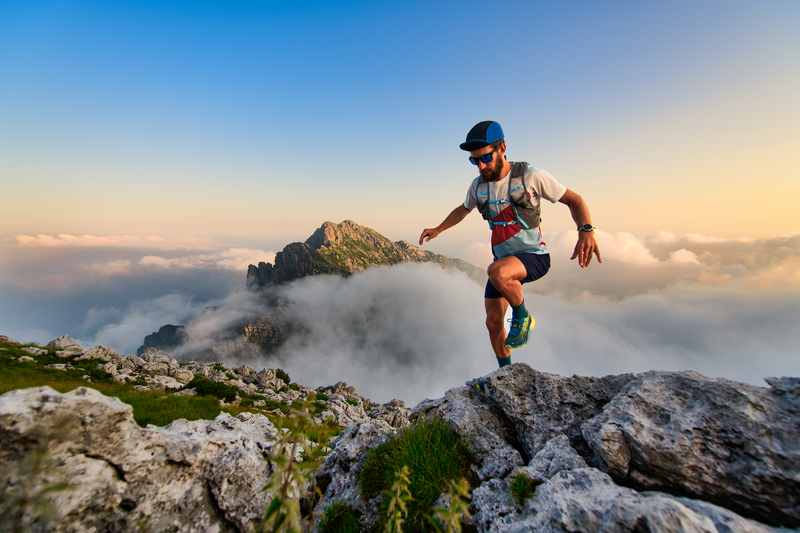
95% of researchers rate our articles as excellent or good
Learn more about the work of our research integrity team to safeguard the quality of each article we publish.
Find out more
ORIGINAL RESEARCH article
Front. Earth Sci. , 18 May 2022
Sec. Biogeoscience
Volume 10 - 2022 | https://doi.org/10.3389/feart.2022.886200
This article is part of the Research Topic Sedimentation on the Continental Margins: From Modern Processes to Deep-Time Records View all 28 articles
Archaeal ammonia oxidation is the most important intermediate pathway in regulating the oceanic nitrogen cycle; however, the study of its specific role on a geological time scale is restricted to a specific part of marginal seas; thus far, only in the southern South China Sea (SCS). To explore the spatial pattern of the role of archaeal ammonia oxidation in the SCS, the GDGT-[2]/[3] ratio (Glycerol Dialkyl Glycerol Tetraether), an indicator of the archaeal ammonia oxidation rate, was analyzed and examined from the collected data profiles since the last glacial period in the northern SCS. The results showed that the GDGT-[2]/[3] ratio in the northern SCS was opposite to that in the southern SCS, with higher GDGT-[2]/[3] values during the Holocene compared to the last glacial period. Based on existing published depths of thermocline (DOT) data in the northern SCS since 30 ka, we believe that hydrological structural variations induced by mesoscale eddies caused this difference. Therefore, physical processes are very important factors that control the nitrogen cycle over a long-time scale. This study may provide new insights into the understanding of the role of archaeal ammonia oxidation within the marine nitrogen cycle over geological time scale.
Nitrogen is a key limiting nutrient of primary productivity, and changes in the total amount of fixed nitrogen in the ocean will lead to changes in primary productivity and thus affect atmospheric CO2 levels, which in turn will have a significant impact on global climate changes (Beatty-Desana et al., 1975; McElroy, 1983; Altabet et al., 2002). The understanding of the interrelationships between the main processes of the marine nitrogen cycle and their regulators is still very limited (Zehr & Capone, 2020).
N isotopes in marine sediment record key information of oceanic biogeochemical information, and indicate the corresponding processes of the marine nitrogen cycle, and also can track the evolution of the marine environment and biogeochemical cycle in the marine system during geological periods (Altabet, 2006). Specifically, the sedimentary nitrogen isotope ratio (δ15N) reflects a signal of processes of nitrogen input (i.e., nitrogen fixation) and nitrogen loss to the atmosphere (i.e., anaerobic denitrification and anaerobic ammonium oxidation (anammox) (Sigman & Fripiat, 2019). In general, the process of nitrogen fixation from the atmosphere can result in a lower δ15N value of nitrate in the seawater with an isotope discrimination of ∼-1‰ (Wada & Hattori, 1976; Liu et al., 1996), while water column denitrification will enrich the 15N of nitrate with isotope fractionation up to 20–30‰ (Cline & Kaplan, 1975; Brandes et al., 1981).
As one of the largest marginal seas in the world, the SCS is surrounded by densely populated islands, which brings a large input of terrigenous nutrients, resulting in high deposition rates and sensitivity to environmental changes, and is thus an ideal area for studying biogeochemical cycles under both natural and anthropogenic influences (Wang and Li, 2009). Previous studies have investigated the sedimentary nitrogen isotope characteristics in the northern and southern SCS during geological periods. Dong et al. (2019) reconstructed the variation in nitrogen isotopes in the southern SCS based on the nitrogen isotopes of organic matter (δ15Norg). Their results showed that δ15Norg values increased during the last glaciation (MIS 2) which is in accord with the trend of the δ15Nforam reconstructed from planktonic foraminifera observed in core MD97-2142 (Ren et al., 2017) and in MD12-3433 (Wang et al., 2018) retrieved from the northern SCS. Nitrogen isotope ratios based on planktonic foraminifera G. scacculifer and G. ruber showed the same trend, indicating that nitrogen fixation has been enhanced since the last glacial period throughout the whole SCS. In short, nitrogen isotope indices are the key for better understanding nitrogen fixation in the SCS and reconstructing nitrogen fixation in the paleo-nitrogen cycle.
However, δ15N incorporates a mixing signal but cannot fully reflect other details within the complex nitrogen cycle (Robinson, 2001; Dong et al., 2019). Therefore, other proxies, such as organic biomarkers, become a potential tool for tracking more refined marine nitrogen cycle processes (Rush and Sinninghe Damsté, 2017), such as nitrification, which act as a tie line between the nitrogen input and nitrogen loss (Canfield et al., 2010; Rush and Sinninghe Damsté, 2017). Previously, the archaeal ammonia oxidation in the southern SCS since 180 ka was reconstructed by Dong et al. (2019) based on the proxy of the relative abundance between shallow-water clade and deep-water clade Thaumarchaeota, the ratio of glycerol dialkyl glycerol tetraethers (GDGT) with 2 and 3 cyclopentyl moieties (GDGT-2 to GDGT-3 ratio, here after referred to as [2]/[3]) (Dong et al., 2019). Ammonia oxidizing archaea (AOA), affiliated with Thaumarchaeota, are distributed throughout the water column but have maximum abundance at the bottom of the deep chlorophyll maximum (DCM). A higher [2]/[3] indicates a higher contribution of deep-water clade Thaumarchaeota with low ammonia-oxidizing activity, while a lower [2]/[3] indicates a higher contribution of shallow-water clade AOA with high ammonia-oxidizing activity (Jia et al., 2017; Dong et al., 2019). However, whether this conclusion pattern is consistent in other seas remains to be verified.
Here, by collecting and analyzing the [2]/[3] data from sediment cores retrieved from the SCS combined with δ15N data, we found that unlike the scenario observed in the southern SCS, the ammonia oxidation and nitrogen fixation have decoupled in the northern SCS since 30 ka. Different hydrodynamics between the northern and southern SCS have been examined to explore the mechanisms of the different relationships between ammonia oxidation and nitrogen fixation, thus improving the understanding of the mechanisms underlying the nitrogen cycle in the SCS since the last glacial period (MIS 1–2).
The SCS is located between the tropical western Pacific Ocean and the Asian continent, and it is under the control of the East Asian monsoon (EAM) system. In the boreal winter, the interaction between the low-pressure system over the western Pacific warm pool and the Siberian high-pressure system triggers the formation of a strong East Asian winter monsoon (EAWM) (Figure 1). The cyclonic circulation is driven by the EAWM in the entire basin. While in boreal summer, the prevailing southwesterly monsoon (i.e., East Asian summer monsoon, EASM) drives anticyclonic circulation formation in the whole basin (Xiu et al., 2010) (Figure 1). Therefore, different surface circulation patterns in the winter and summer seasons are driven by the EAM system in the SCS. Modern physical oceanographic observations have shown that anticyclonic eddies dominate the northern part of the SCS in summer (Xiu et al., 2010; Guo et al., 2015), which drives the thermocline and halocline to deepen and become thicker (Chen et al., 2011).
FIGURE 1. Location of MD01-2392, MD05-2897, and MD97-2142 sites in the southern SCS and MD12-3428, ODP1147, MD12-3433, and MD01-2904 in the northern SCS.
The effect of the EAM system on the water hydrodynamics in the SCS in the geological periods has been investigated. Dong et al. (2015) reconstructed the thermocline depth of the southern SCS since 180 ka using the temperature difference (ΔT) between the reconstructed surface seawater temperature by utilizing
Mesoscale eddies influence the marine carbon and nitrogen cycle by regulating the distribution of nutrients, in turn affecting the biota (Zehr & Kudela, 2011). As a key player in marine nitrogen cycle, the abundance and activity of AOA are affected by temperature, light, availability of ammonia, and its competition (McElroy, 1983) with phytoplankton, especially in shallow coastal waters (Herfort et al., 2007; Q. Liu et al., 2018; Urakawa et al., 2014). Some of these factors can be altered by mesoscale processes, e.g., upwelling (Molina et al., 2010), water mass mixing (Müller et al., 2018) (Haas et al., 2021), and in turn, modify the AOA community. In geological time, the physical and hydrological properties of the surface waters in the SCS have been proposed to influence the growth of shallow AOA (Dong et al., 2019), thus impacting the marine nitrogen cycle.
Membrane lipid biomarkers [2]/[3] of two cores which from the northern SCS were analyzed: one core MD12-3428: (20°08.48′N, 115°49.80′E; core length 10.15 m, water depth 903 m) (Dong et al., 2018), and core ODP 1147 (Figure 1) (18°50.11′N, 116°33.28′E; water depth: 3246 m) (Li et al., 2013). The age model of core MD12-3428 was established based on both accelerator mass spectrometry (AMS) radiocarbon dates of planktonic foraminifera (Globigerinoides ruber) and the oxygen isotopes aligning with existing oxygen isotopes, covering the past 30 ka (H. Zhang H et al., 2016; Y. G. Zhang Y G et al., 2016). The total lipids of MD12-3428 were extracted following Sturt et al. (2004) and GDGT-2, GDGT-3 and other lipids used to calculate the
Additionally, the age model of core ODP 1147 was established by using benthic foraminifera oxygen isotope data. The GDGTs were detected and analyzed by using an Agilent 1200 HPLC coupled to a Waters Micromass-Quattro Ultima™ Pt mass spectrometer with an APCI probe (Li et al., 2013).
where
according to Schouten et al. (2002).
The sea surface temperature indicator
according to (Prahl & Wakeham, 1987). We calculate the sea surface temperature (SST) by following the calibration:
SST =
The ΔT is defined as:
ΔT = SST-SSST
which has been used to indicate the stratification of the ocean in the south China Sea (Dong et al., 2015) (Li et al., 2013). The δ15N isotopic data for the northern SCS since 30 ka were from core MD12-3433 (19°16.88′N, 116°14.52′E; water depth 2125 m) (Wang et al., 2018), and productivity data were from core MD05-2904 (19°27.32′N, 116°15.15′E, water depth 2066 m) (He et al., 2008).
The biomarker [2]/[3] ratio of the southern SCS was also obtained from core MD05-2897 (Figure 1) (08°49.05′N, 111°26.469′E; water depth 1,657 m; column length 11.03 m) (Dong et al., 2015). Ultrasonic extraction was used for total lipid extraction. Detail extraction and analytical methods were described in (Hopmans et al., 2004; Huguet, 2007; Dong et al., 2015). The δ15N isotopic data were from the core MD97-2142 (12°41′N, 119°27′E; water depth: 1,557 m) (Ren et al., 2017) based on the planktonic foraminifera G. ruber and the core MD01-2392 (09°51.13′N, 110°12.64′E; water depth: 1966 m) (Dong et al., 2019) based on sedimentary particulate organic nitrogen.
The [2]/[3] ratio was investigated in both the northern and southern SCS and showed a distinct variation pattern since 30 ka. In the southern SCS (Figure 2A), [2]/[3] ranged from 7.0–9.4 with a mean value of 7.9 and progressively increased in MIS 2. In MIS 1, [2]/[3] ranged from 5.9–10.6 with an average of 7.3 and showed a decreasing trend. However, it had a different pattern in the northern SCS (Figure 2B). In core MD12-3428, the [2]/[3] ranged from 5.3–6.3 with an average of 5.8 in MIS 2, while in MIS 1, [2]/[3] ranged from 6.1–7.2 with a mean value of 6.6. Similarly, in core ODP 1147 retrieved from the northern SCS, [2]/[3] ranged from 4.69 to 6.42 with a mean value of 5.75 in MIS 2, and ranged from 5.67 to 6.42 with 6.16 on average in MIS 1. Both cores in the northern SCS showed increasing trends of [2]/[3] since 30 ka, indicating that they have unique and identical regional implications under which the controlling mechanisms were different from those of the southern SCS.
FIGURE 2. Comparison of the relationship between nitrogen fixation and archaeal ammoxidation in the northern and southern SCS since 30 ka. (A) δ15Norg values of southern cores MD01-2392 (Dong et al., 2019) and MD97-2142 (Ren et al., 2017). (B) Nitrogen isotope FB-δ15N of planktic foraminifers in northern core MD12-3433 (Wang et al., 2018). (C) GDGT-[2]/[3] ratio of southern core MD05-2897 (Dong et al., 2015); (D) GDGT-[2]/[3] ratio of northern cores MD12-3428 (Dong et al., 2018) and ODP 1147 (Li et al., 2013).
The mechanisms that control the [2]/[3] and its implications had been interpreted in the southern SCS by Dong et al. (2019). The biomarker [2]/[3] reflects the abundance of Shallow-water clade Thaumarchaeota which determines the ammonia oxidation. Specifically, shallow-water clade Thaumarchaeota, with lower [2]/[3], have better ammonia concentration adaptation due to their phylogenetic distinction compared to their deep-water clade relatives (Dong et al., 2019) and take part in relatively active ammonia oxidation (Xu et al., 2018). Therefore, a lower [2]/[3] indicates higher shallow-water clade AOA abundance and a higher ammonia oxidation rate (Dong et al., 2019). In core MD05-2897, a similar variation trend between [2]/[3] and δ15N since 30 ka indicates that the coupling of ammonia oxidation and nitrogen fixation in the southern SCS (Figures 2A,C). δ15N decreased from MIS 2 to MIS 1. This indicates an enhancement of nitrogen fixation which, to some extent, is probably contributed by the consumption of oxygen due to the enhancement of ammonia oxidation indicated by the decreasing trend of [2]/[3] since the last glacial period (Figure 2C).
Considering the depth segregation of ammonia oxidation and nitrogen fixation, i.e., ammonia oxidation in the nutricline and nitrogen fixation mainly in the nutrient-depleted layer above the nutricline (Michael Beman et al., 2012; Du et al., 2017), Dong et al. (2019) concluded that the two pathways were not directly but indirectly coupled. Global marine nitrogen data-based investigation showed that sea surface temperature and subsurface minimum O2 are two main factors that influence nitrogen fixation variations on a global scale (Luo et al., 2014). Nitrogen fixation was enhanced during anoxic events in deep geological time (Kuypers et al., 2004). The exuberant growth of ammonia-oxidizing bacteria consumes oxygen in the water column, which would facilitate the N2-fixing enzyme activity (Zehr & Kudela, 2011; Luo et al., 2014; Dong et al., 2019). Similarly, in the northern SCS, the nitrogen fixation has increased since 30 ka indicated by decreasing δ15N value of foraminifera from core MD 12–3433 (Figure 2D). A consistent nitrogen fixation variation trend has occurred throughout the SCS since 30 ka, which might be controlled by similar physical and chemical factors, e.g., stratification. However, the increasing [2]/[3] value from the glacial to the interglacial period in cores from the northern SCS indicates the weakening of ammonia oxidation during the interglacial period (Figure 2B). The decoupling of nitrogen fixation and ammonia oxidation implies that the heterogeneity of regional surroundings between the northern and southern SCS has acted on the ammonia metabolic changes of AOA communities, and resulted in this regional differentiation within the glacial-interglacial timescale (Table 1).
TABLE 1. Core information and the relationship of [2]/[3] vs. δ15N in the southern and northern SCS.
The hydrodynamic processes exhibit discrepancies between the southern and northern SCS under the effect of the EAWM system. In the southern SCS, the surface circulation exhibits a cyclonic structure in winter, driven by the winter monsoon, and Coriolis force and combined with surrounding topographic constraints, causing the upwelling in the southern SCS, which brings nitrogen-limited and phosphorus-rich deep water to the upper layers (Tan & Shi, 2006; Wong et al., 2007; Ren et al., 2017). During glacial periods, the enhanced EAWM provided a positive wind stress curl that built up the cyclonic circulation and strengthened the upwelling, thus lifting the depth of the thermocline and halocline, and leading to a thinner thermocline and halocline (Chen et al., 2011; Dong et al., 2015). During the interglacial periods, the weakening of the EAWM weakened the upwelling, therefore enhancing surface stratification and shifting thermocline and DCM layer downward.
In the northern SCS, however, no significant seasonal upwelling region has been observed. Similar to that in the southern SCS, thermocline depth was deeper during the interglacial period than that in the last glacial period, but this was not because of the weakening of upwelling driven by a weaker EAWM (Figure 3A). Instead, the anticyclonic current loop, which was driven by the EASM dominating this region, drove the thermocline and halocline deeper (Xiu et al., 2010; Guo et al., 2015). Therefore, monsoon-triggered water mixing controls the surface circulation in the northern SCS: in the interglacial period, the strengthening of the EASM enhanced vertical mixing and deepened the thermocline, which, conversely, was thinner and shallower during the last glacial period in the northern SCS. Coincidentally, Li et al. proposed that vertical mixing driven by the EAWM dominantly determined the hydrological structure in the northern SCS over the last 356 ka (Li et al., 2013). Intensification of the EAWM drives stronger vertical mixing, resulting in deeper mixed layer depth (MLD) and a thicker thermocline and halocline, while the weakening of wind turbulence resulted in a shallower thermocline and halocline depth. The evidence indicates that the northern and southern SCS are controlled by different surface circulation systems and thus lead to a different hydrological structure.
FIGURE 3. Biogeochemical characteristics of the northern SCS since 30 ka; (A) ΔT of the cores ODP 1147 (Li et al., 2013) and MD12-3428 (Dong et al., 2018) in the northern SCS; and (B) the total productivity of the core MD01-2904 (He et al., 2008).
Why do different hydrological structures during the glacial and interglacial periods have diverse characteristics in regard of the nitrogen cycle? Factors that affect the growth of shallow-water AOA are considered to be complex and influenced by light and the depth of the upper water column, as well as ammonia concentration and ammonia effectiveness. The AOA prefer to live in the trophic halocline where have adequate ammonia mainly produced by remineralization of organic matter and weak light intensity (Xue et al., 2004; Herfort et al., 2007; Michael Beman et al., 2012; Liu et al., 2018). In the southern SCS, during the glacial periods, the strengthening of the EAWM drove the cyclonic eddy formation and lifted the thermocline and DCM due to the upwelling. Therefore, shallow-water clade AOA communities shrank because the light was more intense in the shallower seawater. In contrast, during the interglacial period, the weakening of the EAWM led to enhanced stratification and weakened upwelling, which shifted the DCM downward. Shallow-water clade AOA, therefore, obtained a steadier water environment and space to survive with weaker light. Additionally, the ammonia concentration was also higher in the deeper layer, which in general resulted in their vigorous growth.
However, in the northern SCS, anticyclonic eddies pushed the nutrient-poor and oxygen-rich surface water down to the deeper water column during the interglacial period. An oxygenated and turbulent water environment is unfavorable for AOA survival. This low-nutrient surface-water mixing failed to stimulate primary productivity during the intergalcial period (Fig. 3B, He et al., 2008), which thus reduced the remineralization-derived carbon dioxide and ammonia for AOA growth, (Ning et al., 2008; Xiu & Chai, 2011; Hu et al., 2014; Guo et al., 2015). Moreover, enhanced export POC flux triggered by anticyclonic eddies has been observed in modern settings (Zhou et al., 2013), which means weaker remineralization in the eddies and hence, lower ammonia and carbon dioxide available to AOA (Figure 4A). In the glacial period, reduced anticyclonic mixing combined with high productivity reinforced the remineralization of fixed organic carbon (Figure 3B), therefore increasing the NH3 and CO2 concentration that supported the shallow-water clade AOA to growth (Figure 4B). In short, reduced anticyclonic activity promoted the stratification, which stimulated the shallow-water clade AOA growth. To some extent, the [2]/[3] ratio can indirectly reflect the relative strength of remineralization in the upper water column because remineralization of organic carbon derived from primary productivity provides both energy and carbon source for AOA, e.g., NH3 and CO2. Higher remineralization, therefore, leads to a higher ammonia oxidation rate, which is embodied in a lower [2]/[3] ratio (Figure 4B).
FIGURE 4. Mechanisms of the relationship between ammonia oxidation and nitrogen fixation in upper waters of the northern SCS since 30 ka. (A) During the interglacial period, EASM induced the formation of anticyclonic eddies which shifted the halocline downward. (B) During the glacial period, weaker EASM reduced the anticyclonic eddies activity and lifted the halocline. PP denotes the primary productivity.
The decoupling of ammonia oxidation and nitrogen fixation in the northern SCS suggests that rather than being a determinant, ammonia oxidation can only affect the efficiency of nitrogen fixation to a certain extent. Factors that shape the nitrogen cycle can be intricate and vary between regions and timescales. The same variation trend of the glacial-interglacial nitrogen cycle in the southern and the northern of the SCS suggests that the nitrogen fixation efficiency may be controlled by the same physicochemical processes, such as thermal stratification, i.e., enhanced nitrogen fixation when the nitricline deepens, which is also consistent with modern observations (Church et al., 2009; Zehr & Kudela, 2011; Luo et al., 2014). Mesoscale physical processes (e.g., mesoscale eddies, temperature, and stratification) have an important effect on marine primary productivity and the microbial community dynamics. In addition, anticyclonic eddies have been proposed to motivate the N cycle in the ocean and enhance the N2 fixation in the subtropical Pacific (Fong et al., 2008; Church et al., 2009) and subtropical Atlantic (McGillicuddy et al., 2007), probably because of the enhancement of diazotroph growth. In the northern SCS, anticyclonic eddies inhibit the growth of shallow-water clade AOA while enhancing the N2-fixing bacteria during the interglacial period, indicating a decoupling between ammonia oxidation and nitrogen fixation. The processes within the N cycle, as well as their influencing factors, are subtle and intricate, and physical factors that shape the biotic community composition are not ignorable (Fong et al., 2008; Church et al., 2009). We proposed that mesoscale eddies might affect the inner cycle of the marine N cycle not even on a temporal and small spatial scale but on the longer geological timescale. Even though the mechanisms behind physical/biological interactions are not well defined (Zehr & Kudela, 2011), determining these physical processes is essential for understanding the primary production and biological responses to climate change and N cycling on a geological timescale.
Previous research found that the variation trend of ammonia oxidation indicated by membrane lipid ratio GDGT-[2]/[3] increased since the last glacial period which coupled with the nitrogen fixation in the southern SCS. However, inverse pattern of [2]/[3] has been found in the northern SCS. The mechanisms of the weakening of ammonia oxidation of shallow AOA in the northern SCS since 30 ka were investigated. We believe that different hydrodynamic processes between the southern and northern SCS triggered by EAM system lead to this difference. From MIS 2 to MIS 1, the strengthening of EASM formed a strong anticyclone, and thus enhanced vertical mixing and deepened the thermocline during the interglacial period in the northern SCS. On one hand, the oxygenated and turbulent water environment is unfavorable for shallow-water AOA growth, and on the other hand, the low primary productivity and enhanced export productivity were also unable to provide sufficient carbon dioxide and ammonia for AOA growth, therefore lead to the decrease of ammonia oxidation since the last glacial period. We proposed that mesoscale eddies might affect the inner cycle of the marine N cycle not even on a temporal and small spatial scale but on the longer geological timescale.
The original contributions presented in the study are included in the article/Supplementary Material, further inquiries can be directed to the corresponding author.
LD conceived this work. MC and XZ contributed equally to this work in collecting data and drafting the manuscript. All the authors contributed to the revising of the manuscript.
This work was supported by the National Natural Science Foundation of China (Grants 42072332, 42122043, 41876076, and 41921006) and the National Key Research and Development Program of China (2020YFA0608300).
The authors declare that the research was conducted in the absence of any commercial or financial relationships that could be construed as a potential conflict of interest.
All claims expressed in this article are solely those of the authors and do not necessarily represent those of their affiliated organizations, or those of the publisher, the editors and the reviewers. Any product that may be evaluated in this article, or claim that may be made by its manufacturer, is not guaranteed or endorsed by the publisher.
Altabet, M. A., Higginson, M. J., and Murray, D. W. (2002). The Effect of Millennial-Scale Changes in Arabian Sea Denitrification on Atmospheric CO2. Nature 415 (6868), 159–162. doi:10.1038/415159a
Altabet, M. A. (2006). Isotopic Tracers of the Marine Nitrogen Cycle. Hdb Env. Chem. 2 (3), 251–293. doi:10.1007/698_2_008
Beatty-Desana, J. W., Hoggard, M. J., and Cooledge, J. W. (1975). Letters to Nature. Nature 255 (5505), 242–243. doi:10.1038/255242a0
Brandes, J. A., Devol, A. H., Yoshinari, T., Jayakumar, D. A., and Naqvi, S. W. A (1998). Isotopic composition of nitrate in the central Arabian Sea and eastern tropical North Pacific: A tracer for mixing and nitrogen cycles. Limnol. Oceanogr. 43, 1680–1689. doi:10.4319/lo.1998.43.7.1680
Canfield, D. E., Glazer, A. N., and Falkowski, P. G. (2010). The Evolution and Future of Earth's Nitrogen Cycle. Science 330 (6001), 192–196. doi:10.1126/science.1186120
Chen, G., Hou, Y., and Chu, X. (2011). Mesoscale Eddies in the South China Sea: Mean Properties, Spatiotemporal Variability, and Impact on Thermohaline Structure. J. Geophys. Res. 116 (6), 1–19. doi:10.1029/2010JC006716
Church, M. J., Mahaffey, C., Letelier, R. M., Lukas, R., Zehr, J. P., and Karl, D. M. (2009). Physical Forcing of Nitrogen Fixation and Diazotroph Community Structure in the North Pacific Subtropical Gyre. Glob. Biogeochem. Cycles 23 (2), a–n. doi:10.1029/2008GB003418
Cline, J. D., and Kaplan, I. R. (1975). Marine Chemistry, 3(1975) 271--299 ©. Mar. Chem. 3(1330), 271–299. doi:10.1016/0304-4203(75)90009-2
Dong, L., Jia, G., Li, Q., Li, L., Shi, J., and Zhang, C. L. (2018). Intact Polar Glycosidic GDGTs in Sediments Settle from Water Column as Evidenced from Downcore Sediment Records. Chem. Geol. 501, 12–18. doi:10.1016/j.chemgeo.2018.09.037
Dong, L., Li, L., Li, Q., Wang, H., and Zhang, C. L. (2015). Hydroclimate Implications of Thermocline Variability in the Southern South China Sea over the Past 180,000 Yr. Quat. Res. 83 (2), 370–377. doi:10.1016/j.yqres.2014.12.003
Dong, L., Li, Z., and Jia, G. (2019). Archaeal Ammonia Oxidation Plays a Part in Late Quaternary Nitrogen Cycling in the South China Sea. Earth Planet. Sci. Lett. 509, 38–46. doi:10.1016/j.epsl.2018.12.023
Du, C., Liu, Z., Kao, S. J., and Dai, M. (2017). Diapycnal Fluxes of Nutrients in an Oligotrophic Oceanic Regime: The South China Sea. Geophys. Res. Lett. 44(22), 11510–11518. doi:10.1002/2017GL074921
Fong, A. A., Karl, D. M., Lukas, R., Letelier, R. M., Zehr, J. P., and Church, M. J. (2008). Nitrogen Fixation in an Anticyclonic Eddy in the Oligotrophic North Pacific Ocean. Isme J. 2 (6), 663–676. doi:10.1038/ismej.2008.22
Guo, M., Chai, F., Xiu, P., Li, S., and Rao, S. (2015). Impacts of Mesoscale Eddies in the South China Sea on Biogeochemical Cycles. Ocean. Dyn. 65 (9), 1335–1352. doi:10.1007/s10236-015-0867-1
Guo, Z. T., Berger, A., Yin, Q. Z., and Qin, L. (2009). Strong Asymmetry of Hemispheric Climates during MIS-13 Inferred from Correlating China Loess and Antarctica Ice Records. Clim. Past. 5 (1), 21–31. doi:10.5194/cp-5-21-2009
Haas, S., Robicheau, B. M., Rakshit, S., Tolman, J., Algar, C. K., LaRoche, J., et al. (2021). Physical Mixing in Coastal Waters Controls and Decouples Nitrification via Biomass Dilution. Proc. Natl. Acad. Sci. U.S.A. 118 (18). doi:10.1073/pnas.2004877118
He, J., Zhao, M., Li, L., Wang, H., and Wang, P. (2008). Biomarker Evidence of Relatively Stable Community Structure in the Northern South China Sea during the Last Glacial and Holocene. Terr. Atmos. Ocean. Sci. 19 (4), 377–387. doi:10.3319/TAO.2008.19.4.377
Herfort, L., Schouten, S., Abbas, B., Veldhuis, M. J. W., Coolen, M. J. L., Wuchter, C., et al. (2007). Variations in Spatial and Temporal Distribution of Archaea in the North Sea in Relation to Environmental Variables. FEMS Microbiol. Ecol. 62 (3), 242–257. doi:10.1111/j.1574-6941.2007.00397.x
Hopmans, E. C., Weijers, J. W. H., Schefuß, E., Herfort, L., Sinninghe Damsté, J. S., and Schouten, S. (2004). A Novel Proxy for Terrestrial Organic Matter in Sediments Based on Branched and Isoprenoid Tetraether Lipids. Earth Planet. Sci. Lett. 224 (1–2), 107–116. doi:10.1016/j.epsl.2004.05.012
Hu, Z., Tan, Y., Song, X., Zhou, L., Lian, X., Huang, L., et al. (2014). Influence of Mesoscale Eddies on Primary Production in the South China Sea during Spring Inter-monsoon Period. Acta Oceanol. Sin. 33 (3), 118–128. doi:10.1007/s13131-014-0431-8
Huguet, C. (2007). TEX86 paleothermometry: proxy validation and application in marine sediments. Utrecht: UU Department of Earth Sciences.
Jia, G., Wang, X., Guo, W., and Dong, L. (2017). Seasonal Distribution of Archaeal Lipids in Surface Water and its Constraint on Their Sources and the TEX86 Temperature Proxy in Sediments of the South China Sea. J. Geophys. Res. Biogeosci. 122 (3), 592–606. doi:10.1002/2016JG003732
Jia, G., Zhang, J., Chen, J., Peng, P. a., and Zhang, C. L. (2012). Archaeal Tetraether Lipids Record Subsurface Water Temperature in the South China Sea. Org. Geochem. 50, 68–77. doi:10.1016/j.orggeochem.2012.07.002
Kim, J.-H., van der Meer, J., Schouten, S., Helmke, P., Willmott, V., Sangiorgi, F., et al. (2010). New Indices and Calibrations Derived from the Distribution of Crenarchaeal Isoprenoid Tetraether Lipids: Implications for Past Sea Surface Temperature Reconstructions. Geochimica Cosmochimica Acta 74 (16), 4639–4654. doi:10.1016/j.gca.2010.05.027
Kuypers, M. M. M., van Breugel, Y., Schouten, S., Erba, E., and Sinninghe Damsté, J. S. (2004). N2-fixing Cyanobacteria Supplied Nutrient N for Cretaceous Oceanic Anoxic Events. Geol 32 (10), 853–856. doi:10.1130/G20458.1
Li, D., Zhao, M., Tian, J., and Li, L. (2013). Comparison and Implication of TEX86 and U37K' Temperature Records over the Last 356kyr of ODP Site 1147 from the Northern South China Sea. Palaeogeogr. Palaeoclimatol. Palaeoecol. 376, 213–223. doi:10.1016/j.palaeo.2013.02.031
Liu, K. K., Su, M. J., Hsueh, C. R., and Gong, G. C. (1996). The Nitrogen Isotopic Composition of Nitrate in the Kuroshio Water Northeast of Taiwan: Evidence for Nitrogen Fixation as a Source of Isotopically Light Nitrate. Mar. Chem. 54 (3–4), 273–292. doi:10.1016/0304-4203(96)00034-5
Liu, Q., Tolar, B. B., Ross, M. J., Cheek, J. B., Sweeney, C. M., Wallsgrove, N. J., et al. (2018). Light and Temperature Control the Seasonal Distribution of Thaumarchaeota in the South Atlantic Bight. Isme J. 12 (6), 1473–1485. doi:10.1038/s41396-018-0066-4
Luo, Y.-W., Lima, I. D., Karl, D. M., Deutsch, C. A., and Doney, S. C. (2014). Data-based Assessment of Environmental Controls on Global Marine Nitrogen Fixation. Biogeosciences 11 (3), 691–708. doi:10.5194/bg-11-691-2014
McElroy, M. B. (1983). Marine Biological Controls on Atmospheric CO2 and Climate. Nature 302 (5906), 328–329. doi:10.1038/302328a0
McGillicuddy, D. J., Anderson, L. A., Bates, N. R., Bibby, T., Buesseler, K. O., Carlson, C. A., et al. (2007). Eddy/Wind Interactions Stimulate Extraordinary Mid-ocean Plankton Blooms. Science 316 (5827), 1021–1026. doi:10.1126/science.1136256
Michael Beman, J., Popp, B. N., and Alford, S. E. (2012). Quantification of Ammonia Oxidation Rates and Ammonia-Oxidizing Archaea and Bacteria at High Resolution in the Gulf of California and Eastern Tropical North Pacific Ocean. Limnol. Oceanogr. 57 (3), 711–726. doi:10.4319/lo.2012.57.3.0711
Molina, V., Belmar, L., and Ulloa, O. (2010). High Diversity of Ammonia-Oxidizing Archaea in Permanent and Seasonal Oxygen-Deficient Waters of the Eastern South Pacific. Environ. Microbiol. 12 (9), 2450–2465. doi:10.1111/j.1462-2920.2010.02218.x
Müller, O., Wilson, B., Paulsen, M. L., Rumińska, A., Armo, H. R., Bratbak, G., et al. (2018). Spatiotemporal Dynamics of Ammonia-Oxidizing Thaumarchaeota in Distinct Arctic Water Masses. Front. Microbiol. 9 (JAN), 1–13. doi:10.3389/fmicb.2018.00024
Ning, X., Peng, X., Le, F., Hao, Q., Sun, J., Liu, C., et al. (2008). Nutrient Limitation of Phytoplankton in Anticyclonic Eddies of the Northern South China Sea. Biogeosciences Discuss. 5 (6), 4591–4619. doi:10.5194/bgd-5-4591-2008
Pelejero, C., and Grimalt, J. O. (1997). Pelejero_and_Grimalt97GCA.pdf. Geochimica Cosmochimica Acta 61 (Issue 22), 4789. doi:10.1016/s0016-7037(97)00280-9
Prahl, F. G., and Wakeham, S. G. (1987). Calibration of Unsaturation Patterns in Long-Chain Ketone Compositions for Palaeotemperature Assessment. Nature 6 146 (330), 367–369. doi:10.1038/330367a0
Qu, T., Du, Y., Gan, J., and Wang, D. (2007). Mean Seasonal Cycle of Isothermal Depth in the South China Sea. J. Geophys. Res. 112 (2), 2–13. doi:10.1029/2006JC003583
Ren, H., Sigman, D. M., Martínez-García, A., Anderson, R. F., Chen, M.-T., Ravelo, A. C., et al. (2017). Impact of Glacial/interglacial Sea Level Change on the Ocean Nitrogen Cycle. Proc. Natl. Acad. Sci. U.S.A. 114 (33), E6759–E6766. doi:10.1073/pnas.1701315114
Robinson, D. (2001). δ15N as an Integrator of the Nitrogen Cycle. Trends Ecol. Evol. 16 (3), 153–162. doi:10.1016/S0169-5347(00)02098-X
Rush, D., and Sinninghe Damsté, J. S. (2017). Lipids as Paleomarkers to Constrain the Marine Nitrogen Cycle. Environ. Microbiol. 19 (6), 2119–2132. doi:10.1111/1462-2920.13682
Schouten, S., Ellen C, H., Schefu, E., and Sinninghe Damste, S. J. (2002). Distributional Variations in Marine Crenarchaeotal Membrane Lipids : a New Tool for Reconstructing Ancient Sea Water Temperatures. Earth Planet. Sci. Lett. 204 (1–2), 265–274. doi:10.1016/s0012-821x(02)00979-2
Sigman, D. M., and Fripiat, F. (2019). “Nitrogen Isotopes in the Ocean,” in Encyclopedia of Ocean Sciences. 3rd ed. (Elsevier), Issue 1, 263–278. doi:10.1016/B978-0-12-409548-9.11605-7
Sturt, H. F., Summons, R. E., Smith, K., Elvert, M., and Hinrichs, K.-U. (2004). Intact Polar Membrane Lipids in Prokaryotes and Sediments Deciphered by High-Performance Liquid Chromatography/electrospray Ionization Multistage Mass Spectrometry-New Biomarkers for Biogeochemistry and Microbial Ecology. Rapid Commun. Mass Spectrom. 18 (6), 617–628. doi:10.1002/rcm.1378
Tan, S., and Shi, G. (2006). Satellite-derived Primary Productivity and its Spatial and Temporal Variability in the China Seas. J. Geogr. Sci. 16 (4), 447–457. doi:10.1007/s11442-006-0408-4
Urakawa, H., Martens-Habbena, W., Huguet, C., de la Torre, J. R., Ingalls, A. E., Devol, A. H., et al. (2014). Ammonia Availability Shapes the Seasonal Distribution and Activity of Archaeal and Bacterial Ammonia Oxidizers in the Puget Sound Estuary. Limnol. Oceanogr. 59 (4), 1321–1335. doi:10.4319/lo.2014.59.4.1321
Wada, E., and Hattori, A. (1976). Natural Abundance of 15N in Particulate Organic Matter in the North Pacific Ocean. Geochimica Cosmochimica Acta 40 (2), 249–251. doi:10.1016/0016-7037(76)90183-6
Wang, P., and Li, Q. (2009). The South China Sea: Paleoceanography and Sedimentology. Springer Science & Business Media, 13.
Wang, T., Ravelo, A. C., Ren, H., Dang, H., Jin, H., Liu, J., et al. (2018). Nitrogen Isotope Variations in the Northern South China Sea since Marine Isotopic Stage 3: Reconstructed from Foraminifera‐Bound and Bulk Sedimentary Nitrogen. Paleoceanogr. Paleoclimatology 33 (6), 594–605. doi:10.1029/2018PA003344
Wong, G. T. F., Ku, T.-L., Mulholland, M., Tseng, C.-M., and Wang, D.-P. (2007). The SouthEast Asian Time-Series Study (SEATS) and the Biogeochemistry of the South China Sea-An Overview. Deep Sea Res. Part II Top. Stud. Oceanogr. 54 (14–15), 1434–1447. doi:10.1016/j.dsr2.2007.05.012
Xiu, P., and Chai, F. (2011). Modeled Biogeochemical Responses to Mesoscale Eddies in the South China Sea. J. Geophys. Res. 116 (10), 1–16. doi:10.1029/2010JC006800
Xiu, P., Chai, F., Shi, L., Xue, H., and Chao, Y. (2010). A Census of Eddy Activities in the South China Sea during 1993-2007. J. Geophys. Res. 115 (3), 1–15. doi:10.1029/2009JC005657
Xu, M. N., Zhang, W., Zhu, Y., Liu, L., Zheng, Z., Wan, X. S., et al. (2018). Enhanced Ammonia Oxidation Caused by Lateral Kuroshio Intrusion in the Boundary Zone of the Northern South China Sea. Geophys. Res. Lett. 45 (13), 6585–6593. doi:10.1029/2018GL077896
Xue, H., Chai, F., Pettigrew, N., Xu, D., Shi, M., and Xu, J. (2004). Kuroshio Intrusion and the Circulation in the South China Sea. J. Geophys. Res. 109 (2), 1–14. doi:10.1029/2002jc001724
Zehr, J. P., and Capone, D. G. (2020). Changing Perspectives in Marine Nitrogen Fixation. Science 368 (6492). doi:10.1126/science.aay9514
Zehr, J. P., and Kudela, R. M. (2011). Nitrogen Cycle of the Open Ocean: From Genes to Ecosystems. Annu. Rev. Mar. Sci. 3, 197–225. doi:10.1146/annurev-marine-120709-142819
Zhang H, H., Liu, C., Jin, X., Shi, J., Zhao, S., and Jian, Z. (2016). Dynamics of Primary Productivity in the Northern South China Sea over the Past 24,000 Years. Geochem. Geophys. Geosyst 12 (17), 4878–4891. doi:10.1002/2016GC006602.Received
Zhang, Y. G., Pagani, M., and Wang, Z. (2016). Ring Index: A New Strategy to Evaluate the Integrity of TEX 86 Paleothermometry. Paleoceanography 31 (2), 220–232. doi:10.1002/2015PA002848
Keywords: archaeal ammonia oxidation, nitrogen fixation, GDGTs, the last glacial period, mesoscale eddies, northern South China Sea
Citation: Chen M, Zhao X, Li D-W and Dong L (2022) Mesoscale Eddy Effects on Nitrogen Cycles in the Northern South China Sea Since the Last Glacial. Front. Earth Sci. 10:886200. doi: 10.3389/feart.2022.886200
Received: 28 February 2022; Accepted: 28 April 2022;
Published: 18 May 2022.
Edited by:
Fangjian Xu, Hainan University, ChinaReviewed by:
Tiantian Tang, Xiamen University, ChinaCopyright © 2022 Chen, Zhao, Li and Dong. This is an open-access article distributed under the terms of the Creative Commons Attribution License (CC BY). The use, distribution or reproduction in other forums is permitted, provided the original author(s) and the copyright owner(s) are credited and that the original publication in this journal is cited, in accordance with accepted academic practice. No use, distribution or reproduction is permitted which does not comply with these terms.
*Correspondence: Liang Dong, ZG9uZ2xpYW5nQHNqdHUuZWR1LmNu
†These authors have contributed equally to this work
Disclaimer: All claims expressed in this article are solely those of the authors and do not necessarily represent those of their affiliated organizations, or those of the publisher, the editors and the reviewers. Any product that may be evaluated in this article or claim that may be made by its manufacturer is not guaranteed or endorsed by the publisher.
Research integrity at Frontiers
Learn more about the work of our research integrity team to safeguard the quality of each article we publish.