
FIGURE 10. Conceptual schematic illustrating the different factors interacting with each other to result in flocculent (fluffy) ocean surface sediment and its preferential lateral transport. (A) With increasing oxygen exposure time, the absolute amount of OC in sediment decreases. In addition, the type of association between the OC and the mineral surfaces will change, from free organic debris to aggregates to sorbed OC due to the preferential degradation of less strongly bound/protected OC (cf., Arnarson and Keil, 2007; Bergamaschi et al., 1997; Burdige, 2007). (B) With increasing particulate density, we find that the absolute amount of OC in sediment decreases. On top of that, the type of association changes as well, from free organic matter (low density/high OC/SA) to sorption (high density, low OC/SA) (cf., Arnarson and Keil, 2001; Blair and Aller, 2012). (C) An increase in a sediment’s propensity for resuspension requires high OC/SA ratios, and low-density aggregates, fluffy sediment, or organic debris. Compacted, high-density sediment with little OC%, possibly sorbed to mineral surfaces, will have a lower propensity for resuspension (cf., Jepsen et al., 1997; Mayer, 1994).
Most studies on benthic sediment and carbon dynamics in the modern ocean focus on continental shelf settings overlain by oxygenated waters and existing models that describe sediment resuspension thresholds and hydrodynamic behaviour are developed for similar settings. In these depositional environments, mineral surfaces offer protection of organic matter from degradation, and the hydrodynamic properties of the fine-grained clastic sediments exert primary control on the (re)distribution of organic materials that they host. In contrast, under oxygen-poor conditions, less efficient remineralization leads to an abundance of organic matter in underlying suboxic or anoxic sediment, much of which resides within discrete OM fragments or organic-rich aggregates. This results in the formation of low-density particulate matter characterized by high OC loadings with respect to available mineral surface area. As a result, the surface sediment is highly unconsolidated, exhibiting a flocculent or fluffy consistency, which reduces the governing threshold shear stress of motion and increases the propensity for resuspension and lateral displacement. As the low-density particulate matter and discrete organic particles require lower bottom shear stress for remobilization, the hydrodynamic behaviour of organic matter-rich sedimentary particles may be quite different compared to their lower OC-higher density equivalents deposited under more oxygenated conditions (Supplementary Figure S5).
Although low-density, fluffy, surface layers of marine sediments that exhibit increased propensity for resuspension are not unique to low-oxygen environments, and bottom nepheloid layers occur widely (Thomsen and Gust, 2000; Gerbersdorf et al., 2004; Kleeberg and Herzog, 2014), particle-associated organic matter is usually subject to degradation during protracted lateral transport in oxic water columns, limiting its broader-scale influence. In contrast, low-density organic-rich particulate matter entrained in oxygen-deficient waters may remain buoyant and subject to large-scale displacement with minimal OM attenuation during translocation (Dang and Lovell, 2016), leading to accumulation of OC-rich sediments in distal depocenters (e.g., Namibian Shelf).
The SLOB-hypothesis implies that we cannot directly apply our understanding of the impact of hydrodynamic processes on sedimentary organic matter deposited under oxygenated conditions to those accumulating under anoxic or suboxic conditions. This is especially true where oxic sediments lack a fluffy (sloppy) sediment-water interface enriched in low-density particulate matter. Furthermore, SLOB sediments may not adhere to the general two-layered critical shear stress model, which differentiates between the fluffy surface and more consolidated sediment layers below (Beier et al., 1991; Thomsen and Gust, 2000; Beaulieu, 2003; Kleeberg and Herzog, 2014) owing to its greater propensity for mobilization and translocation at lower shear stresses. Consequently, lateral transport of organic matter on sub-oxic continental shelves or in fully anoxic ocean basins may be more widespread, and may be induced under less energetic conditions, than previously assumed. As surficial sediments are most enriched in organic matter, their mobilization may play a key role in the re-supply of nutrients to the overlying water column, impacting benthic communities, and benthic-pelagic coupling.
We suggest new approaches are needed to assess the role of surface sediment texture and consequently, the resuspension of propensity of fluffy, unconsolidated sediments in oxygen deficient settings on organic matter burial. Moreover, the effects of sediment properties on organic matter redistribution and preservation potential need to be examined, including but not limited to the structural changes imparted by biofilms and their resistance to disruption, the effective protection potential of surface area sorption for labile compounds, the importance of varying sedimentation rates on sediment texture, and the function and mechanism of repeated resuspension/deposition cycles in lateral transport of OC.
As oxygen deficient zones are predicted to spread and intensify with climate change (e.g., Diaz and Rosenberg, 2008; Breitburg et al., 2018), the effect of the accompanying increase of sedimentary OC and its subsequent resuspension is yet unknown but possibly significant due to its varied impact on biota, and the biological carbon pump on continental margins and in the deep ocean (Keil, 2017). Will planktic communities shift in response to intensified stratification and diminishing oxygen concentrations? How will benthic communities respond to low oxygen concentrations? A strong decrease in bioturbation intensity and shift in dominant bioturbating fauna has been predicted for upcoming decades (Bianchi et al., 2021). Because macrofaunal reworking and ventilation is a key driver of microbial community structure in continental shelf sediments (Chen X. et al., 2017; Deng et al., 2020), this decrease in bioturbation intensity will likely cause major shifts in surface sedimentary microbial communities, including changes in dominance from aerobic and O2-tolerant to strictly anaerobic taxa. These community and physiological shifts will likely be reflected in changes in catabolic rates, metabolic pathways, and the structure of sediment microbial food webs. Yet, little is known about potential feedbacks, associated with these processes, although they may be of key importance to our understanding of marine OC burial (Jessen et al., 2017).
The hypothesis that organic matter-rich sediment requires lower shear stress for resuspension and redistribution in low-oxygen or anoxic water bodies has potentially important implications for paleoceanographic interpretation of sedimentary records which generally assume direct (vertical) transport of proxy signals to the sea floor. Lateral transport and translocation of pre-aged organic matter may lead to both spatial and temporal aliasing of proxy records in sediments (Ohkouchi et al., 2002; Mollenhauer et al., 2007; Ausin et al., 2019; Ausín et al., 2022).
The phenomenon of increased sediment mobility due to organic matter-enrichment may be particularly relevant during past intervals when the oceans experienced large-scale anoxia, such as the Cretaceous Oceanic Anoxic Events (Schlanger and Jenkyns, 1976; Degens et al., 1986; Emeis, 1987; Tyson and Pearson, 1991; Jenkyns, 2010), the Mediterranean Sapropels which formed during oceanic anoxic events associated with Pleistocene glacial-interglacial cycles (Grant et al., 2016), or during much earlier in Earth’s history (i.e., Archaean, early/mid Proterozoic) when ocean basins were anoxic (Lyons et al., 2014). Even apparently quiescent depositional conditions might have been conducive to mobilization of low-density OC-rich sediments. Presently, however, we do not understand the processes well enough to apply them to past depositional environments or predict how future oceans will respond to higher temperatures and surface productivity. A combination of approaches, for example using different proxies as well as experiments, is required to account for these issues, and to assess their impact on the sedimentary OC record.
The datasets presented in this study can be found in online repositories. The names of the repository/repositories and accession number(s) can be found in the article/Supplementary Material.
While the original working hypothesis was formulated by TE and TB, EB conceived the project, interpreted the empirical the findings and wrote the manuscript with equal contribution from all co-authors. EB and NH performed the lab work and gathered the data. DL is the cruise leader on the R/V Mirabilis and collected the samples. ML also contributed to the writing of the manuscript.
This project was funded by a grant from ETH Zurich (ETH-07 19-1: The Semi-Liquid Ocean Bottom (SLOB) Hypothesis). Open access funding provided by ETH Zurich.
The authors declare that the research was conducted in the absence of any commercial or financial relationships that could be construed as a potential conflict of interest.
All claims expressed in this article are solely those of the authors and do not necessarily represent those of their affiliated organizations, or those of the publisher, the editors and the reviewers. Any product that may be evaluated in this article, or claim that may be made by its manufacturer, is not guaranteed or endorsed by the publisher.
The authors want to thank the Regional Graduate Network in Oceanography (RGNO), a SCOR-supported cooperative Research and Capacity Building Initiative in southern Africa for offering the opportunity for repeated field work and the crew of R/V Mirabilis for supporting our sampling trips. We would also like to thank the local scientists from the Ministry of Fisheries and Marine Resources (MFMR) including but not limited to Anja van der Plas and Margit Wilhelm. Also, we thank Daniel Montluçon for sharing his expertise in discussions, field work, and lab work. We thank Duncan Dale for sample preparation prior to measurements on the AMS and finally, we acknowledge Sarah Paradis for her work on the MOSAIC database platform.
The Supplementary Material for this article can be found online at: https://www.frontiersin.org/articles/10.3389/feart.2022.886395/full#supplementary-material
Abshire, M. L., Owens, J. D., Cofrancesco, J., Inthorn, M., and Riedinger, N. (2020). Geochemical Signatures of Redepositional Environments: The Namibian continental Margin. Mar. Geology. 429, 106316. doi:10.1016/j.margeo.2020.106316
Armstrong, R. A., Lee, C., Hedges, J. I., Honjo, S., and Wakeham, S. G. (2002). A New, Mechanistic Model for Organic Carbon Fluxes in the Ocean Based on the Quantitative Association of POC with Ballast Mineralsb. Deep. Res. Part. Top. Stud. Oceanogr. 49 (1–3), 219–236. doi:10.1016/S0967-0645(01)00101-1
Arnarson, T. S., and Keil, R. G. (2007). Changes in Organic Matter-mineral Interactions for marine Sediments with Varying Oxygen Exposure Times. Geochimica et Cosmochimica Acta 71 (14), 3545–3556. doi:10.1016/j.gca.2007.04.027
Arnarson, T. S., and Keil, R. G. (2001). Organic-mineral Interactions in marine Sediments Studied Using Density Fractionation and X-ray Photoelectron Spectroscopy. Org. Geochem. 32 (12), 1401–1415. doi:10.1016/S0146-6380(01)00114-0
Arndt, S., Jørgensen, B. B., LaRowe, D. E., Middelburg, J. J., Pancost, R. D., and Regnier, P. (2013). Quantifying the Degradation of Organic Matter in marine Sediments: A Review and Synthesis. Earth-Science Rev. 123, 53–86. doi:10.1016/j.earscirev.2013.02.008
Arning, E. T., Birgel, D., Schulz-Vogt, H. N., Holmkvist, L., J⊘rgensen, B. B., Larson, A., et al. (2008). Lipid Biomarker Patterns of Phosphogenic Sediments from Upwelling Regions. Geomicrobiology J. 25 (2), 69–82. doi:10.1080/01490450801934854
Ausín, B., Bruni, E., Haghipour, N., Welte, C., Bernasconi, S. M., and Eglinton, T. I. (2021). Controls on the Abundance, Provenance and Age of Organic Carbon Buried in continental Margin Sediments. Earth Planet. Sci. Lett. 558, 116759. doi:10.1016/j.epsl.2021.116759
Ausín, B., Haghipour, N., Bruni, E., and Eglinton, T. (2022). The Influence of Lateral Transport on Sedimentary Alkenone Paleoproxy Signals. Biogeosciences 19 (3), 613–627. doi:10.5194/bg-19-613-2022
Ausín, B., Haghipour, N., Wacker, L., Voelker, A. H. L., Hodell, D., Magill, C., et al. (2019). Radiocarbon Age Offsets between Two Surface Dwelling Planktonic Foraminifera Species during Abrupt Climate Events in the SW Iberian Margin. Paleoceanography and Paleoclimatology 34 (1), 63–78. doi:10.1029/2018PA003490
Ausín, B., Magill, C., Haghipour, N., Fernández, Á., Wacker, L., Hodell, D., et al. (2019). (In)coherent Multiproxy Signals in marine Sediments: Implications for High-Resolution Paleoclimate Reconstruction. Earth Planet. Sci. Lett. 515, 38–46. doi:10.3929/ETHZ-B-00022561610.1016/j.epsl.2019.03.003
Bailey, G. W. (1991). Organic Carbon Flux and Development of Oxygen Deficiency on the Modern Benguela Continential Shelf South of 22°S: Spatial and Temporal Variability. Geol. Soc. Lond. Spec. Publications 58 (1), 171–183. doi:10.1144/gsl.sp.1991.058.01.12
Bao, R., Blattmann, T. M., McIntyre, C., Zhao, M., and Eglinton, T. I. (2019a). Relationships between Grain Size and Organic Carbon 14C Heterogeneity in continental Margin Sediments. Earth Planet. Sci. Lett. 505 (January), 76–85. doi:10.1016/j.epsl.2018.10.013
Bao, R., McIntyre, C., Zhao, M., Zhu, C., Kao, S.-J., and Eglinton, T. I. (2016). Widespread Dispersal and Aging of Organic Carbon in Shallow Marginal Seas. Geology 44 (10), 791–794. doi:10.1130/G37948.1
Bao, R., van der Voort, T. S., Zhao, M., Guo, X., Montluçon, D. B., McIntyre, C., et al. (2018). Influence of Hydrodynamic Processes on the Fate of Sedimentary Organic Matter on Continental Margins. Glob. Biogeochem. Cycles 32 (9), 1420–1432. doi:10.1029/2018GB005921
Bao, R., Zhao, M., McNichol, A., Galy, V., McIntyre, C., Haghipour, N., et al. (2019b). Temporal Constraints on Lateral Organic Matter Transport along a Coastal Mud belt. Org. Geochem. 128 (February), 86–93. doi:10.1016/j.orggeochem.2019.01.007
Barlow, R., Lamont, T., Mitchell-Innes, B., Lucas, M., and Thomalla, S. (2009). Primary Production in the Benguela Ecosystem, 1999-2002. Afr. J. Mar. Sci. 31 (1), 97–101. doi:10.2989/AJMS.2009.31.1.9.780
Beaulieu, S. E. (2003). Resuspension of Phytodetritus from the Sea Floor: A Laboratory Flume Study. Limnol. Oceanogr. 48 (3), 1235–1244. doi:10.4319/lo.2003.48.3.1235
Beheshti, A. A., and Ataie-Ashtiani, B. (2008). Analysis of Threshold and Incipient Conditions for Sediment Movement. Coastal Eng. 55 (5), 423–430. doi:10.1016/j.coastaleng.2008.01.003
Beier, J. A., Wakeham, S. G., Pilskaln, C. H., and Honjo, S. (1991). Enrichment in Saturated Compounds of Black Sea Interfacial Sediment. Nature 351 (6328), 642–644. doi:10.1038/351642a0
Benthien, A., and Müller, P. J. (2000). Anomalously Low Alkenone Temperatures Caused by Lateral Particle and Sediment Transport in the Malvinas Current Region, Western Argentine Basin. Deep Sea Res. Oceanographic Res. Pap. 47 (12), 2369–2393. doi:10.1016/S0967-0637(00)00030-3
Bergamaschi, B. A., Tsamakis, E., Keil, R. G., Eglinton, T. I., Montluçon, D. B., and Hedges, J. I. (1997). The Effect of Grain Size and Surface Area on Organic Matter, Lignin and Carbohydrate Concentration, and Molecular Compositions in Peru Margin Sediments. Geochimica et Cosmochimica Acta 61 (6), 1247–1260. doi:10.1016/s0016-7037(96)00394-8
Berger, W. H., Lange, C. B., and Wefer, G. (2002). Upwelling History of the Benguela-Namibia System: A Synthesis of Leg 175 Results. Proc. Ocean Drill. Progr. Sci. Results 175, 1–53. doi:10.2973/odp.proc.sr.175.235.2002
Berlamont, J., Ockenden, M., Toorman, E., and Winterwerp, J. (1993). The Characterisation of Cohesive Sediment Properties. Coast. Eng. 21 (1–3), 105–128. doi:10.1016/0378-3839(93)90047-C
Berner, R. A. (2001). GEOCARB III: A Revised Model of Atmospheric CO2 over Phanerozoic Time. Am. J. Sci. 301 (2), 182–204. doi:10.2475/ajs.301.2.182
Bianchi, T. S., Aller, R. C., Atwood, T. B., Brown, C. J., Buatois, L. A., Levin, L. A., et al. (2021). What Global Biogeochemical Consequences Will marine Animal-Sediment Interactions Have during Climate Change? Elem. Sci. Anthr. 9 (1). doi:10.1525/elementa.2020.00180
Bianchi, T. S., Cui, X., Blair, N. E., Burdige, D. J., Eglinton, T. I., and Galy, V. (2018). Centers of Organic Carbon Burial and Oxidation at the Land-Ocean Interface. Org. Geochem. 115, 138–155. doi:10.1016/j.orggeochem.2017.09.008
Bianchi, T. S., Galler, J. J., and Allison, M. A. (2007). Hydrodynamic Sorting and Transport of Terrestrially Derived Organic Carbon in Sediments of the Mississippi and Atchafalaya Rivers. Estuarine, Coastal Shelf Sci. 73 (1–2), 211–222. doi:10.1016/j.ecss.2007.01.004
Black, K. S., Tolhurst, T. J., Paterson, D. M., and Hagerthey, S. E. (2002). Working with Natural Cohesive Sediments. J. Hydraul. Eng. 128 (1), 2–8. doi:10.1061/(ASCE)0733-9429(2002)128:1(2)
Blair, N. E., and Aller, R. C. (2012). The Fate of Terrestrial Organic Carbon in the Marine Environment. Annu. Rev. Mar. Sci. 4 (1), 401–423. doi:10.1146/annurev-marine-120709-142717
Blees, J., Niemann, H., Wenk, C. B., Zopfi, J., Schubert, C. J., Jenzer, J. S., et al. (2014). Bacterial Methanotrophs Drive the Formation of a Seasonal Anoxic Benthic Nepheloid Layer in an alpine lake. Limnol. Oceanogr. 59 (4), 1410–1420. doi:10.4319/lo.2014.59.4.1410
Bock, M. J., and Mayer, L. M. (2000). Mesodensity Organo–clay Associations in a Near-Shore Sediment. Mar. Geol. 163 (1–4), 65–75. doi:10.1016/S0025-3227(99)00105-X
Breitburg, D., Levin, L. A., Oschlies, A., Grégoire, M., Chavez, F. P., Conley, D. J., et al. (2018). Declining Oxygen in the Global Ocean and Coastal Waters. Science 359 (6371), eaam7240. doi:10.1126/science.aam7240
Bremner, J. M. (1983). “Biogenic Sediments on the South West African (Namibian) Continental Margin,” in Coastal Upwelling its Sediment Record. Editors J. Thiede, and E. Suess (Boston, MA: Springer US), 73–103. doi:10.1007/978-1-4613-3709-6_4
Bröder, L., Tesi, T., Andersson, A., Semiletov, I., and Gustafsson, Ö. (2018). Bounding Cross-Shelf Transport Time and Degradation in Siberian-Arctic Land-Ocean Carbon Transfer. Nat. Commun. 9 (1). doi:10.1038/s41467-018-03192-1
Burdige, D. J. (2007). Preservation of Organic Matter in Marine Sediments: Controls, Mechanisms, and an Imbalance in Sediment Organic Carbon Budgets? Chem. Rev. 107 (2), 467–485. doi:10.1021/cr050347q
Cheel, R. J. (2005). Fluid Flow and Sediment Transport, in Introduction to Clastic Sedimentology. St. Catharines, Ontario, Canada. [online] Available from: https://brocku.ca/sedimentology/SedNotes/Chap4.pdf.5573
Chen, X., Andersen, T. J., Morono, Y., Inagaki, F., Jørgensen, B. B., and Lever, M. A. (2017a). Bioturbation as a Key Driver behind the Dominance of Bacteria over Archaea in Near-Surface Sediment. Sci. Rep. 7 (1), 2400. doi:10.1038/s41598-017-02295-x
Chen, X. D., Zhang, C. K., Paterson, D. M., Thompson, C. E. L., Townend, I. H., Gong, Z., et al. (2017b). Hindered Erosion: The Biological Mediation of Noncohesive Sediment Behavior. Water Resour. Res. 53 (6), 4787–4801. doi:10.1002/2016WR020105
Chen, X. D., Zhang, C. K., Zhou, Z., Gong, Z., Zhou, J. J., Tao, J. F., et al. (2017c). Stabilizing Effects of Bacterial Biofilms: EPS Penetration and Redistribution of Bed Stability Down the Sediment Profile. J. Geophys. Res. Biogeosci. 122 (12), 3113–3125. doi:10.1002/2017JG004050
Cui, X., Bianchi, T. S., Hutchings, J. A., Savage, C., and Curtis, J. H. (2016). Partitioning of Organic Carbon Among Density Fractions in Surface Sediments of Fiordland, New Zealand. J. Geophys. Res. Biogeosci. 121 (3), 1016–1031. doi:10.1002/2015JG003225
Dang, H., and Lovell, C. R. (2016). Microbial Surface Colonization and Biofilm Development in Marine Environments. Microbiol. Mol. Biol. Rev. 80 (1), 91–138. doi:10.1128/MMBR.00037-15
de Vries, S., Wengrove, M., and Bosboom, J. (2020). “Marine Sediment Transport,” in Sandy Beach Morphodynamics (London, United Kingdom: Elsevier), 187–212. doi:10.1016/b978-0-08-102927-5.00009-6
Degens, E. T., Emeis, K.-C., Mycke, B., and Wiesner, M. G. (1986). “Turbidites, the Principal Mechanism Yielding Black Shales in the Early Deep Atlantic Ocean,” in North Atlantic Paleoceanography. Editors C. P. Summerhayes, and N. J. Shackleton (London: Geological Society Special Publication), 21, 361–376. doi:10.1144/gsl.sp.1986.021.01.26
Deng, L., Bölsterli, D., Kristensen, E., Meile, C., Su, C.-C., Bernasconi, S. M., et al. (2020). Macrofaunal Control of Microbial Community Structure in continental Margin Sediments. Proc. Natl. Acad. Sci. U.S.A. 117 (27), 15911–15922. doi:10.1073/pnas.1917494117
Dey, S. (1999). Sediment Threshold. Appl. Math. Model. 23, 399–417. doi:10.1007/978-3-642-19062-9_410.1016/s0307-904x(98)10081-1
Diaz, R. J. (2016). “Anoxia, Hypoxia, and Dead Zones,”. Editor M. J. Kennish (Dordrecht, Dordrecht.: Springer), 30, 19–29. doi:10.1007/978-94-017-8801-4_82Curr. Biol.
Diaz, R. J., and Rosenberg, R. (2008). Spreading Dead Zones and Consequences for Marine Ecosystems. Science 321 (5891), 926–929. doi:10.1126/science.1156401
Dzuy, N. Q., and Boger, D. V. (1983). Yield Stress Measurement for Concentrated Suspensions. J. Rheology 27 (4), 321–349. doi:10.1122/1.549709
Emeis, K.-C. (1987). Cretaceous Black Shales of the Suoh Atlantic Ocean: The Role and Origin of Recycled Organic Matter. Mitteilungen Aus Dem Geol. Inst. der Univ. Hambg. 62, 209–232.
Fang, H., Fazeli, M., Cheng, W., Huang, L., and Hu, H. (2015). Biostabilization and Transport of Cohesive Sediment Deposits in the Three Gorges Reservoir. PLoS One 10 (11), e0142673. doi:10.1371/journal.pone.0142673
Fang, H., Shang, Q., Chen, M., and He, G. (2014). Changes in the Critical Erosion Velocity for Sediment Colonized by Biofilm. Sedimentology 61 (3), 648–659. doi:10.1111/sed.12065
Fennel, W. (1999). Theory of the Benguela Upwelling System. J. Phys. Oceanogr. 29 (2), 177–190. doi:10.1175/1520-0485(1999)029<0177:TOTBUS>2.0.CO;2
Fettweis, M., and Baeye, M. (2015). Seasonal Variation in Concentration, Size, and Settling Velocity of Muddy marine Flocs in the Benthic Boundary Layer. J. Geophys. Res. Oceans 120 (8), 5648–5667. doi:10.1002/2014JC010644
Fisher, J. S., Pickral, J., and Odum, W. E. (1979). Organic Detritus Particles: Initiation of Motion Criteria. Limnol. Oceanogr. 24 (3), 529–532. doi:10.4319/lo.1979.24.3.0529
Fisher, J. S., Sill, B. L., and Clark, D. F. (1983). Organic Detritus Particles: Initiation of Motion Criteria on Sand and Gravel Beds. Water Resour. Res. 19 (6), 1627–1631. doi:10.1029/wr019i006p01627
Francois, R., Honjo, S., Krishfield, R., and Manganini, S. (2002). Factors Controlling the Flux of Organic Carbon to the Bathypelagic Zone of the Ocean. Glob. Biogeochem. Cycles 16 (4), 34. doi:10.1029/2001GB001722
Gardner, W. D., Jo Richardson, M., Mishonov, A. V., and Biscaye, P. E. (2018c). Global Comparison of Benthic Nepheloid Layers Based on 52 Years of Nephelometer and Transmissometer Measurements. Prog. Oceanogr. 168 (May), 100–111. doi:10.1016/j.pocean.2018.09.008
Gardner, W. D., Mishonov, A. V., and Richardson, M. J. (2018a). Decadal Comparisons of Particulate Matter in Repeat Transects in the Atlantic, Pacific, and Indian Ocean Basins. Geophys. Res. Lett. 45 (1), 277–286. doi:10.1002/2017GL076571
Gardner, W. D., Richardson, M. J., and Mishonov, A. V. (2018b). Global Assessment of Benthic Nepheloid Layers and Linkage with Upper Ocean Dynamics. Earth Planet. Sci. Lett. 482, 126–134. doi:10.1016/j.epsl.2017.11.008
Gardner, W. D., Tucholke, B. E., Richardson, M. J., and Biscaye, P. E. (2017). Benthic Storms, Nepheloid Layers, and Linkage with Upper Ocean Dynamics in the Western North Atlantic. Mar. Geology. 385, 304–327. doi:10.1016/j.margeo.2016.12.012
Gerbersdorf, S., Meyercordt, J., and Meyer-Reil, L.-A. (2004). Microphytobenthic Primary Production within the Flocculent Layer, its Fractions and Aggregates, Studied in Two Shallow Baltic Estuaries of Different Eutrophic Status. J. Exp. Mar. Bio. Ecol. 307 (1), 47–72. doi:10.1016/j.jembe.2004.01.020
Gerbersdorf, S. U., Jancke, T., Westrich, B., and Paterson, D. M. (2008). Microbial Stabilization of Riverine Sediments by Extracellular Polymeric Substances. Geobiology 6 (1), 57–69. doi:10.1111/j.1472-4669.2007.00120.x
Gibbs, R. J., Matthews, M. D., and Link, D. A. (1971). The Relationship between Sphere Size and Settling Velocity. J. Sediment. Petrol. 41 (1), 7–18. doi:10.1306/74D721D0-2B21-11D7-8648000102C1865D
Grant, K. M., Grimm, R., Mikolajewicz, U., Marino, G., Ziegler, M., and Rohling, E. J. (2016). The Timing of Mediterranean Sapropel Deposition Relative to Insolation, Sea-Level and African Monsoon Changes. Quat. Sci. Rev. 140, 125–141. doi:10.1016/j.quascirev.2016.03.026
Green, M. O. (2016). “Wave-Driven Sediment Resuspension,” in Encyclopedia of Earth Sciences Series. Editor M. J. Kennish (Dordrecht.: Springer Netherlands), 734–737.
Hartnett, H. E., Keil, R. G., Hedges, J. I., and Devol, A. H. (1998). Influence of Oxygen Exposure Time on Organic Carbon Preservation in continental Margin Sediments. Nature 391 (6667), 572–574. doi:10.1038/35351
Hedges, J. I., Hu, F. S., Devol, A. H., Hartnett, H. E., Tsamakis, E., and Keil, R. G. (1999). Sedimentary Organic Matter Preservation: A Test for Selective Degradation under Oxic Conditions. Am. J. Sci. 299 (7–9), 529–555. doi:10.2475/ajs.299.7-9.529
Hedges, J. I., and Keil, R. G. (1995). Sedimentary Organic Matter Preservation: an Assessment and Speculative Synthesis. Mar. Chem. 49 (2–3), 81–115. doi:10.1016/0304-4203(95)00008-F
Henrich, R., and Hüneke, H. (2011). Hemipelagic Advection and Periplatform Sedimentation. Dev. Sedimentol. 63 (C), 353–396. doi:10.1016/B978-0-444-53000-4.00005-6
Honjo, S., Manganini, S. J., Krishfield, R. A., and Francois, R. (2008). Particulate Organic Carbon Fluxes to the Ocean interior and Factors Controlling the Biological Pump: A Synthesis of Global Sediment Trap Programs since 1983. Prog. Oceanogr. 76 (3), 217–285. doi:10.1016/j.pocean.2007.11.003
Hwang, K.-Y., Kim, H.-S., and Hwang, I. (2011). Effect of Resuspension on the Release of Heavy Metals and Water Chemistry in Anoxic and Oxic Sediments. Clean. Soil Air Water 39 (10), 908–915. doi:10.1002/clen.201000417
Ingalls, A. E., Aller, R. C., Lee, C., and Wakeham, S. G. (2004). Organic Matter Diagenesis in Shallow Water Carbonate Sediments. Geochimica et Cosmochimica Acta 68 (21), 4363–4379. doi:10.1016/j.gca.2004.01.002
Inthorn, M. (2006). Lateral Particle Transport in Nepheloid Layers-A Key Factor for Organic Matter Distribution and Quality in the Benguela High-Productivity Area, 124. Bremen: Berichte, Fachbereich Geowissenschaften, Univ.244
Inthorn, M., Mohrholz, V., and Zabel, M. (2006c). Nepheloid Layer Distribution in the Benguela Upwelling Area Offshore Namibia. Deep Sea Res. Part Oceanographic Res. Pap. 53 (8), 1423–1438. doi:10.1016/j.dsr.2006.06.004
Inthorn, M., Rutgers van der Loeff, M., and Zabel, M. (2006a). A Study of Particle Exchange at the Sediment-Water Interface in the Benguela Upwelling Area Based on 234Th/238U Disequilibrium. Deep Sea Res. Part Oceanographic Res. Pap. 53 (11), 1742–1761. doi:10.1016/j.dsr.2006.08.004
Inthorn, M., Wagner, T., Scheeder, G., and Zabel, M. (2006b). Lateral Transport Controls Distribution, Quality, and Burial of Organic Matter along continental Slopes in High-Productivity Areas. Geol 34 (3), 205–208. doi:10.1130/G22153.1
Israelachvili, J. N. (2011). Intermolecular and Surface Forces. 3rd ed. Oxford, United Kingdom: Elsevier Science Publishers.
Iversen, M. H., and Ploug, H. (2010). Ballast Minerals and the Sinking Carbon Flux in the Ocean: Carbon-specific Respiration Rates and Sinking Velocities of Macroscopic Organic Aggregates (marine Snow). Biogeosciences Discuss. 7 (3), 3335–3364. doi:10.5194/bgd-7-3335-2010
Jahnke, R. A. (2009). “Organic Carbon Cycling in Continental Margin Environments,” in Encyclopedia of Ocean Sciences (New York: Elsevier), 252–260. doi:10.1016/b978-012374473-9.00644-5
Jenkyns, H. C. (2010). Geochemistry of Oceanic Anoxic Events. Geochem. Geophys. Geosyst. 11 (3), a–n. doi:10.1029/2009GC002788
Jepsen, R., Roberts, J., and Lick, W. (1997). Effects of Bulk Density on Sediment Erosion Rates. Air Soil Pollut. 99 (1–4), 21–31. doi:10.1023/A:101835562607010.1007/978-94-011-5552-6_3
Jessen, G. L., Lichtschlag, A., Ramette, A., Pantoja, S., Rossel, P. E., Schubert, C. J., et al. (2017). Hypoxia Causes Preservation of Labile Organic Matter and Changes Seafloor Microbial Community Composition (Black Sea). Sci. Adv. 3 (2), e1601897. doi:10.1126/sciadv.1601897
Junker, T., Mohrholz, V., Schmidt, M., Siegfried, L., and van der Plas, A. (2019). Coastal Trapped Wave Propagation along the Southwest African Shelf as Revealed by Moored Observations. J. Phys. Oceanogr. 49 (3), 851–866. doi:10.1175/JPO-D-18-0046.1
Junker, T., Mohrholz, V., Siegfried, L., and van der Plas, A. (2017). Seasonal to Interannual Variability of Water Mass Characteristics and Currents on the Namibian Shelf. J. Mar. Syst. 165, 36–46. doi:10.1016/j.jmarsys.2016.09.003
Keeling, R. F., Körtzinger, A., and Gruber, N. (2010). Ocean Deoxygenation in a Warming World. Annu. Rev. Mar. Sci. 2 (1), 199–229. doi:10.1146/annurev.marine.010908.163855
Keil, R. (2017). Anthropogenic Forcing of Carbonate and Organic Carbon Preservation in Marine Sediments. Annu. Rev. Mar. Sci. 9 (1), 151–172. doi:10.1146/annurev-marine-010816-060724
Keil, R. G., Dickens, A. F., Arnarson, T., Nunn, B. L., and Devol, A. H. (2004). What Is the Oxygen Exposure Time of Laterally Transported Organic Matter along the Washington Margin? Mar. Chem. 92 (1-4 SPEC. ISS.), 157–165. doi:10.1016/j.marchem.2004.06.024
Keil, R. G., and Mayer, L. M. (2013). Mineral Matrices and Organic Matter. 2nd ed. Oxford, United Kingdom: Elsevier.
Keil, R. G., Montluçon, D. B., Prahl, F. G., and Hedges, J. I. (1994b). Sorptive Preservation of Labile Organic Matter in marine Sediments. Nature 370 (January 19946490), 549–552. doi:10.1038/370549a0
Keil, R. G., Tsamakis, E., Fuh, C. B., Giddings, J. C., and Hedges, J. I. (1994a). Mineralogical and Textural Controls on the Organic Composition of Coastal marine Sediments: Hydrodynamic Separation Using SPLITT-Fractionation. Geochimica et Cosmochimica Acta 58 (2), 879–893. doi:10.1016/0016-7037(94)90512-6
Klaas, C., and Archer, D. E. (2002). Association of Sinking Organic Matter with Various Types of mineral Ballast in the Deep Sea: Implications for the Rain Ratio. Glob. Biogeochem. Cycles 16 (4), 63. doi:10.1029/2001GB001765
Kleber, M., Sollins, P., and Sutton, R. (2007). A Conceptual Model of Organo-mineral Interactions in Soils: Self-Assembly of Organic Molecular Fragments into Zonal Structures on mineral Surfaces. Biogeochemistry 85 (1), 9–24. doi:10.1007/s10533-007-9103-5
Kleeberg, A., and Herzog, C. (2014). Sediment Microstructure and Resuspension Behavior Depend on Each Other. Biogeochemistry 119 (1–3), 199–213. doi:10.1007/s10533-014-9959-0
Kusch, S., Eglinton, T. I., Mix, A. C., and Mollenhauer, G. (2010). Timescales of Lateral Sediment Transport in the Panama Basin as Revealed by Radiocarbon Ages of Alkenones, Total Organic Carbon and Foraminifera. Earth Planet. Sci. Lett. 290 (3–4), 340–350. doi:10.1016/j.epsl.2009.12.030
Lagostina, L. (2019). Temperature, Redox Conditions and Organic Matter Chemical Composition as Microbial Community Drivers in marine Sediments. Zürich: ETH Zürich.
Lahajnar, N., Mohrholz, V., Angenendt, S., Brust-Möbius, J., Bucholz, F., Chikwililwa, C., et al. (2014). Geochemistry and Ecology of the Namibian Upwelling System NAMUFIL: Namibian Upwelling Filament Study.
LaRowe, D. E., Arndt, S., Bradley, J. A., Estes, E. R., Hoarfrost, A., Lang, S. Q., et al. (2020). The Fate of Organic Carbon in marine Sediments - New Insights from Recent Data and Analysis. Earth-Science Rev. 204 (August 2019), 103146. doi:10.1016/j.earscirev.2020.103146
Lass, H. U., and Mohrholz, V. (2005). On the Fluctuations and Vertical Structure of the Shelf Circulation off Walvis Bay, Namibia. Continental Shelf Res. 25 (12–13), 1473–1497. doi:10.1016/j.csr.2005.04.012
Lavik, G., Stührmann, T., Brüchert, V., Van der Plas, A., Mohrholz, V., Lam, P., et al. (2009). Detoxification of Sulphidic African Shelf Waters by Blooming Chemolithotrophs. Nature 457 (7229), 581–584. doi:10.1038/nature07588
Lazarus, D., Bittniok, B., Diester-Haass, L., Meyers, P., and Billups, K. (2006). Comparison of Radiolarian and Sedimentologic Paleoproductivity Proxies in the Latest Miocene-Recent Benguela Upwelling System. Mar. Micropaleontology 60 (4), 269–294. doi:10.1016/j.marmicro.2006.06.003
le Roux, J. P. (1991). A Rapid Method to Determine the Critical Shear Stress for Sphere Entrainment under Unidirectional Fluid Flow. Sediment. Geol. 75 (1–2), 1–3. doi:10.1016/0037-0738(91)90045-F
Levin, L. A. (2003). Oxygen Minimum Zone Benthos: Adaptation and Community Response to Hypoxia. Oceanogr. Mar. Biol. Annu. Rev. 41, 1–45. [online] Available from: http://scrippsscholars.ucsd.edu/llevin/content/oxygen-minimum-zone-benthos-adaptation-and-community-response-hypoxia.
Levin, L. A., and Sibuet, M. (2012). Understanding Continental Margin Biodiversity: A New Imperative. Annu. Rev. Mar. Sci. 4 (1), 79–112. doi:10.1146/annurev-marine-120709-142714
Lubarsky, H. V., Hubas, C., Chocholek, M., Larson, F., Manz, W., Paterson, D. M., et al. (2010). The Stabilisation Potential of Individual and Mixed Assemblages of Natural Bacteria and Microalgae. PLoS One, 5, e13794. doi:10.1371/journal.pone.0013794
Lyons, T. W., Reinhard, C. T., and Planavsky, N. J. (2014). The Rise of Oxygen in Earth's Early Ocean and Atmosphere. Nature 506 (7488), 307–315. doi:10.1038/nature13068
Mantz, P. A. (1977). Incipient Transport of Fine Grains and Flakes by Fluids - Extended Shields Diagram. J. Hydr. Div. 103 (6), 601–615. (HY6) [online] Available from: https://trid.trb.org/Results?txtKeywords=&txtTitle=INCIPIENT TRANSPORT OF FINE GRAINS AND FLAKES BY FLUIDS-EXTENDED SHIELD DIAGRAM&txtSerial=%22Journal of the Hydraulics Division%22&ddlSubject=1800&txtReportNum=&ddlTrisfile=&tx (Accessed May 16, 2019). doi:10.1061/jyceaj.0004766
Mayer, L. M., Schick, L. L., Hardy, K. R., Wagai, R., and McCarthy, J. (2004). Organic Matter in Small Mesopores in Sediments and Soils. Geochimica et Cosmochimica Acta 68 (19), 3863–3872. doi:10.1016/j.gca.2004.03.019
Mayer, L. M. (1994). Surface Area Control of Organic Carbon Accumulation in continental Shelf Sediments. Geochimica et Cosmochimica Acta 58 (4), 1271–1284. doi:10.1016/0016-7037(94)90381-6
McCave, I. N., Manighetti, B., and Robinson, S. G. (1995). Sortable silt and fine Sediment Size/composition Slicing: Parameters for Palaeocurrent Speed and Palaeoceanography. Paleoceanography 10 (3), 593–610. doi:10.1029/94PA03039
McCave, I. N. (2009). “Nepheloid Layers,” in Encyclopedia of Ocean Sciences (Oxford, United Kingdom: Elsevier), 8–18. doi:10.1016/b978-012374473-9.00671-8
McCave, I. N. (1984). Particulate Size Spectra, Behavior, and Origin of Nepheloid Layers over the Nova Scotian Continental Rise. J. Geophys. Res. 88 (C12), 7647–7666. doi:10.1029/JC088iC12p07647
McCave, I. N., Thornalley, D. J. R., and Hall, I. R. (2017). Relation of Sortable silt Grain-Size to Deep-Sea Current Speeds: Calibration of the 'Mud Current Meter'. Deep Sea Res. Part Oceanographic Res. Pap. 127 (July), 1–12. doi:10.1016/j.dsr.2017.07.003
Mehta, A. J. (1988). “Laboratory Studies on Cohesive Sediment Deposition and Erosion,” in Physical Processes in Estuaries. Editors J. Dronkers, and W. van Leussen (Berlin, Heidelberg, Berlin, Heidelberg: Springer), 427–445. doi:10.1007/978-3-642-73691-9_21
Middelburg, J. J. (1989). A Simple Rate Model for Organic Matter Decomposition in marine Sediments. Geochimica et Cosmochimica Acta 53 (7), 1577–1581. doi:10.1016/0016-7037(89)90239-1
Miederma, S. A. (2010). “Constructing the Shields Curve, a New Theoretical Approach and its Applications. See Also Other Papers on the Shields Curve,” in World Dredging Conference XIX (Beijing China: WODCON XIX). [online] Available from: https://www.researchgate.net/publication/228750218_Constructing_the_Shields_curve_a_new_theoretical_approach_and_its_applications_See_also_other_papers_on_the_Shields_curve/references.
Miller, M. C., McCave, I. N., and Komar, P. D. (1977). Threshold of Sediment Motion under Unidirectional Currents. Sedimentology 24 (4), 507–527. doi:10.1111/j.1365-3091.1977.tb00136.x
Mills, B. J. W., Krause, A. J., Scotese, C. R., Hill, D. J., Shields, G. A., and Lenton, T. M. (2019). Modelling the Long-Term Carbon Cycle, Atmospheric CO2, and Earth Surface Temperature from Late Neoproterozoic to Present Day. Gondwana Res. 67, 172–186. doi:10.1016/j.gr.2018.12.001
Moffitt, S. E., Moffitt, R. A., Sauthoff, W., Davis, C. V., Hewett, K., and Hill, T. M. (2015). Paleoceanographic Insights on Recent Oxygen Minimum Zone Expansion: Lessons for Modern Oceanography. PLoS One 10 (1), e0115246–39. doi:10.1371/journal.pone.0115246
Mohrholz, V., Bartholomae, C. H., van der Plas, A. K., and Lass, H. U. (2008). The Seasonal Variability of the Northern Benguela Undercurrent and its Relation to the Oxygen Budget on the Shelf. Continental Shelf Res. 28 (3), 424–441. doi:10.1016/j.csr.2007.10.001
Mohrholz, V., Eggert, A., Junker, T., Nausch, G., Ohde, T., and Schmidt, M. (2014). Cross Shelf Hydrographic and Hydrochemical Conditions and Their Short Term Variability at the Northern Benguela during a normal Upwelling Season. J. Mar. Syst. 140, 92–110. doi:10.1016/j.jmarsys.2014.04.019
Mollenhauer, G., Eglinton, T. I., Hopmans, E. C., and Sinninghe Damsté, J. S. A. (2008). A Radiocarbon-Based Assessment of the Preservation Characteristics of Crenarchaeol and Alkenones From Continental Margin Sediments. Org. Geochem. 39, 1039–1045. doi:10.1016/j.orggeochem.2008.02.006
Mollenhauer, G., Eglinton, T. I., Ohkouchi, N., Schneider, R. R., Müller, P. J., Grootes, P. M., et al. (2003). Asynchronous Alkenone and Foraminifera Records from the Benguela Upwelling System. Geochimica et Cosmochimica Acta 67 (12), 2157–2171. doi:10.1016/S0016-7037(03)00168-6
Mollenhauer, G., Inthorn, M., Vogt, T., Zabel, M., Sinninghe Damsté, J. S., and Eglinton, T. I. (2007). Aging of marine Organic Matter during Cross-Shelf Lateral Transport in the Benguela Upwelling System Revealed by Compound-specific Radiocarbon Dating. Geochem. Geophys. Geosyst. 8 (9), a–n. doi:10.1029/2007GC001603
Mollenhauer, G., Kienast, M., Lamy, F., Meggers, H., Schneider, R. R., Hayes, J. M., et al. (2005). An Evaluation of14C Age Relationships between Co-occurring Foraminifera, Alkenones, and Total Organic Carbon in continental Margin Sediments. Paleoceanography 20 (1), a–n. doi:10.1029/2004PA001103
Mollenhauer, G., Schneider, R. R., Jennerjahn, T., Müller, P. J., and Wefer, G. (2004). Organic Carbon Accumulation in the South Atlantic Ocean: Its Modern, Mid-holocene and Last Glacial Distribution. Glob. Planet. Change 40 (3–4), 249–266. doi:10.1016/j.gloplacha.2003.08.002
Mollenhauer, G., Schneider, R. R., Müller, P. J., Spieß, V., and Wefer, G. (2002). Glacial/interglacial Variablity in the Benguela Upwelling System: Spatial Distribution and Budgets of Organic Carbon Accumulation. Glob. Biogeochem. Cycles 16 (4), 81–1. doi:10.1029/2001GB001488
Monteiro, P. M. S., Nelson, G., Van Der Plas, A., Mabille, E., Bailey, G. W., and Klingelhoeffer, E. (2005). Internal Tide-Shelf Topography Interactions as a Forcing Factor Governing the Large-Scale Distribution and Burial Fluxes of Particulate Organic Matter (POM) in the Benguela Upwelling System. Continental Shelf Res. 25 (15), 1864–1876. doi:10.1016/j.csr.2005.06.012
Monteiro, P. M. S., Van Der Plas, A., Mohrholz, V., Mabille, E., Pascall, A., and Joubert, W. (2006). Variability of Natural Hypoxia and Methane in a Coastal Upwelling System: Oceanic Physics or Shelf Biology? Geophys. Res. Lett. 33 (16), L16614. doi:10.1029/2006GL026234
Moore, D. G., and Keller, G. H. (1984). “Marine Sediments, Geotechnical Properties,” in Applied Geology. Encyclopedia of Earth Sciences Series. Editor C. W. Finkl (Boston, MA: Springer US), 343–350.
Moriarty, J. M., Harris, C. K., Friedrichs, M. A. M., Fennel, K., and Xu, K. (2018). Impact of Seabed Resuspension on Oxygen and Nitrogen Dynamics in the Northern Gulf of Mexico: A Numerical Modeling Study. J. Geophys. Res. Oceans 123 (10), 7237–7263. doi:10.1029/2018JC013950
Nagel, B., Gaye, B., Lahajnar, N., Struck, U., and Emeis, K.-C. (2016). Effects of Current Regimes and Oxygenation on Particulate Matter Preservation on the Namibian Shelf: Insights from Amino Acid Biogeochemistry. Mar. Chem. 186, 121–132. doi:10.1016/j.marchem.2016.09.001
NASA (2018). NASA Ocean Color Data, Goddard Sp. Flight Center, Ocean Ecol. Lab. Ocean Biol. Process. Group. Moderate-Resolution Imaging Spectroradiom. Aqua (CHL). NASA [online] Available from: https://oceancolor.gsfc.nasa.gov/(Accessed January 7, 2022).
Ncei - National Centers for Environmental Information, (2016). Global Ocean Currents Database - Database, NCEI Glob. Ocean Curr. Database. [online] Available from: https://www.ncei.noaa.gov/access/data/global-ocean-currents-database/category.html (Accessed January 7, 2022).
Nelson, G., and Hutchings, L. (1983). The Benguela Upwelling Area. Prog. Oceanography 12 (3), 333–356. doi:10.1016/0079-6611(83)90013-7
Neumann, A., Lahajnar, N., and Emeis, K.-C. (2016). Benthic Remineralisation Rates in Shelf and Slope Sediments of the Northern Benguela Upwelling Margin. Continental Shelf Res. 113, 47–61. doi:10.1016/j.csr.2015.12.009
Ohkouchi, N., Eglinton, T. I., Keigwin, L. D., and Hayes, J. M. (2002). Spatial and Temporal Offsets between Proxy Records in a Sediment Drift. Science (80-. ) 298 (5596), 1224–1227. doi:10.1126/science.1075287
Ohkouchi, N. (2002). Spatial and Temporal Offsets between Proxy Records in a Sediment Drift. Science 298 (5596), 1224–1227. doi:10.1126/science.1075287
Oschlies, A., Brandt, P., Stramma, L., and Schmidtko, S. (2018). Drivers and Mechanisms of Ocean Deoxygenation. Nat. Geosci. 11 (7), 467–473. doi:10.1038/s41561-018-0152-2
Passow, U., and De La Rocha, C. L. (2006). Accumulation of mineral Ballast on Organic Aggregates. Glob. Biogeochem. Cycles 20 (1), n/a. doi:10.1029/2005GB002579
Premuzic, E. T., Benkovitz, C. M., Gaffney, J. S., and Walsh, J. J. (1982). The Nature and Distribution of Organic Matter in the Surface Sediments of World Oceans and Seas. Org. Geochem. 4 (2), 63–77. doi:10.1016/0146-6380(82)90009-2
Ransom, B., Kim, D., Kastner, M., and Wainwright, S. (1998). Organic Matter Preservation on continental Slopes: Importance of Mineralogy and Surface Area. Geochim. Cosmochim. Acta 62 (8), 1329–1345. doi:10.1016/S0016-7037(98)00050-7
Reimer, P. J., Brown, T. A., and Reimer, R. W. (2004). Discussion: Reporting and Calibration of post-bomb 14C Data. Radiocarbon 46 (1), 1111–1150. doi:10.2458/azu_js_rc.46.4183
Richardson, M. J., and Gardner, W. D. (1985). Analysis of Suspended-Particle-Size Distributions over the Nova Scotian Continental Rise. Mar. Geol. 66 (1–4), 189–203. doi:10.1016/0025-3227(85)90029-5
Righetti, M., and Lucarelli, C. (2007). May the Shields Theory Be Extended to Cohesive and Adhesive Benthic Sediments? J. Geophys. Res. Ocean. 112 (5), 1–14. doi:10.1029/2006JC003669
Righetti, M., and Lucarelli, C. (2010). Resuspension Phenomena of Benthic Sediments: the Role of Cohesion and Biological Adhesion. River Res. Appl. 26, 404–413. doi:10.1002/rra10.1002/rra.1296
Robinson, R. S., Meyers, P. A., and Murray, R. W. (2002). Geochemical Evidence for Variations in Delivery and Deposition of Sediment in Pleistocene Light-Dark Color Cycles under the Benguela Current Upwelling System. Mar. Geol. 180 (1–4), 249–270. doi:10.1016/S0025-3227(01)00217-1
Saidy, A. R., Smernik, R. J., Baldock, J. A., Kaiser, K., and Sanderman, J. (2013). The Sorption of Organic Carbon onto Differing clay Minerals in the Presence and Absence of Hydrous Iron Oxide. Geoderma 209–210, 15–21. doi:10.1016/j.geoderma.2013.05.026
Salman, V., Bailey, J. V., and Teske, A. (2013). Phylogenetic and Morphologic Complexity of Giant sulphur Bacteria, Antonie Van Leeuwenhoek. Int. J. Gen. Mol. Microbiol. 104 (2), 169–186. doi:10.1007/s10482-013-9952-y
Sanders, H. L. (1968). Mar. Benthic Divers. A Comp. Study Am. Nat. 102 (925), 243–282. doi:10.1086/282541
Schlanger, S. O., and Jenkyns, H. C. (1976). Cretaceous Oceanic Anoxic Events: Causes and Consequences. Geol. en Mijnb. 55 (3–4), 179–184. [online] Available from: https://ora.ox.ac.uk/objects/uuid:0921605b-4793-43df-889d-7b896790de62.
Schreiner, K. M., Bianchi, T. S., Eglinton, T. I., Allison, M. A., and Hanna, A. J. M. (2013). Sources of Terrigenous Inputs to Surface Sediments of the Colville River Delta and Simpson’s Lagoon, Beaufort Sea, Alaska. J. Geophys. Res. Biogeosciences 118 (2), 808–824. doi:10.1002/jgrg.20065
Schulz, H. N., Brinkhoff, T., Ferdelman, T. G., Hernández Mariné, M., Teske, A., and Jørgensen, B. B. (1999). Dense Populations of a Giant Sulfur Bacterium in Namibian Shelf Sediments. Science (80-. ) 284 (5413), 493–495. doi:10.1126/science.284.5413.493
Shannon, L. V., Nelson, G., and Jury, M. R. (1996). “Hydrological and Meteorological Aspects of Upwelling in the Southern Benguela Current,” in Coastal Upwelling (: American Geophysical Union), 146–159.
Shields, A. (1936). Anwendung der Ähnlichkeitsmechanik und der Turbulenzforschung auf die Geschiebebewegung. Berlin.: Technische Hochschule.
Simon, M., Grossart, H., Schweitzer, B., and Ploug, H. (2002). Microbial Ecology of Organic Aggregates in Aquatic Ecosystems. Aquat. Microb. Ecol. 28 (2), 175–211. doi:10.3354/ame028175
Skogen, M. D. (1999). A Biophysical Model Applied to the Benguela Upwelling System. South Afr. J. Mar. Sci. 7615 (21), 235–249. doi:10.2989/025776199784126042
Sloth, N. P., Riemann, B., Nielsen, L. P., and Blackburn, T. H. (1996). Resilience of Pelagic and Benthic Microbial Communities to Sediment Resuspension in a Coastal Ecosystem, Knebel Vig, Denmark. Estuar. Coast. Shelf Sci. 42 (4), 405–415. doi:10.1006/ecss.1996.0027
Sollins, P., Swanston, C., Kleber, M., Filley, T., Kramer, M., Crow, S., et al. (2006). Organic C and N Stabilization in a forest Soil: Evidence from Sequential Density Fractionation. Soil Biol. Biochem. 38 (11), 3313–3324. doi:10.1016/j.soilbio.2006.04.014
Southard, J. (2006). Introduction to Fluid Motions, Sediment Transport, and Current-Generated Sedimentary Structures. Cambridge, MA: MIT OpenCourseSoftware, 260–284. Available from: https://ocw.mit.edu/courses/earth-atmospheric-and-planetary-sciences/12-090-introduction-to-fluid-motions-sediment-transport-and-current-generated-sedimentary-structures-fall-2006/course-textbook/ch9.pdf.
Summerhayes, C. P., Kroon, D., Rosell-Melé, A., Jordan, R. W., Schrader, H.-J., Hearn, R., et al. (1995). Variability in the Benguela Current Upwelling System over the Past 70,000 Years. Prog. Oceanogr. 35 (3), 207–251. doi:10.1016/0079-6611(95)00008-5
Synal, H. A., Stocker, M., and Suter, M. (2007). MICADAS: A New Compact Radiocarbon AMS System. Nucl. Instr. Methods Phys. Res. Sect. B Beam Interact. Mater. Atoms 259 (1), 7–13. doi:10.1016/j.nimb.2007.01.138
Teisson, C., Ockenden, M., Le Hir, P., Kranenburg, C., and Hamm, L. (1993). Cohesive Sediment Transport Processes. Coast. Eng. 21 (1–3), 129–162. doi:10.1016/0378-3839(93)90048-D
Thiele, S., Basse, A., Becker, J. W., Lipski, A., Iversen, M. H., and Mollenhauer, G. (2018). Microbial Communities in the Nepheloid Layers and Hypoxic Zones of the Canary Current Upwelling System. Microbiologyopen 8, e705. doi:10.1002/mbo3.705
Thomsen, L., and Gust, G. (2000). Sediment Erosion Thresholds and Characteristics of Resuspended Aggregates on the Western European continental Margin. Deep. Res. Part. Oceanogr. Res. Pap. 47 (10), 1881–1897. doi:10.1016/S0967-0637(00)00003-0
Tolhurst, T. J., Consalvey, M., and Paterson, D. M. (2008). Changes in Cohesive Sediment Properties Associated with the Growth of a Diatom Biofilm. Hydrobiologia 596 (1), 225–239. doi:10.1007/s10750-007-9099-9
Tolhurst, T. J., Gust, G., and Paterson, D. M. (2002). The Influence of an Extracellular Polymeric Substance (EPS) on Cohesive Sediment Stability. Proc. Mar. Sci. 5, 409–425. doi:10.1016/s1568-2692(02)80030-4
Tyson, R. V., and Pearson, T. H. (1991). Modern and Ancient continental Shelf Anoxia: An Overview. Geol. Soc. Spec. Publ. 58 (1), 1–24. doi:10.1144/GSL.SP.1991.058.01.01
Unsöld, G. (1984). Der Transportbeginn feinstkörnigen rolligen Sohlmaterials in gleichförmigen turbulenten Strömungen: eine experimentelle Überprüfung und Erweiterung der SHIELDS-Funktion, 125. Reports Sonderforschungsbereich 95 Wechselwirkung Meer - Meeresboden, (70).
Van Der Plas, A. K., Monteiro, P. M. S., and Pascall, A. (2007). Cross-shelf Biogeochemical Characteristics of Sediments in the central Benguela and Their Relationship to Overlying Water Column Hypoxia. Afr. J. Mar. Sci. 29 (1), 37–47. doi:10.2989/AJMS.2007.29.1.3.68
Van Der Voort, T. S., Blattmann, T. M., Usman, M., Montluçon, D., Loeffler, T., Tavagna, M. L., et al. (2021). MOSAIC (Modern Ocean Sediment Archive and Inventory of Carbon): A (Radio)carbon-centric Database for Seafloor Surficial Sediments. Earth Syst. Sci. Data 13 (5), 2135–2146. doi:10.5194/essd-13-2135-2021
Wakeham, S. G., Canuel, E. A., Lerberg, E. J., Mason, P., Sampere, T. P., and Bianchi, T. S. (2009). Partitioning of Organic Matter in continental Margin Sediments Among Density Fractions. Mar. Chem. 115 (3–4), 211–225. doi:10.1016/j.marchem.2009.08.005
Wakeham, S. G., and Canuel, E. A. (2016). The Nature of Organic Carbon in Density-Fractionated Sediments in the Sacramento-San Joaquin River Delta (California). Biogeosciences 13 (2), 567–582. doi:10.5194/bg-13-567-2016
Ward, B. D. (1969). Relative Density Effects on Incipient Bed Movement. Water Resour. Res. 5 (5), 1090–1096. doi:10.1029/WR005i005p01090
Welte, C., Hendriks, L., Wacker, L., Haghipour, N., Eglinton, T. I., Günther, D., et al. (2018). Towards the Limits: Analysis of Microscale 14C Samples Using EA-AMS. Nucl. Instr. Methods Phys. Res. Sect. B Beam Interact. Mater. Atoms 437, 66–74. doi:10.1016/j.nimb.2018.09.046
Yalin, M. S., and Karahan, E. (1979). Inception of Sediment Transport. J. Hydraul. Div. 105 (11), 1433–1443. doi:10.1061/jyceaj.0005306
Yang, W. Y., Yu, G. L., Tan, S. K., and Wang, H. K. (2014). Rheological Properties of Dense Natural Cohesive Sediments Subject to Shear Loadings. Int. J. Sediment. Res. 29 (4), 454–470. doi:10.1016/S1001-6279(14)60059-7
Ziervogel, K., Dike, C., Asper, V., Montoya, J., Battles, J., D’souza, N., et al. (2016). Enhanced Particle Fluxes and Heterotrophic Bacterial Activities in Gulf of Mexico Bottom Waters Following Storm-Induced Sediment Resuspension. Deep. Res. Part. Top. Stud. Oceanogr. 129, 77–88. doi:10.1016/j.dsr2.2015.06.017
Keywords: sedimentary processes, anoxia, resuspension, SLOB hypothesis, marine carbon cycle, sediment threshold of motion, organic matter
Citation: Bruni ET, Blattmann TM, Haghipour N, Louw D, Lever M and Eglinton TI (2022) Sedimentary Hydrodynamic Processes Under Low-Oxygen Conditions: Implications for Past, Present, and Future Oceans. Front. Earth Sci. 10:886395. doi: 10.3389/feart.2022.886395
Received: 28 February 2022; Accepted: 08 April 2022;
Published: 09 May 2022.
Edited by:
Xiting Liu, Ocean University of China, ChinaReviewed by:
Ding He, Hong Kong University of Science and Technology, Hong Kong SAR, ChinaCopyright © 2022 Bruni, Blattmann, Haghipour, Louw, Lever and Eglinton. This is an open-access article distributed under the terms of the Creative Commons Attribution License (CC BY). The use, distribution or reproduction in other forums is permitted, provided the original author(s) and the copyright owner(s) are credited and that the original publication in this journal is cited, in accordance with accepted academic practice. No use, distribution or reproduction is permitted which does not comply with these terms.
*Correspondence: Elena T. Bruni, ZWxlbmEuYnJ1bmlAZXJkdy5ldGh6LmNo
Disclaimer: All claims expressed in this article are solely those of the authors and do not necessarily represent those of their affiliated organizations, or those of the publisher, the editors and the reviewers. Any product that may be evaluated in this article or claim that may be made by its manufacturer is not guaranteed or endorsed by the publisher.
Research integrity at Frontiers
Learn more about the work of our research integrity team to safeguard the quality of each article we publish.