- 1Department of Geology, University of Vienna, Vienna, Austria
- 2Department of Geography and Earth Sciences, Aberystwyth University, Aberystwyth, United Kingdom
- 3Institute of Geology, Chinese Academy of Geological Sciences, Beijing, China
- 4School of Earth Science, The University of Western Australia, Perth, WA, Australia
- 5College of Earth Science and Engineering, James Cook University, Douglas, QLD, Australia
- 6Department of Geography, Royal Holloway University of London, Egham, United Kingdom
- 7Géosciences Rennes, UMR6118, Université de Rennes 1, Rennes, France
- 8Ecole et Observatoire des Sciences de la Terre, Centre de Géochimie de la Surface, CNRS-UMR, Strasbourg, France
The deep time (pre-Quaternary) glacial record is an important means to understand the growth, development, and recession of the global cryosphere on very long timescales (106–108 Myr). Sedimentological description and interpretation of outcrops has traditionally played an important role. Whilst such data remain vital, new insights are now possible thanks to freely accessible aerial and satellite imagery, the widespread availability and affordability of Uncrewed Aerial Vehicles, and accessibility to 3D rendering software. In this paper, we showcase examples of glaciated landscapes from the Cryogenian, Ediacaran, Late Ordovician and Late Carboniferous where this approach is revolutionizing our understanding of deep time glaciation. Although some problems cannot be overcome (erosion or dissolution of the evidence), robust interpretations in terms of the evolving subglacial environment can be made. Citing examples from Australia (Cryogenian), China (Ediacaran), North and South Africa (Late Ordovician, Late Carboniferous), and Namibia (Late Carboniferous), we illustrate how the power of glacial geomorphology can be harnessed to interpret Earth’s ancient glacial record.
Introduction
Earth’s stratigraphic record contains a vast deep time archive of climatic swings and ice ages from ca. 2.9 Ga to the present day (Eyles, 2008). The record is typically composed of poorly sorted sediments called diamictites, which can be deposited under a direct glacial influence but are also known to originate in a variety of settings where slope remobilization occurs (cf. Visser, 1997; Arnaud et al., 2011; Busfield and Le Heron, 2016; Nascimento et al., 2016; Le Heron et al., 2017; Kennedy et al., 2018; Kennedy and Eyles, 2020). A far more lucid insight into behaviour of ice masses in deep time may be gained by shifting from traditional sedimentological description and focusing instead on new methods, including semi-quantitative analysis of geomorphological features underlying putative glacial deposits. The aim of this paper is to provide a brief overview of current and new approaches to ancient glacial land surface mapping, highlighting examples of Cryogenian, Ediacaran, Ordovician and Carboniferous age. Concurrently, we present new ice sheet data from Namibia (Late Carboniferous) and Australia (Cryogenian) as well as integrating literature examples. We suggest strategies to tackle the so-called “preservation lottery” in the ancient record, and showcase a remote sensing approach using UAV data and freely available imagery from Bing Maps and Google Earth with wide application to understanding our planet’s deep-time glacial history. This new multi-scale mapping approach to the ancient record gives unprecedented insight into processes operating from the glacier bed through to continent-scale ice sheet dynamics.
Contemporary approaches to ice-sheet mapping and reconstruction leverage satellite imagery, LiDAR mapping and submarine geophysical techniques to generate high resolution, large scale, ice-flow interpretations in a range of settings. For example, recent large projects such as BRITICE (Clark et al., 2021), PATICE (Davies et al., 2020) or DATED-1 (Hughes et al., 2016; Batchelor et al., 2019) produced high resolution Pleistocene ice sheet reconstructions; Margold et al. (2015) employed derived digital elevation models (DEMs) to produce continental-scale flow maps for the Laurentian Ice Sheet, and Kurjanski et al. (2019) revealed insight into Northern Hemisphere ice stream shutdown cycles by mapping shallow marine bedforms. Abundant satellite imagery and LiDAR data from many sources now provides opportunity to additionally map sediment-landform systems in formerly glaciated landscapes (Möller and Dowling, 2016). In contrast to their application in the modern/Pleistocene realm, application in Palaeozoic or Precambrian glacial landscapes are nascent, but offer great potential. As an example, traditional aerial photograph and field-based mapping of subhorizontal plateaux exposing Late Ordovician glacial strata in North Africa (Beuf et al., 1971) was enhanced using satellite image interpretation (e.g., Moreau et al., 2005; Le Heron et al., 2006; Le Heron and Craig, 2008; Moreau, 2011; Girard et al., 2012; Deschamps et al., 2013), and additional basin-scale subsurface datasets (Deschamps et al., 2013; Battaler et al., 2019). The satellite mapping approach thus allowed for mapping of much larger areas than previously possible with aerial photographs. Here, satellite imagery applied to large scale (hundreds to thousands of kilometres) mapping of deep-time ice sheets is possible owing to 1) the desert landscape and 2) a general lack of tectonic deformation in the Saharan Basins.
Study of deep time ice-ages offers insight into the meltback or recessional phases of glaciation and consequent impact on landscape. This understanding is pertinent in predicting and planning for outcomes in the active meltback phase of the current warming climate. Aspects of glacial meltwater systems, striated pavements, and an array of subglacial bedforms are better understood by integrating aerial photographs and digital elevation models (DEMs) with traditional field data. The use of Uncrewed Aerial Vehicles (UAVs) provides a cost-effective solution to the acquisition of aerial photographs and DEMs that allow detailed mapping, typically to the resolution of about 1 cm (Chandler et al., 2018; Śledź et al., 2021). In Quaternary settings, the deployment of UAVs and other remotely operated vehicles to understand glacial land systems and glacier forefields is now routine (Chandler et al., 2018; Śledź et al., 2021; Normandeau et al., 2021). However, very few studies have deployed UAVs to understand deep-time glacial processes and geomorphology (for exceptions see Le Heron et al., 2019a, 2019b; Chen et al., 2020). UAVs have only very recently been deployed to understand aspects of Ediacaran (ca. 600 Ma), Ordovician (ca. 444 Ma), and Late Palaeozoic Ice Age (ca. 300 Ma) successions, and the integration of satellite images and aerial photographs offers exciting prospects to study the ancient record.
The Satellite Revolution
Secrets of the Sahara: Late Ordovician Glaciers in the Tassili N’Ajjer Plateau, Algeria
Mapping of the Late Ordovician glacial successions in central Sahara in the 1950s and 60s resulted in Beuf et al.’s (1971) seminal monograph documenting regional ice sheet dynamics. They mapped the spatial distribution of striated pavements and their outcrop character, establishing a regional south to north ice flow direction (Figure 1A), and included exquisite maps and images of large palaeovalleys such as that at Djanet, and sinuous channel belts. Palaeogeographic interpretations with significant emphasis on the glacial geomorphology were published by Rognon et al. (1972) and mapping clarified the distribution of large palaeovalley incisions up to several hundred metres deep and hundreds of metres wide (e.g., the Iherir palaeovalley; Beuf et al., 1971), together with smaller (m-to tens of m-scale) sandstone-filled ribbon-like channels, later interpreted as the topset deposits to large-ice contact deltas (Ghienne et al., 2010; Girard et al., 2012). Although the palaeovalleys are reinterpreted as tunnel valley networks (Deschamps et al., 2013), their lateral distribution was established in Beuf et al. (1971), with a similar S-N orientation to the glacial striations, and they were later clearly characterised as ice-marginal features in Girard et al. (2012) (Figure 1B).
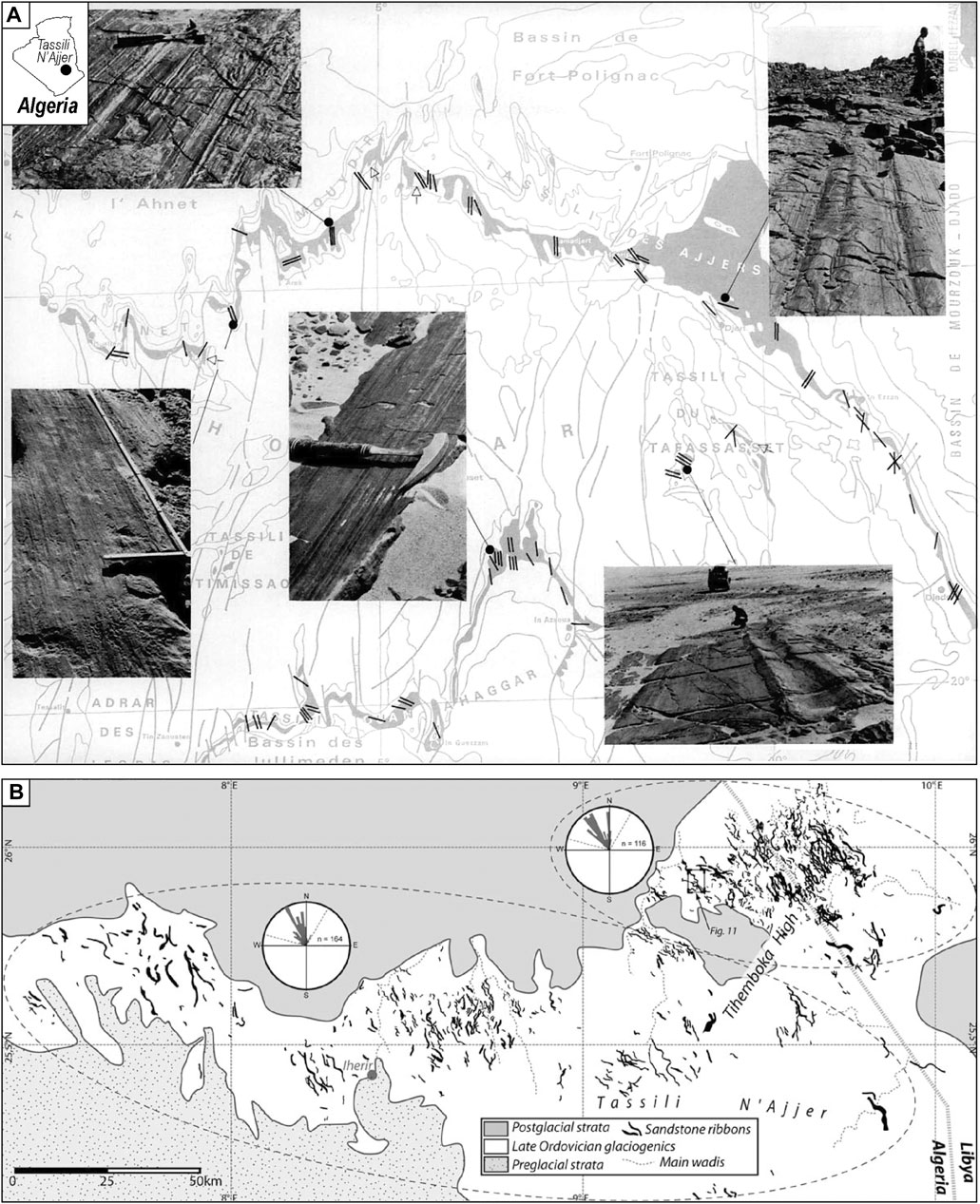
FIGURE 1. Historical successes in capturing big picture glacial geomorphology in the ancient record. (A) The mapping efforts of Beuf et al. (1971) are a landmark achievement in the documentation of the Late Ordovician glacial record of southern Algeria. This map (reproduced with permission) shows the distribution of soft-sediment striated surfaces across the Tassili N’Ajjer (shown here as the Tassili des Ajjers) plateau (represented by simple, black parallel lines). These authors did substantial fieldwork in the region, and the photos show five separate examples of these soft-sediment striated surfaces over a wide area. (B) Mapping in the Tassili N’Ajjer 40 years later (Girard et al., 2012), with focus of meltwater structures (sandstone ribbons in this image). The impressive, dense network of structures results from high fidelity aerial photograph and satellite image mapping made possible thanks to the undeformed nature of the substrate, the gently dipping plateau, and the lack of vegetation in the central Sahara. Reproduced with permission of the Geological Society of London.
Beuf et al. (1971) used aerial photographs to map large areas (tens of square kilometres), but it was not until satellite data became available that regional-scale mapping became realistic. For example, Moreau et al. (2005) harnessed the insight from the satellite imagery to map palaeo-ice stream flowsets across the Algerian-Libyan border and Ghienne et al. (2007) developed larger-scale reconstructions of palaeo-ice streams across North Africa for the first time. This culminated in large-scale ice sheet reconstructions in which palaeo-ice streams and meltwater systems (tunnel valleys) were integrated into a single map (Le Heron and Craig, 2008).
These second-generation models were derived from integration of satellite imagery with seismic and borehole data collected in the course of petroleum exploration, underpinned by the spatial relationships between glacial palaeo-landforms established by Beuf et al. (1971) in the Tassili N’Ajjer. Nevertheless, at the time of this pioneering work, costly individual LANDSATTM images were purchased on an individual basis, inhibiting “blue skies” research. The advent of freely available, global, high resolution satellite imagery now provides unparalleled opportunity for applying these contemporary approaches in many new hitherto understudied sites.
Observing Traces of Cryogenian Glaciers From Space and the Pussycat Palaeovalley
The Kimberley region in Australia (Figure 2) has much to reveal in terms of the geometry and potential behaviour of Neoproterozoic ice masses. Continental-scale glaciation across northwestern Australia was first documented through regional mapping programs in the 1960s and 1970s (Perry and Roberts, 1968; Dow and Gemuts, 1969; Gellatly et al., 1975). The Cryogenian Walsh Formation in the west Kimberley, interpreted as a Marinoan equivalent glacial deposit (Grey and Corkeron, 1998; Corkeron 2007) represents the western-most extent of grounded ices sheets, preserving lodgement till, grounding line and subglacial outwash deposits (Corkeron, 2011). Ice-scoured quartzite basement is documented from multiple striated pavements in three areas underlying Walsh Formation tillite (Perry and Roberts, 1968; Corkeron 2008) indicating south-southwest directed regional ice flow. This trend is consistent with provenance studies and ice-flow interpretations across northern Australia from striated pavements beneath correlated Marinoan glacial deposits (Dow and Gemuts, 1969; Dow, 1965; Dundas et al., 1987; Corkeron, 2008) suggesting an ice source from and unknown northern neighbour.
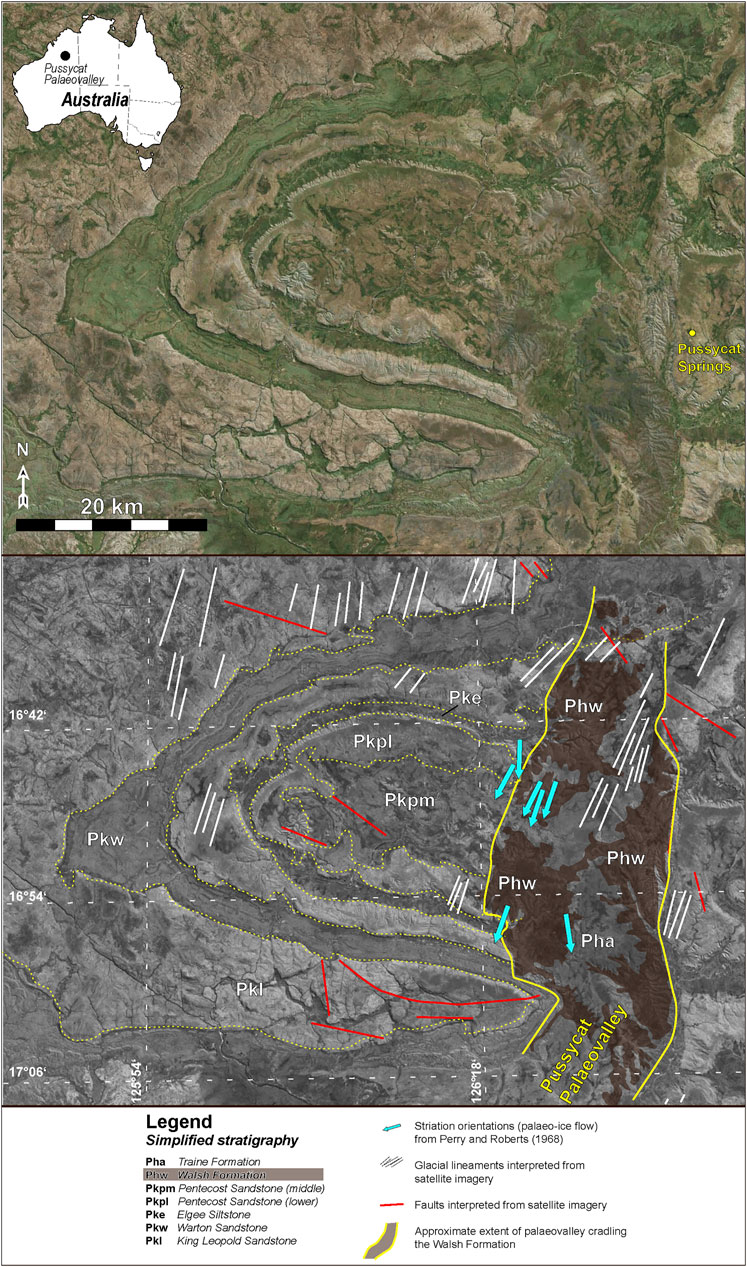
FIGURE 2. A newly discovered Cryogenian glaciated land surface in Australia. Bing Maps image with corresponding interpretation showing a zone to the west of Pussycat Springs, Kimberley, Western Australia of folded Mesoproterozoic strata overlain by the Walsh Formation (a Cryogenian diamictite). Interpretation of this ancient land surface shows the location and orientation of measured striated pavements with ice flow directions indicated (data from Perry and Roberts, 1968), together with interpreted lineaments of probable glaciogenic origins observed on the satellite imagery. The latter cross cut many units underneath the Walsh Formation. The geometry of the Walsh Formation outcrop is notably confined to a belt of N-S oriented strata, which we herein interpret as a possible palaeovalley (the Pussycat Palaeovalley). Non-glacial lineaments (faults) are distinguished also.
Whilst evidence for a glacial record is convincing at outcrop, fresh perspectives can be gained through examination of open source aerial/satellite images (Bing Maps) of the Hann River (Figure 2). The district adjacent to pavements described by Perry and Roberts (1968) reveals features consistent with a previously unrecognised ice-incised palaeovalley constraining the distribution of the glacigenic Walsh Formation in this area. Parallel ridges and grooves of metre to 10s of metre-scale incise Mesoproterozoic basement surfaces lie in a topographic depression (Figure 2) immediately below the Walsh Formation. These prominent N-S oriented bedrock lineations are interpreted as subglacial lineations (such as rock drumlins, whalebacks, roche moutonnées) whose precise genesis should be confirmed with detailed field investigation, but are consistent with documented outcrop striation orientations (Perry and Roberts, 1968). North-south lineation is absent from the Walsh Formation landforms. Additionally, the Walsh Formation shows both regional and local angular unconformity with the regionally folded underlying Mesoproterozoic succession. In the Hann River district, the Walsh Formation is broadly flat-lying with roughly parallel north-south trending bounding contacts onlapping basement and confining the Walsh Formation distribution to a ∼13 km wide belt (Figure 2).
Based on these local features and regional relationships, it is proposed that the Walsh Formation infills a palaeo-valley, here named the Pussycat Palaeovalley on account of the proximity of Pussycat Springs. We tentatively propose that this region exhibits a complex land surface, resulting from 1) folding of earlier Mesoproterozoic strata, 2) development of subglacial lineations, and 3) incision and infill of the Pussycat Palaeovalley.
Anatomy of an Exhumed Carboniferous Glacial Landscape in Chad
The Ennedi Plateau of northern Chad exposes excellent evidence of palaeo-ice stream networks clearly visible on freely accessible aerial/satellite images hosted on Bing Maps, Google Earth or similar platforms. These palaeo-ice stream networks broadly belong to the Late Palaeozoic Ice Age (LPIA) although the age constraints are loose (Le Heron, 2018). The Ennedi region consists of a sandstone plateau, dipping gently to the north into the Kufra Basin of Libya (Figure 3A). New investigations show belts of mega-scale glacial lineations (MSGLs) covering a much wider zone than previously recognised in Le Heron (2018), extending at least 300 km across the plateau (Kettler et al., in review). Here, utilizing freely available satellite images (Bing Maps) we illustrate some of these newly discovered features (Figure 3B).
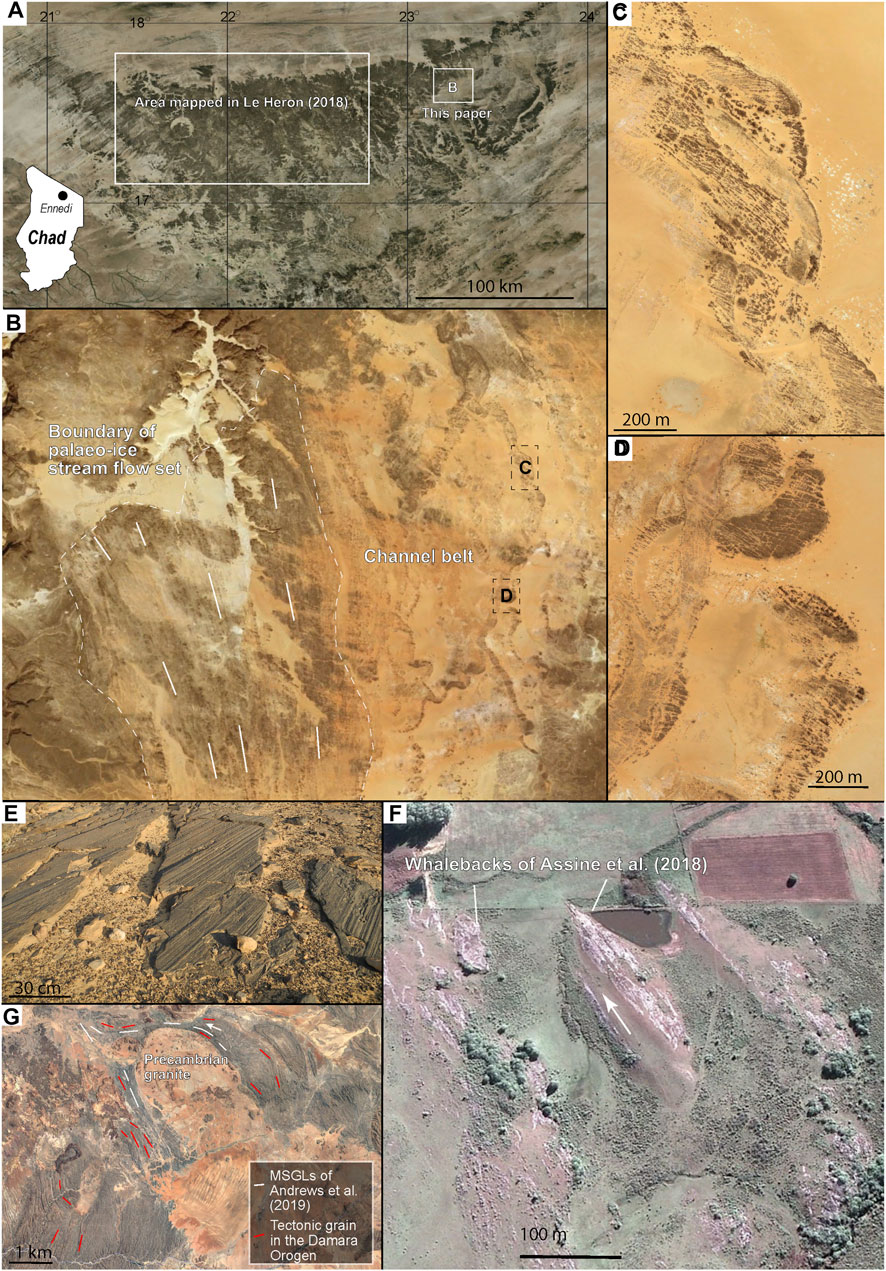
FIGURE 3. The interpretation of Late Palaeozoic Ice Age (LPIA) glacial land surfaces from satellite data. (A) The Ennedi plateaux of northern Chad, showing the mapped location of the palaeo-ice stream network documented in Le Heron (2018), and its eastward continuation (this study). (B) Interpreted palaeo-ice stream flow set with mega-scale glacial lineations (MGSLs) to the left of the image, and an extensive channel belt to the right. The location of the images (C,D) are highlighted in the small rectangles. (E) Soft-sediment striations at outcrop in the Ennedi plateaux. Image courtesy of Ursula Steiner, whose photo was taken at 17.623°N 22.777°E. (F) Google Earth image of whalebacks published in Assine et al. (2018)- hard bedrock structures in the LPIA of northern Uruguay. See Assine et al. (2018) for precise location information. (G) The zone of the Damara Orogen, central Namibia, featured in Andrews et al. (2019). These authors interpreted MGSLs, yet here we caution that these are parallel to the tectonic grain of the orogen, underscoring that the utmost care must be taking when mapping and interpreting deep time glacial land surfaces from satellite imagery. See Andrews et al. (2019) for precise location information.
MGSLs are organised into discrete flow sets measuring up to 15 km wide and >50 km long (Figure 3B), which traverse shallowly-dipping sandstone plateaux. These are separated by topographically elevated regions of the plateaux where MSGLs are absent and could therefore be interpreted as inter-stream areas. Together with the previously mapped zone, the network of palaeo-ice streams is now known to extend over ∼200 km from west to east. Importantly, palaeo-ice stream flow sets occur adjacent to both braided (Figure 3C) and meandering (Figure 3D) channel systems. The channels are set in positive relief with respect to the surrounding desert plain and are traversed by fracture sets suggesting that they are ancient structures that have undergone burial and lithification. The sand-filled channel systems are interpreted to have initially scoured a soft substrate (mud) prior to burial, lithification, then uplift and exposure at the present-day land surface, with subsequent aeolian deflation explaining the removal of interchannel material. We suggest that the channel networks are incompatible with subglacial meltwater, because very extensive freely meandering river belts are not typical in the confining conditions beneath an overlying ice sheet. Instead, the channel belts might either record deposition in a proglacial setting accompanying the retreat of LPIA ice masses in this part of Africa, or simply a postglacial river system. Much remains to be learned from the Ennedi plateau, and little fieldwork has been done thus far. However, we also note the presence of soft-sediment striated surfaces at outcrop from within a belt of MSGLs (Figure 3E). These surfaces are identical to those described from other Palaeozoic outcrops (e.g., Le Heron et al., 2019b, 2020). This discovery solidifies the interpretation that the MSGLs are glaciogenic and not formed through another process.
These recent advances in mapping and interpretation of LPIA land surfaces and ancient glaciogenic landforms (Le Heron, 2018) are leading to exciting new discoveries. A network of glacial valleys and fjords has recently been mapped in the Kaokoland region of northern Namibia (Dietrich et al., 2021) whose pattern is striking on satellite images, as highlighted by the Earth observatory of the NASA (https://earthobservatory.nasa.gov/images/148822/fossil-fjords-in-namibia).A very convincing exhumed LPIA palaeo-landscape in Uruguay was identified by Assine et al. (2018) (Figure 3F), where whalebacks that are cut into granite are clearly visible on Google Earth imagery. A similar study in the Damara Orogen of central Namibia (Andrews et al., 2019) (Figure 3G), however, identified striking similarities between the orientation of the tectonic grain of the Damara Orogen and the interpreted glacial lineaments, highlighting the care needed to differentiate glacial geomorphology from other features in complex basement terrain.
Supercharging Established Aerial Photography With a Drone Mapping Approach
The Methods
The application of UAV imagery surveys (drone mapping) to previously identified glacial landscapes, not only expands our capacity for detailed interpretations at scale, but provides a digital record of ancient landscapes under threat from urbanisation and development. In complex topography and deformed strata (in contrast to planar glacial pavements) the integration of UAV aerial photography with 3D rendering using off-the-shelf software generates high resolution 3D models across landscape scales that were previously unattainable. These models greatly enhance the interpretative resolution of glacial processes in complex landscapes. In this section, we provide three examples where the aerial photography approach enhances our understanding of Ediacaran and Late Palaeozoic Ice Age (LPIA) palaeo-geomorphology and thereby palaeo-ice flow behaviour. The workflow to produce the images is straightforward, and is explained in detail in Le Heron et al. (2019a), but can be summarized as follows. Photographs are taken from an UAV with approximately 60% overlap, and are imported and aligned automatically in Agisoft Metashape. From these, point clouds are generated, a mesh is built from triangulating the points, and texture added. The result is a high quality 3D outcrop model. For mapping purposes an orthomosaic is then generated together with a digital elevation model (DEM). These data are then layered and multiplied together in QGIS, allowing the power of both elevation data and photography to be harnessed and incorporated into a single image.
A Bird’s Eye View of the Base of Ediacaran Ice Sheets
Along the north China Craton, glacially striated surfaces beneath the Luoquan Formation of probable late Ediacaran age were first described in detail by Guan et al. (1986). Drone technology employed by Chen et al. (2020), allowed for further detailed mapping and provides a digital archive of these rare palaeo-landforms, now threatened by increasing road-network development. Likewise, Le Heron (2019a) produced aerial images and map interpretations for the Shimengou striated pavement outcrop in the Pingdingshan region (Figure 4) from UAV imagery. This study demonstrated the ability to interpret high resolution “p-forms” which form through substantial metwater involvement at the ice-bed interface on hard, consolidated bedrock. The method also allowed for regional discrimination of zones defined by well developed, moderately developed, and poorly developed or absent striations.
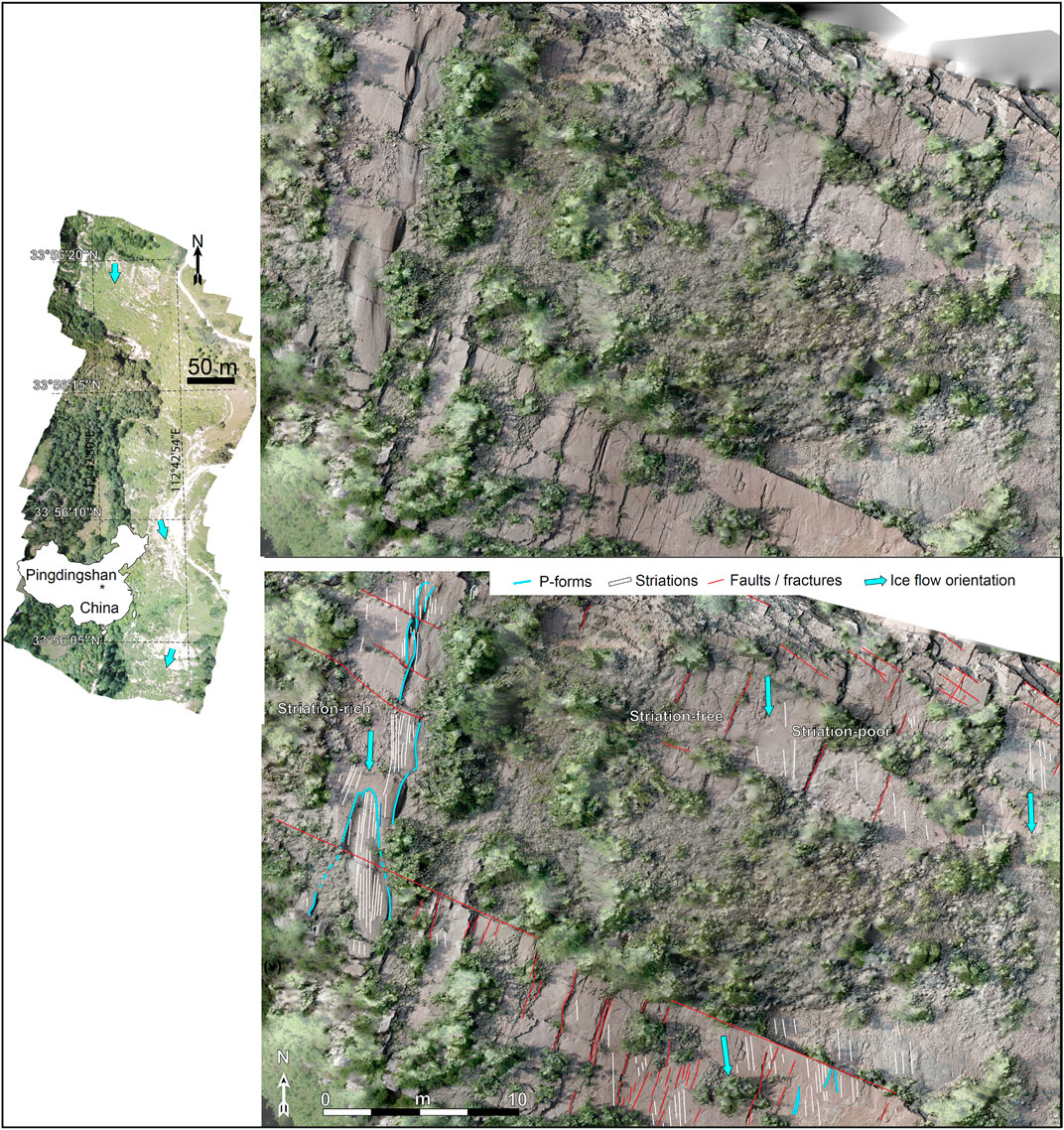
FIGURE 4. The Pingdingshan striated pavement of Ediacaran age, central China, from Le Heron et al. (2019a). The image derives from data collected by a DJI Mavic Pro UAV in June 2018 as follows. A semi-transparent orthophoto has been draped over a digital elevation model to that small scale topographic differences are emphasised, thereby assisting geomorphic mapping. The abundance of p-forms on this surface highlights the role of subglacial meltwater is sculpting m-scale topography into lithified sandstone basement.
The spatial variation in striation development, as mapped from a bird’s eye perspective, may provide fine resolution insight into the spatial variability of subglacial erosion processes. For example, the ubiquitous surface polish preserved over the outcrop surface may record the precipitation of a subglacial silica “gel” that “sealed in” the striations and provided them some protection from later erosion, as proposed for analogous Pleistocene surfaces in the United States (Siman-Tov et al., 2017).
As a global archive, glacially striated surfaces of Ediacaran age are rare: probably the best examples come from the Henan Province in the central China Orogen and in the Kimberley region of Western Australia (Corkeron, 2008), although there are published interpretations of striated surfaces of this age from the Moelv Formation (Baltica), Kahar Formation (Iran), Ouarzazate Grp (Morocco), and the Långmarkberg Fm (Baltica) (Germs, 1972; Hambrey and Harland, 1981; Arnaud et al., 2011; Vandyk et al., in 2021). Thus, the digital documentation of these rare palaeo-landforms from an aerial view is essential to preserve the record of these structures for posterity.
“Glacial Unconformities” in Soft-Sediment Re-Imagined as Thin Stratigraphic Archives
Until recently, glacial surfaces such as in the Karoo Basin in South Africa were viewed simply as erosional unconformities, and the processes associated with their formation were attributed to general models of subglacial erosion and deformation. Reappraisal of landforms and their spatial distributions using UAV-sourced low cost aerial photographs to supplement field observations offers new insight into a diversity of subglacial process as preserved by newly described cross-cutting landform structures. These new interpretations indicate a more complex interaction of processes and glacial phases associated with the Late Palaeozoic Ice Age (LPIA) in South Africa than was previously understood.
Visser (1997) summarised the LPIA ice flow record of South Africa as a series of highland valley glaciers flowing northward and southward into the Karoo Basin, thereby joining trunk ice streams that flowed westward into southern Brazil and Argentina (Figure 5A). In the Karoo Basin, hard bedrock striated surfaces are recorded in multiple basin margin locations, such as in Douglas (du Toit, 1954) at Nooitgedacht near Kimberley (Visser and Loock, 1988) and in KwaZulu-Natal (Dietrich and Hofmann, 2019). However, soft-sediment striated surfaces are also recorded (e.g., in Oorlogskloof; Visser, 1990) and most likely represent basin-marginal and intra-basinal equivalents, respectively. The soft-sediment striated surfaces formed preferentially where sediment accumulated and ice masses overrode unconsolidated substrate. For example, Visser (1990) interpreted ∼1–5 m wide bulbous structures that cross-cut the Oorlogskloof pavement as soft sediment deformation structures formed during the retreat phase of the ice mass.
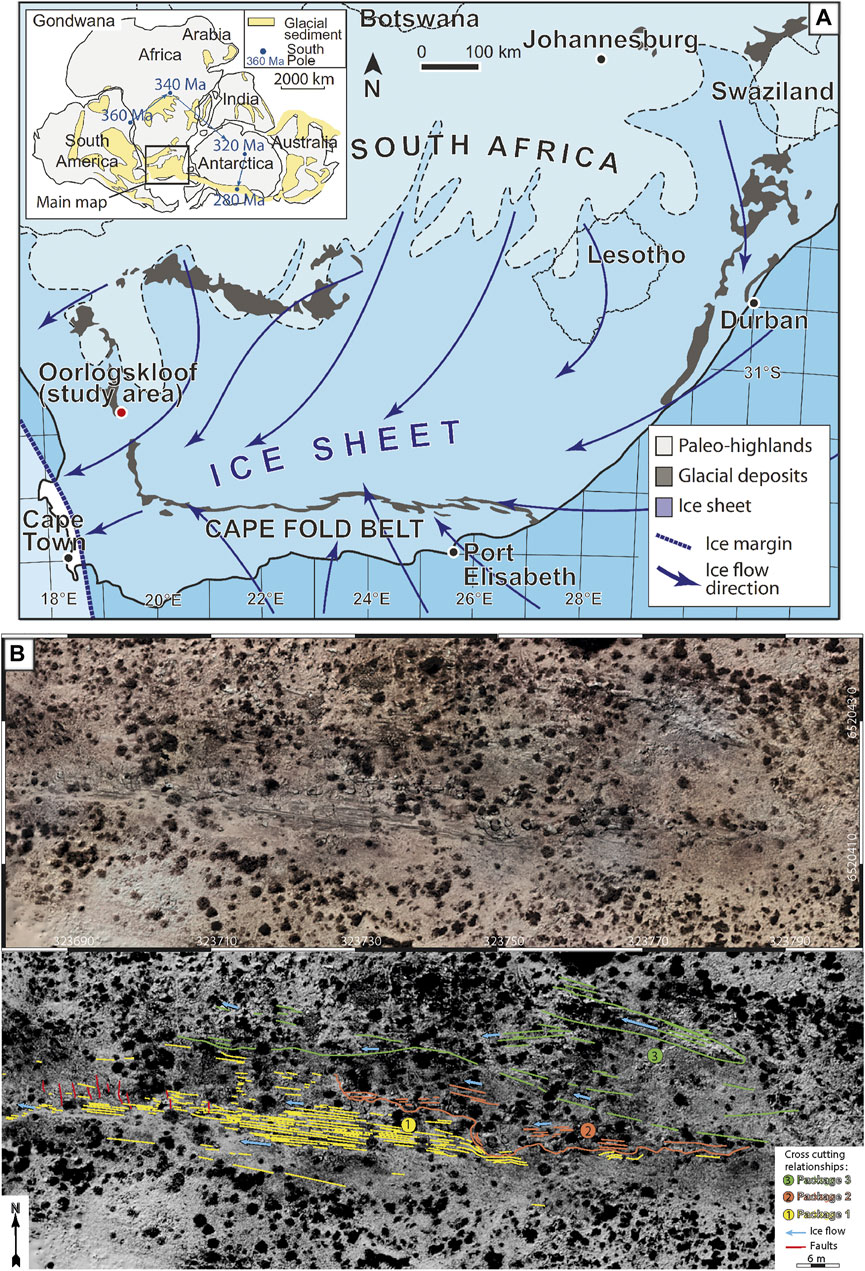
FIGURE 5. (A) Paleogeographic map of South Africa during the late Paleozoic ice age, and outline of ice-sheet flow character, based on Visser (1997), Lopez-Gamundi and Buatois (2010). Study area at Oorlogskloof is situated at the western margin of the Karoo Basin. (B) Bird’s eye view of the Oorlogskloof glacial pavement of South Africa (top) together with an interpretation of the surface and the recognition of cross-cutting relationships (bottom). The bird’s eye view consists of drone data, namely an orthophoto layered over a digital elevation model. From Le Heron et al. (2019b).
Rather than viewing the Oorlogskloof surface as a type of “unconformity” recording subglacial erosion and deformation, recent reappraisal using UAV imagery identified and mapped three crosscutting suites of structures, each exhibiting flutes and striae in slightly different orientations (Le Heron et al., 2019b). The crosscutting relationships (Figure 5B) indicate a three-phase model whereby 1) deformation beneath a grounded ice mass was followed by 2) decoupling and transition to a floating ice margin, and finally 3) renewed grounding and flute development. Whilst the earlier interpretation of variable coupling (Visser, 1990) had merit, viewing the retreat phase as decoupling and sudden retreat presented an oversimplified view, mostly due to the lack of a bird’s eye view.
Supplementing field observations with UAV imagery facilitated this new interpretation, because packages of units with striations in different orientations could be mapped easily and at scale. By confirming the existence of three cross-cutting packages on a soft-sediment striated pavement, the stratigraphy can be demonstrated. Thus, this approach suggests that these surfaces should not be regarded as representing “unconformities” or “bypass”, but rather are valuable archives of stratigraphic information allowing for an improved understanding of grounding and ultimately retreat behaviour of Palaeozoic ice masses.
The Fjords of Gondwana and Their Geomorphology
Spectacular palaeovalley systems are a well-known feature of the LPIA, and perhaps the most extensively documented of these in recent decades have been the palaeofjords of Argentina (Aquino et al., 2014; Kneller et al., 2004; Dykstra et al., 2006; Valdez Buso et al., 2017; 2017, 2021). Notably, quantitative data and cross sectional profiles have recently been published for the Vichigasta palaeovalley, and their geometry characterised using 12.5 m resolution DEMs produced from the ALOS-PALSAR sensor (Valdez Buso et al., 2017). Small-scale, but spectacular, hard bedrock glacial landforms were also described by Bussert (2010) in Ethiopia. By comparison, though, the palaeovalleys of southern Africa have attracted far less modern study. In Namibia, Martin and Schalk (1959) observed that patches of diamictite belonging to the Dwyka Formation occur within modern-day valleys occupied by ephemeral rivers, such as the Hoarusib valley (Figure 6A). Moreover, they observed that some of the modern-day valley sides exhibited outstanding evidence for glacial abrasion via smooth and polished bedrock surfaces and, locally, striated pavements. This network of rivers follows a complex path through predominantly Neoproterozoic and older bedrock of the Damara Belt. Whilst modern geochronological constraints on the northern Namibian rocks are missing, in neighbouring South Africa a number of deglacial cycles straddling the Late Carboniferous-early Permian boundary have been constrained using U-Pb detrital zircon geochronology (Griffis et al., 2019). The co-occurrence of diamictite within the modern-day valleys motivated Martin (1981) to propose that they were at least 300 million years old, with his mapping of interpreted palaeovalley pathways and diamictite distribution showing a complex, interconnected network.
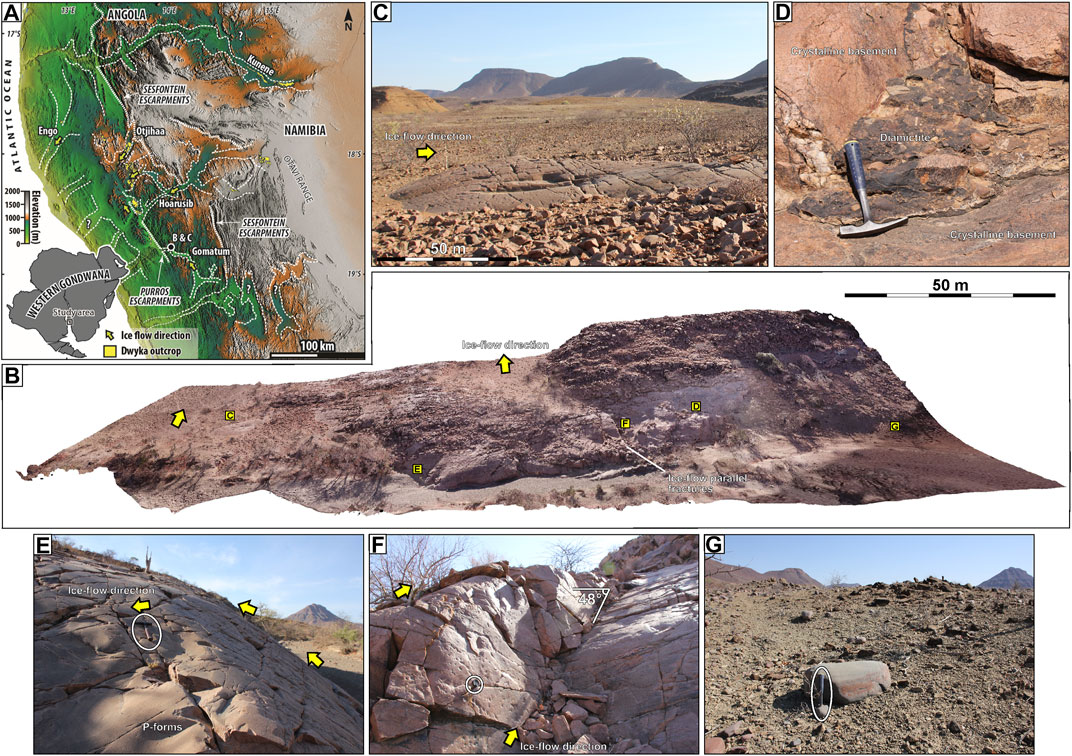
FIGURE 6. Digital outcrop model of a late Carboniferous roche moutonée in Namibia, supplemented by field photographs. In this region, Martin and Schalk (1959) were the first to document bedrock grooves and polished, striated crystalline basement carved during the Late Palaeozoic Ice Age. (A) Map of the fjord network of northern Namibia, showing location of the example and palaeo-ice flow directions. (B) Three dimensional model produced from photographs taken by a DJI Mavic Pro UAV in September 2019. The image shows a large, high relief bedrock feature with a very steep stoss slope. The location of other features in this figure are indicated by the lettering, together with the Late Carboniferous ice flow direction. (C) Small roches moutonée which allows palaeo-ice flow orientation to be confidently indicated. (D) Typical relationship between crystalline basement and (fragmentary) diamictite. The latter is plastered onto high angle slopes yet is remarkably well preserved. (E) P-forms developed on the steep stoss slope (ice flow direction indicated). (F) Glacial polish developed on a steep slope. (G) In present lower lying areas, large facetted, striated boulders weather out from diamictite.
In the regional Gondwanan palaeogeographic context, the Namibian palaeovalleys would have drained toward the west draining into the Paraná Basin of Brazil. There, in the southernmost Paraná Basin, similar palaeovalleys have been recognised and interpreted as fjords by some workers (Tedesco et al., 2016), whereas other workers have found little evidence for glacial fill in neighbouring valleys (Fedorchuk et al., 2019), questioning whether they all record the same process of incision, whether they testify to a more complex pattern of glaciation than previously thought, or raising the spectre of much older incisions that were then further exhumed by valley glaciers. New work on the palaeovalleys in northern Namibia has also incorporated analysis of the sedimentary fill, which when integrated with regional geomorphology suggests the recession of regional plateau ice fields, incision of the valleys, and their subsequent transgression and transformation to a fjord network (Dietrich et al., 2021). The existence of fjords in Namibia to some extent mirrors the situation in Argentina (where palaeovalleys are interpreted similarly: e.g., Aquino et al., 2014; Kneller et al., 2004; Dykstra et al., 2006; Valdez Buso et al., 2017, 2021). However, the Namibian outcrops are particularly amenable to mapping in accessible terrain.
A 3D model assembled from 200 drone photographs reveals aspects of the glacial geomorphology of the easternmost part of the fjord network (Figure 6B). The focus here is to demonstrate the value of the approach for future studies. The drone imagery was collected using a DJI Mavic Pro aircraft in September 2019 and processed using Agisoft Metashape to produce a rendered 3D model. Airborne imagery reveals the geometry of the present-day topographic surface which consists of a large granitic hill, smoothed by the westward flow of confined valley glaciers (Dietrich et al., 2021; Figure 6A). At this scale, the westward flow is supported by the presence of smaller scale roches moutonnées which have a distinctive asymmetric profile (Figures 6B,C). Rarely, diamictite is found across the surface in pockets, locally plastered in sharp relief onto the topography (Figures 6B,D). Elsewhere, gently undulating p-forms are present over the granite bedrock, which ubiquitously exhibit a polished character (Figures 6B,E). Locally, on a 48° sloped surface, the glacial polish continues across ice-flow parallel fractures in granite (Figure 6F). The continuation of glacial polish over the entire outcrop testifies that the steep slopes were generated during or prior to the LPIA rather than by modern weathering. In lower-lying areas (Figures 6B,G), polished, faceted and striated carbonate boulders weather out over the desert plain. Collectively, the data confirm the presence of a substantial inverse bedrock slope. Given the context, it seems most likely that the patchy diamictite was deposited in irregular subglacial depressions. This might imply either exploitation of a previously weathered, jointed surface, or alternatively subglacial ripping (e.g., Hall et al., 2020) followed by superimposition of the glacial polish (Siman-Tov et al., 2017). This example highlights the advantage of integrating aerial photographs with a 3D model (Figure 6B) in showing 1) the broader lateral relationships between phenomena documented at outcrop and 2) the geometry of glaciated bedrock can be characterized more accurately than from a ground-level perspective.
Discussion: The Preservation Lottery Through Geological Time
Small Scale (<1 m) Challenges
From the ancient record, in particular the exceptional examples discussed above from northern and southern Africa, Australia and China, field studies focussed on isolated outcrops that expose striated surfaces. These surfaces are recognisable as they preserve a sheen, striations and other cm to mm-scale features that are identical to their modern counterparts. For example, a striated surface scoured more recently than 1851 in Kauntertal, Austria, is indistinguishable from a 300-million-year-old, Late Carboniferous surface in northern Namibia (Figures 7A,B). The early Permian striated surface of Hallett’s Cove, South Australia, preserves a rich fidelity of structures, including crosscutting striations and chattermarks; a similar delicate record of crosscutting relationships is contained within the polished surface of Ediacaran age in Shimengou, central China (Figures 7C,D). Taking the early Permian striated pavements of South Australia as an example, modern stream flow plays a role in the erasure of evidence for subglacial structures on a striated pavement at Glacier Rock (Figure 7E). We argue that the loss of surface veneer through weathering is a critical problem in the “preservation lottery.” In Ontario, Pleistocene striated bedforms show loss of striations through weathering (Figure 7F). A similar weathering effect has been observed on both Cryogenian and Late Ordovician striated pavements (Figures 7G,H).
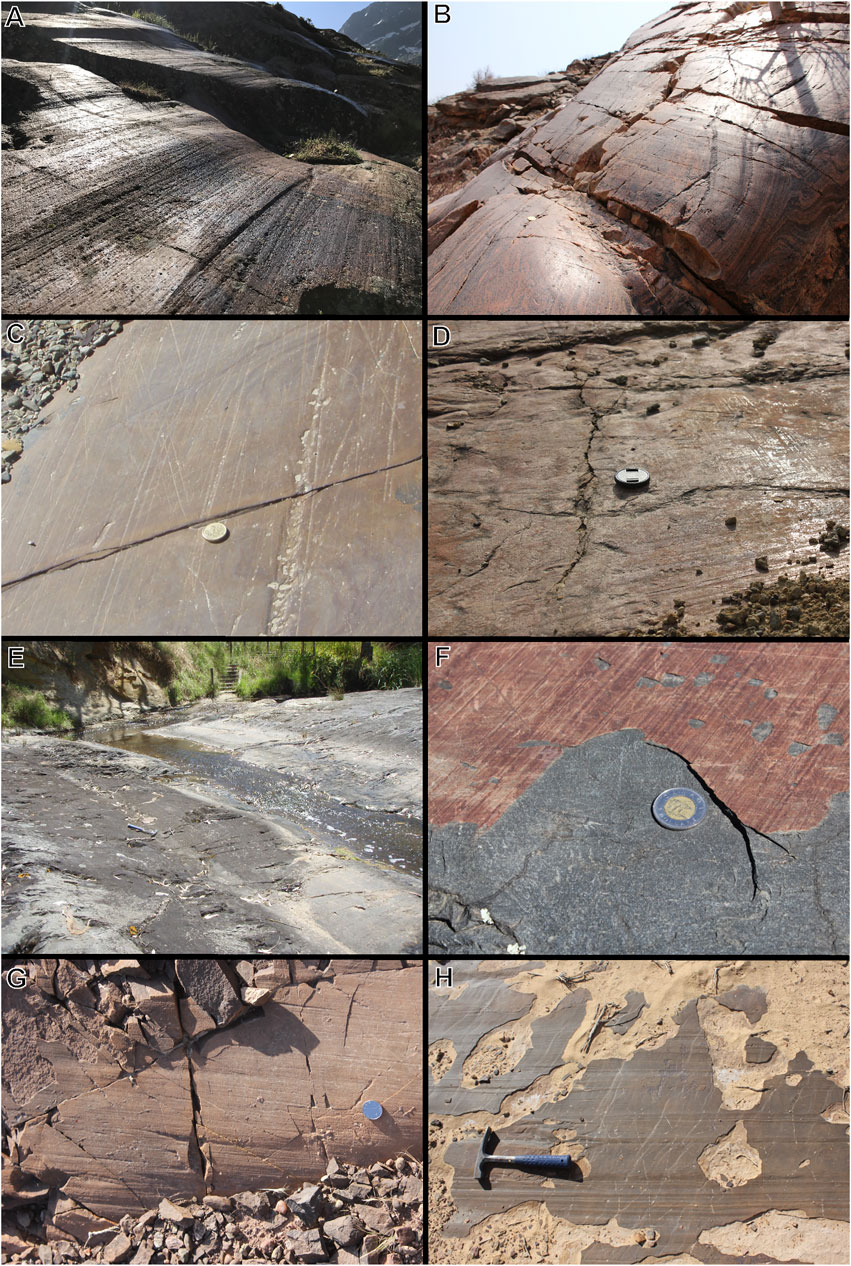
FIGURE 7. Striated surfaces through space and time. (A) Polished bedrock (paragneiss) exposed in recent decades following the retreat of the Gepatsch Glacier in Kaunertal, Austria. (B) Polished bedrock (orthogneiss) in northern Namibia, which was glaciated approximately 300 million years ago (Dietrich et al., in press). (C) Early Permian striated surface developed on Neoproterozoic siltstone at Hallett Cove, South Australia. Note crosscutting striations and chatter marks. (D) Surface sheen (glacial polish) at Pingdingshan, central China, reveals evidence for two contrasting flow directions beneath and Ediacaran ice sheet. (E) Interaction of a modern creek with an early Permian grooved and ridged surface at Glacier Rock, South Australia. (F) The peeling crust of glacial varnish, preserving striations, weathers away from paragneiss bedrock in Ontario, Canada. This surface is of Pleistocene age and is associated with MSGLs developed in bedrock (Krabbendam et al., 2016). (G) Probable late Cryogenian striated pavement in the Tarim Craton, NW China, beneath the Yuermeinak Formation (see Vandyk et al., 2019). (H) Example of a peeling and highly weathered soft-sediment striated surface (e.g., Le Heron et al., 2020) from the Late Ordovician of Saudi Arabia.
Following the work of Siman-Tov et al. (2017, 2021) on Quaternary surfaces in California and Washington, we propose that surface water weathering results in the selective loss of the silica gel precipitated subglacially and attributed to the surface sheen. Erosion of the mm-thick subglacial precipitate reveals a surface devoid of evidence of subglacial abrasion. In each of our “hard rock” examples from Cryogenian, Ediacaran, Late Carboniferous and Holocene glacial pavements, it is apparent that preserving fine detail is dependent on serendipity, and presumably highly localised weathering conditions that either favour preservation or destruction of the detail. In contrast, the LPIA surface of Oorlogskloof (Figure 7H) differs fundamentally because the striations record the shearing of soft sediment (Le Heron et al., 2020), rather than abrasion processes (Denis et al., 2010) which are most commonly envisaged beneath modern and Quaternary ice masses. Nevertheless, the increasing deployment of remotely operated vehicles on submerged, formerly glaciated continental shelves (Normandeau et al., 2021) may reveal similar structures in future research.
The role of abrasion was emphasised in the explanation of complex “nano-stratigraphy” observed on Quaternary glaciated surfaces of the United States (Siman-Tov et al., 2017). Siman-Tov et al. (2021) subsequently used detailed thin section imagery and chemical analysis to suggest that the surface sheen on striated surfaces is <1 μm thick. Where subglacial obstacles (i.e., protrusions from the bed up into the ice) are present, a laminated chemical deposit can occur in its lee. The phenomenon results from a paired dissolution-precipitation process, whereby “chemically aggressive water”, perhaps produced through stoss-side pressure melting, enriched the subglacial water film with solutes. Regelation in the lee of a bedrock obstacle then concentrates the solutes and instigates precipitation of either Si or Ca-rich layers, depending on the nature of the solute (Siman-Tov et al., 2021). Presently, it is unclear whether this model explains the preservation of crosscutting striations in ancient glacial varnish (Figures 7C,D). Nevertheless, viewing the layers of subglacial varnish as a kind of nano-stratigraphy, containing discrete stratigraphic events (e.g., separate ice advance cycles), may reveal interesting new insights into palaeo-ice sheet behaviour at the micro-to nano-scales. This phenomenon merits deeper investigation in the ancient record, and whether the nano-stratigraphy reflects separate freezing or regelation phases, for example.
Larger Scale (>1 m) Challenges
Comprehensive ice sheet reconstructions in the deep time record pose multiple challenges. The first of these challenges, corresponds to data gaps related to outcrop style. For the Cryogenian record, Busfield and Le Heron (2018) noted that “paleoland surfaces are concealed in successions of dipping strata, and are hence not amenable to satellite-image or aerial-photograph analysis”. Many of the famous and well-studied Cryogenian outcrops fall under this category, such as the Wilyerpa Formation of the Flinders Ranges (Busfield and Le Heron, 2014), the Chuos Formation of Namibia (Le Heron et al., 2013a; Busfield and Le Heron, 2013), or the Kingston Peak Formation of California, USA (Busfield and Le Heron, 2016; Le Heron et al., 2017). Most recently, Vandyk et al. (2021) reappraised the origins of a previously unchallenged striated pavement at the base of the Mineral Fork Formation, Utah, also in a suite of dipping strata. Using highly detailed photogrammetric methods, coupled with field observations, the striae were shown to be tectonic or recent erosional features, consistent with similar features formed within the surrounding modern landscape. In that study, the existence of a palaeotopography at the base of the presumed glaciogenic strata was demonstrated, but the origins of that topography was concluded to be equivocal, perhaps resulting from base-level drawdown rather than direct glacial incision.
The second of our large-scale challenges corresponds to dissolution of the geomorphic evidence. Around the world, many Cryogenian glacial sequences rest directly on carbonate platform stratigraphy (Spence et al., 2016). The solubility of carbonate bedrock has two major implications in terms of the geomorphic record: 1) the development of subglacial landforms is different and 2) post-glacial modification is to be expected, and thus the quality of any preserved subglacial landforms is poorer than those developed on metamorphic or igneous bedrock, for example. This is complicated by the fact that work on modern glacial erosional processes has focussed largely on, and has been biased toward, non-carbonate crystalline bedrock substrates and analysis of carbonate bedrock has been relatively restricted (Steinemann et al., 2020 and references therein). Nevertheless, Böhm (1885) described the results of erosion and karstification of Triassic Dachstein carbonate in Steiermark, Austria. North of the Gesäuse some corries occur, yet aside from some exotic erratic blocks such as schist, physical evidence for glaciation is poor even though excellent evidence for Quaternary glaciation is known from neighbouring, non-carbonate mountain ranges (Böhm, 1885).
Specific patterns of dissolution (cryokarst features) are also expected beneath glaciers where they advance over carbonate. In the LPIA record, carbonate substrates (where present) were not simply modified during deglaciation, but rather subglacial meltwater and erosion played an active role in karstification. For example, a wide range of karst structures are recognised in Devonian limestones of Western Australia, which are interpreted to have been produced by dissolution beneath mid Carboniferous to early Permian continental ice sheets (Playford, 2002). Thus, fundamental questions should be asked why subglacial karst is not preserved in the Cryogenian. The Snowball Earth hypothesis has continually had to reinvent itself as it is confronted with substantial sedimentological evidence that militates against it (Allen and Etienne, 2008). Yet, given the vast sea level drawdown that would be expected during “global” glaciation, the lack of karst is noteworthy.
Our third grand challenge, concerns substantial missing pieces of the jigsaw puzzle. Some of these appear to have been “misplaced”, whereas others will never be found. By comparison to the increasingly high-resolution ice sheet reconstructions in the Late Ordovician (Ghienne et al., 2007; Dietrich et al., 2018) or Late Carboniferous (e.g. Isbell and Cole, 2008; Griffis et al., 2021), Cryogenian and Ediacaran reconstructions are often of low spatial resolution but achievable in areas of locally high-quality data (e.g., Chen et al., 2020; Chen et al., 2020). This shifts the focus to the role of traditional outcrop sedimentology on to macro-scale (subglacial structures such as drumlins, whalebacks, or roches moutonnées) to micro-scale (petrographic or micromorphological: Busfield and Le Heron, 2018) facies analysis in such successions to tease out interpretations and to develop palaeo-glaciological models. In so doing, this highlights the intimate connection between palaeogeomorphology and sedimentology in the palaeo-glaciological record. The lowermost sediments in a formation, in contact with a subglacial surface, may have been deposited millions of years after the erosion of the surface. For example, in the Yuermeinak Formation of NW China. Vandyk et al. (2019) recognised two different facies immediately overlying a Cryogenian striated surface. One facies association comprised diamictites interbedded with dropstone-bearing rhythmites, tens of metres in thickness. The other was a distinctly different, thin diamictite facies. The former contained predominantly sub-angular to angular clasts. The latter contained rounded clasts with a high abundance of faceted, striated examples that were derived from the immediately underlying substrate. The former was interpreted as sediment gravity flows deposited an unknown time after erosion of the subglacial surface whereas the latter was a tillite, recording contemporaneous deposition during or shortly after subglacial erosion (Vandyk et al., 2019). Despite their glacigenic characteristics, both facies were not necessarily synchronous. Similar findings were obtained from strata pertaining to the lower Karoo (Late Paleozoic) in Botswana where non-dated massive breccias containing angular, m-scale locally-derived clasts, initially interpreted of glacial origin but recently reappraised as non-glacial, are abutting against a bedrock palaeotopography of probable glacial origin pertaining to the LPIA (Dietrich et al., 2019).
Even for extensive outcrop belts of Late Ordovician strata in the Sahara (e.g., Ghienne et al., 2003) or Arabia (Michael et al., 2018; Tofaif et al., 2019), preservation gaps remain on the order of hundreds of kilometres (Le Heron, 2016). This is presumed to be owing to subsequent uplift, denudation and erosion resulting from the genesis of large intracratonic basins such as the Murzuq Basin in Libya (Le Heron et al., 2013b; Ghienne et al., 2013). The “misplaced” pieces of the puzzle correspond to data preserved in the subsurface of sedimentary basins which have escaped erosion, and can be imaged on seismic reflection data. These include convincing evidence for tunnel valley networks at 3 km depth in the northern part of the Murzuq Basin, southern Libya (Smart, 2000), and to other features including ice contact deltas (Bataller et al., 2019).
The modern geographic and climatic setting in which outcrops are found also plays a role. Most of the examples given in this paper highlight the value of mapping in terrains characterised by arid to semi-arid climate. The absence of vegetation in these settings allows for more lucid interpretations. Nevertheless, the future is bright for the mapping of the deep time record in vegetated terrains. In many regions of the world, the rapid expansion of freely available (or at least low cost) LiDAR datasets has facilitated rapid advances in the mapping of Quaternary landforms (e.g., Thorndycraft et al., 2016). Allowing the interpreter to “see” the geomorphology concealed beneath dense vegetation, these data will likely have major application to the interpretation of the pre-Pleistocene record in coming years.
Conclusion
The mapping of ancient (Precambrian to Palaeozoic) glacial land surfaces has historical precedents, notably the work of Beuf et al. (1971) and Rognon et al. (1972) on the Late Ordovician geomorphology of the Sahara. This work was ahead of its time, and set the tone for the “big picture” approach to glacial geomorphology that is only now possible thanks to the widespread availability of free satellite imagery and affordable UAV technology;
Analysis of satellite imagery in formerly glaciated areas of both Cryogenian (Kimberley, WA, Australia) and Late Carboniferous (Ennedi, Chad) age allows important hypotheses to be tested concerning the flow patterns of deep-time ice sheets. In the former case, a suite of mega-scale streamlined subglacial structures is interpreted for the first time which are parallel to striations already observed in the field (Perry and Roberts, 1968). In the latter case, the morphology of channel systems described is used to argue for an ice-marginal or deglacial system;
Analyses of data collected by UAVs greatly advance our understanding of subglacial bed conditions in the Ediacaran, Late Ordovician and Late Carboniferous records. At Pingdingshan, central China (Ediacaran), the lateral distribution of p-forms enables us to understand the role of water in the generation and evolution of polished bedrock surfaces. At Oorlogskloof, South Africa (Late Carboniferous), the spatial organisation of subglacial bedforms into three discrete cross-cutting packages can only be accurately determined thanks to aerial imagery, attesting to complex coupling relationships between the ice and its bed. The latter two cases emphasise how soft glacier beds record stratigraphy, and how their mapping can play a central role in understanding subglacial conditions;
There is much potential in marrying traditional fieldwork with the bird’s eye view from satellite imagery and UAV photogrammetry. Nevertheless, grand challenges remain, including substantial data gaps both on the small scale (the fragile and fragmentary nature of many striated pavements) and the larger scale. In terms of the latter, dissolution may play a key role in Cryogenian records. In other cases, tectonic uplift and basin evolution explains the fragmentary nature of the record.
Data Availability Statement
The original contributions presented in the study are included in the article/Supplementary Material, further inquiries can be directed to the corresponding author.
Author Contributions
DPLH conceived the paper, wrote the draft, and designed the figures. MEB, MC, BJD, PD, J-FG, TMV, and RW edited and improved the text and contributed interpretations. XC developed the palaeovalley interpretation for Figure 2. LS provided technical support for UAV and satellite image manipulation and to the interpretations.
Funding
LHDP and DP acknowledge funding from the South Africa–Austria joint project of the National Research Foundation (NRF) of South Africa and the Österreichischer Austauschdienst (OEAD project ZA 08/2019).
Conflict of Interest
The authors declare that the research was conducted in the absence of any commercial or financial relationships that could be construed as a potential conflict of interest.
Publisher’s Note
All claims expressed in this article are solely those of the authors and do not necessarily represent those of their affiliated organizations, or those of the publisher, the editors and the reviewers. Any product that may be evaluated in this article, or claim that may be made by its manufacturer, is not guaranteed or endorsed by the publisher.
References
Allen, P. A., and Etienne, J. L. (2008). Sedimentary Challenge to Snowball Earth. Nat. Geosci. 1, 817–825. doi:10.1038/ngeo355
Andrews, G. D., McGrady, A. T., Brown, S. R., and Maynard, S. M. (2019). First Description of Subglacial Megalineations from the Late Paleozoic Ice Age in Southern Africa. PLOS ONE 14, e0210673. doi:10.1371/journal.pone.0210673
Aquino, C. D., Milana, J. P., and Faccini, U. F. (2014). New Glacial Evidence at the Talacasto Paleofjord (Paganzo Basin, W-Argentina) and its Implications for the Paleogeography of the Gondwana Margin. J. S. Am. Earth Sci. 56, 278–300. doi:10.1016/j.jsames.2014.09.001
Arnaud, E., and Etienne, J. L. (2011). Chapter 3 Recognition of Glacial Influence in Neoproterozoic Sedimentary Successions. Geol. Soc., 39–50. doi:10.1144/M36.3
Assine, M. L., de Santa Ana, H., Veroslavsky, G., and Vesely, F. F. (2018). Exhumed Subglacial Landscape in Uruguay: Erosional Landforms, Depositional Environments, and Paleo-Ice Flow in the Context of the Late Paleozoic Gondwanan Glaciation. Geol. Soc. Lond. Memoirs 36, 1–12. doi:10.1016/j.sedgeo.2018.03.011
Bataller, F. J., McDougall, N., and Moscariello, A. (2019). Ordovician Glacial Paleogeography: Integration of Seismic Spectral Decomposition, Well Sedimentological Data, and Glacial Modern Analogs in the Murzuq Basin, Libya. Interpretation 7, T383–T408. doi:10.1190/INT-2018-0069.1
Batchelor, C. L., Margold, M., Krapp, M., Murton, D. K., Dalton, A. S., Gibbard, P. L., et al. (2019). The Configuration of Northern Hemisphere Ice Sheets through the Quaternary. Nat. Commun. 10, 3713. doi:10.1038/s41467-019-11601-2
Beuf, S., Biju-Duval, B., de Charpal, O., Rognon, P., Gariel, O., and Bennacef, A. (1971). Les Grès du paléozoīque inférieur au Sahara: sédimentation et discontiunités, évolution structurale d'un craton. Paris: Editions Technip, 484p.
Busfield, M. E., and Le Heron, D. P. (2016). A Neoproterozoic Ice Advance Sequence, Sperry Wash, California. Sedimentology 63, 307–330. doi:10.1111/sed.12210
Busfield, M. E., and Le Heron, D. P. (2013). Glacitectonic Deformation in the Chuos Formation of Northern namibia: Implications for Neoproterozoic Ice Dynamics. Proc. Geologists' Assoc. 124, 778–789. doi:10.1016/j.pgeola.2012.10.005
Busfield, M. E., and Le Heron, D. P. (2014). Sequencing the Sturtian Icehouse: Dynamic Ice Behaviour in South Australia. J. Geol. Soc. 171, 443–456. doi:10.1144/jgs2013-067
Busfield, M. E., and Le Heron, D. P. (2018). Snowball Earth under the Microscope. J. Sediment. Res. 88, 659–677. doi:10.2110/jsr.2018.34
Bussert, R. (2010). Exhumed erosional landforms of the Late Palaeozoic glaciation in northern Ethiopia: Indicators of ice-flow direction, palaeolandscape and regional ice dynamics. Gondwana Research 18, 356–369. doi:10.2110/jsr.2018.34
Chandler, B. M. P., Lovell, H., Boston, C. M., Lukas, S., Barr, I. D., Benediktsson, Í. Ö., et al. (2018). Glacial Geomorphological Mapping: A Review of Approaches and Frameworks for Best Practice. Earth-Science Rev. 185, 806–846. doi:10.1016/j.earscirev.2018.07.015
Chen, X., Kuang, H., Liu, Y., Wang, Y., Yang, Z., Vandyk, T. M., et al. (2020). Subglacial Bedforms and Landscapes Formed by an Ice Sheet of Ediacaran-Cambrian Age in West Henan, North China. Precambrian Res. 344, 105727. doi:10.1016/j.precamres.2020.105727
Clark, C. D., Chiverrell, R. C., Fabel, D., Hindmarsh, R. C. A., Ó Cofaigh, C., and Scourse, J. D. (2021). Timing, Pace and Controls on Ice Sheet Retreat: an Introduction to the BRITICE‐CHRONO Transect Reconstructions of the British-Irish Ice Sheet. J. Quat. Sci. 36, 673–680. doi:10.1002/jqs.3326
Corkeron, M. (2007). ‘Cap Carbonates’ and Neoproterozoic Glacigenic Successions from the Kimberley Region, North-West Australia. Sedimentology 54, 871–903. doi:10.1111/j.1365-3091.2007.00864.x
Corkeron, M. (2011). Chapter 65 Neoproterozoic Glacial Deposits of the Kimberly Region and Northwestern Northern Territory, Australia. Geol. Soc. Lond. Memoirs 36, 659–672. doi:10.1144/M36.65
Corkeron, M. (2008). Deposition and Palaeogeography of a Glacigenic Neoproterozoic Succession in the East Kimberley, Australia. Sediment. Geol. 204, 61–82. doi:10.1016/j.sedgeo.2007.12.010
Corkeron, M. L., and George, A. D. (2001). Glacial Incursion on a Neoproterozoic Carbonate Platform in the Kimberley Region, Australia. Geol. Soc. Am. Bull. 113, 2. doi:10.1130/0016-7606(2001)113<1121:gioanc>2.0.co;2
Davies, B. J., Darvill, C. M., Lovell, H., Bendle, J. M., Dowdeswell, J. A., Fabel, D., et al. (2020). The Evolution of the Patagonian Ice Sheet from 35 Ka to the Present Day (PATICE). Earth-Science Rev. 204, 103152. doi:10.1016/j.earscirev.2020.103152
Denis, M., Guiraud, M., Konaté, M., and Buoncristiani, J.-F. (2010). Subglacial Deformation and Water-Pressure Cycles as a Key for Understanding Ice Stream Dynamics: Evidence from the Late Ordovician Succession of the Djado Basin (Niger). Int. J. Earth Sci. Geol. Rundsch) 99 (6), 1399–1425. doi:10.1007/s00531-009-0455-z
Deschamps, R., Eschard, R., and Roussé, S. (2013). Architecture of Late Ordovician Glacial Valleys in the Tassili N'Ajjer Area (Algeria). Sediment. Geol. 289, 124–147. doi:10.1016/j.sedgeo.2013.02.012
Dietrich, P., Franchi, F., Setlhabi, L., Prevec, R., and Bamford, M. (2019). The Nonglacial Diamictite of Toutswemogala Hill (Lower Karoo Supergroup, Central Botswana): Implications on the Extent of the Late Paleozoic Ice Age in the Kalahari-Karoo Basin. J. Sediment. Res. 89, 875–889. doi:10.2110/jsr.2019.48
Dietrich, P., Ghienne, J.-F., Lajeunesse, P., Normandeau, A., Deschamps, R., and Razin, P. (2018). “Deglacial Sequences and Glacio-Isostatic Adjustment: Quaternary Compared with Ordovician Glaciations,” in Glaciated Margins: The Sedimentary and Geophysical Archives. Editors D. P. Le Heron, K. A. Hogan, E. R. Phillips, M. Huuse, M. E. Busfield, and A. G. C. Graham (Bath: Geological Society, London, Special Publication), 475, 149–179. doi:10.1144/SP475.9
Dietrich, P., Griffis, N. P., Le Heron, D. P., Montañez, I. P., Kettler, C., Robin, C., et al. (2021). Fjord Network in Namibia: A Snapshot into the Dynamics of the Late Paleozoic Glaciation. Geology 49, 1521–1526. doi:10.1130/G49067.1
Dietrich, P., and Hofmann, A. (2019). I Ce‐margin Fluctuation Sequences and Grounding Zone Wedges: The Record of the Late Palaeozoic Ice Age in the Eastern Karoo Basin (Dwyka Group, South Africa). Depositional Rec. 5, 247–271. doi:10.1002/dep2.74
Dow, D. B. (1965). Evidence of a Late Pre-Cambrian glaciation in the Kimberley region of Western Australia. Geological Magazine 102, 407–414.
Dow, D. B., and Gemuts, I. (1969). Geology of the Kimberley Region, Western Australia. Bulletin: The East Kimberley 106 Bureau of Mineral Resources, Geology and Geophysics.
Dykstra, M., Kneller, B., and Milana, J. P. (2006). Deglacial and Postglacial Sedimentary Architecture in a Deeply Incised Paleovalley/paleofjord; the Late Carboniferous (Pennsylvanian) Jejenes Fm., San Juan, Argentina. Geol. Soc. Am. Bull. 118 (7), 913–937. doi:10.1130/b25810.1
Eyles, N. (2008). Glacio-epochs and the Supercontinent Cycle after ∼3.0 Ga: Tectonic Boundary Conditions for Glaciation. Palaeogeogr. Palaeoclimatol. Palaeoecol. 258, 89–129. doi:10.1016/j.palaeo.2007.09.021
Fedorchuk, N. D., Isbell, J. L., Griffis, N. P., Montañez, I. P., Vesely, F. F., Iannuzzi, R., et al. (2019). Origin of Paleovalleys on the Rio Grande Do Sul Shield (Brazil): Implications for the Extent of Late Paleozoic Glaciation in West-Central Gondwana. Palaeogeogr. Palaeoclimatol. Palaeoecol. 531, 108738. doi:10.1016/j.palaeo.2018.04.013
Gellatly, D. C., Derrick, G. M., and Plumb, K. A. (1975). The Geology of the Landsdowne 1:250000 Sheet Area. Western Australia: Australian Government Publishing Service.
Grey, K., and Corkeron, M. (1998). Late Neoproterozoic stromatolites in glacigenic successions of the Kimberley region. Western Australia: evidence for a younger Marinoan glaciation. Precambrian Research 92, 65–87.
Germs, G. J. B. (1972). “Chapter V: Glacial Phenomena,” in Bulletin 12: The Stratigraphy and Paleontology of the Lower Nama Group South West Africa. Precambrian Research Unit, Department of Geology, University of Cape Town, Cape Town, South Africa, Chamber of Mines, 97–107.
Ghienne, J.-F., Deynoux, M., Manatschal, G., and Rubino, J.-L. (2003). Palaeovalleys and Fault-Controlled Depocentres in the Late-Ordovician Glacial Record of the Murzuq Basin (Central Libya). Comptes Rendus Geosci. 335, 1091–1100. doi:10.1016/j.crte.2003.09.010
Ghienne, J.-F., Girard, F., Moreau, J., and Rubino, J.-L. (2010). Late Ordovician Climbing-Dune Cross-Stratification: A Signature of Outburst Floods in Proglacial Outwash Environments? Sedimentology 57, 1175–1198. doi:10.1111/j.1365-3091.2009.01142.x
Ghienne, J.-F., Le Heron, D. P., Moreau, J., Denis, M., and Deynoux, M. (2007). “The Late Ordovician Glacial Sedimentary System of the North Gondwana Platform,” in Hambrey, M.J. Glacial Sedimentary Processes and Products. Editors P. Christoffersen, N. F. Glasser, and B. Hubbard (Singapore: Blackwell Publishing Ltd.), 295–319. doi:10.1002/9781444304435.ch17
Ghienne, J.-F., Moreau, J., Degermann, L., and Rubino, J.-L. (2013). Lower Palaeozoic Unconformities in an Intracratonic Platform Setting: Glacial Erosion versus Tectonics in the Eastern Murzuq Basin (Southern Libya). Int. J. Earth Sci. Geol. Rundsch) 102, 455–482. doi:10.1007/s00531-012-0815-y
Girard, F., Ghienne, J.-F., and Rubino, J.-L. (2012). Channelized Sandstone Bodies ('cordons') in the Tassili N'Ajjer (Algeria & Libya): Snapshots of a Late Ordovician Proglacial Outwash Plain. Geol. Soc. Lond. Spec. Publ. 368, 355–379. doi:10.1144/SP368.3
Griffis, N., Montañez, I., Mundil, R., Heron, D. L., Dietrich, P., Kettler, C., et al. (2021). High-latitude Ice and Climate Control on Sediment Supply across SW Gondwana during the Late Carboniferous and Early Permian. GSA Bull. 133, 2113–2124. doi:10.1130/B35852.1
Griffis, N. P., Montañez, I. P., Mundil, R., Richey, J., Isbell, J., Fedorchuk, N., et al. (2019). Coupled Stratigraphic and U-Pb Zircon Age Constraints on the Late Paleozoic Icehouse-To-Greenhouse Turnover in South-Central Gondwana. Geology 47, 1146–1150. doi:10.1130/g46740.1
Guan, B. D., Wu, R. T., Hambrey, M. J., and Geng, W. C. (1986). Glacial Sediments and Erosional Pavements Near the Cambrian-Precambrian Boundary in Western Henan Province, China. J. Geol. Soc. 143, 311
Hall, A. M., Krabbendam, M., van Boeckel, M., Goodfellow, B. W., Hättestrand, C., Heyman, J., et al. (2020). Glacial Ripping: Geomorphological Evidence from Sweden for a New Process of Glacial Erosion. Geogr. Ann. Ser. A, Phys. Geogr. 102, 333–353. doi:10.1080/04353676.2020.1774244
Hambrey, M. J., and Harland, W. B. (1981). Earth’s Pre-pleistocene Glacial Record. Cambridge: Cambridge University Press.
Hughes, A. L. C., Gyllencreutz, R., Lohne, Ø. S., Mangerud, J., and Svendsen, J. I. (2016). The Last Eurasian Ice Sheets - a Chronological Database and Time‐slice Reconstruction, DATED‐1. Boreas 45, 1–45. doi:10.1111/bor.12142
Isbell, J. L., Cole, D. I., and Catuneanu, O. (2008). Carboniferous-Permian Glaciation in the Main Karoo Basin, South Africa: Stratigraphy, Depositional Controls, and Glacial Dynamics. Special Pap. Geol. Soc. Am. 441, 71–82. doi:10.1130/2008.2441(05)
Kennedy, K., Eyles, N., and Broughton, D. (2018). Basinal Setting and Origin of Thick (1·8 Km) Mass-Flow Dominated Grand Conglomérat Diamictites, Kamoa, Democratic Republic of Congo: Resolving Climate and Tectonic Controls during Neoproterozoic Glaciations. Sedimentology 66, 556–589. doi:10.1111/sed.12494
Kennedy, K., and Eyles, N. (2020). Syn‐rift Mass Flow Generated 'tectonofacies' and 'tectonosequences' of the Kingston Peak Formation, Death Valley, California, and Their Bearing on Supposed Neoproterozoic Panglacial Climates. Sedimentology 68, 352–381. doi:10.1111/sed.12781
Kettler, C., Wohlschlägl, R., Russell, C., Ghienne, J.-F., and Le Heron, D. P. (in review). A World-Class Example of a Late Palaeozoic Glaciated Landscape in Chad. J. Geol. Soc. Lond.
Kneller, B., Pablo Milana, J., Buckee, C., and al Jaʼaidi, O. (2004). A Depositional Record of Deglaciation in a Paleofjord (Late Carboniferous [Pennsylvanian] of San Juan Province, Argentina): The Role of Catastrophic Sedimentation. Geo. Soc. Am. Bull. 116, 348–367. doi:10.1130/b25242.1
Kor, P. S. G., Shaw, J., and Sharpe, D. R. (1991). Erosion of bedrock by subglacial meltwater, Georgian Bay, Ontario: A regional view. Canadian Journal of Earth Sciences 28, 623–642. doi:10.1139/e91054
Krabbendam, M., Eyles, N., Putkinen, N., Bradwell, T., and Arbelaez-Moreno, L. (2016). Streamlined Hard Beds Formed by Palaeo-Ice Streams: A Review. Sediment. Geol. 338, 24–50. doi:10.1016/j.sedgeo.2015.12.007
Kurjanski, B., Rea, B. R., Spagnolo, M., Winsborrow, M., Cornwell, D. G., Andreassen, K., et al. (2019). Morphological Evidence for Marine Ice Stream Shutdown, Central Barents Sea. Mar. Geol. 414, 64–76. doi:10.1016/j.margeo.2019.05.001
Le Heron, D. P. (2018). An Exhumed Paleozoic Glacial Landscape in Chad. Geology 46, 91–94. doi:10.1130/G39510.1
Le Heron, D. P., Busfield, M. E., and Kamona, F. (2013a). An Interglacial on Snowball Earth? Dynamic Ice Behaviour Revealed in the Chuos Formation, Namibia. Sedimentology 60, 411–427. doi:10.1111/j.1365-3091.2012.01346.x
Le Heron, D. P., and Craig, J. (2008). First-order Reconstructions of a Late Ordovician Saharan Ice Sheet. J. Geol. Soc. 165, 19–29. doi:10.1144/0016-76492007-002
Le Heron, D. P., Craig, J., Sutcliffe, O. E., and Whittington, R. (2006). Late Ordovician Glaciogenic Reservoir Heterogeneity: An Example from the Murzuq Basin, Libya. Mar. Petroleum Geol. 23, 655–677. doi:10.1016/j.marpetgeo.2006.05.006
Le Heron, D. P., Dietrich, P., Busfield, M. E., Kettler, C., Bermanschläger, S., and Grasemann, B. (2019b). Scratching the Surface: Footprint of a Late Carboniferous Ice Sheet. Geology 47, 1034–1038. doi:10.1130/G46590.1
Le Heron, D. P., Heninger, M., Baal, C., and Bestmann, M. (2020). Sediment Deformation and Production beneath Soft-Bedded Palaeozoic Ice Sheets. Sediment. Geol. 408, 105761. doi:10.1016/j.sedgeo.2020.105761
Le Heron, D. P., Meinhold, G., and Bergig, K. A. (2013b). Neoproterozoic-Devonian Stratigraphic Evolution of the Eastern Murzuq Basin, Libya: A Tale of Tilting in the Central Sahara. Basin Res. 25, 52–73. doi:10.1111/j.1365-2117.2012.00555.x
Le Heron, D. P. (2016). The Hirnantian Glacial Landsystem of the Sahara: a Meltwater-Dominated System. Geol. Soc. Lond. Memoirs 46, 509–516. doi:10.1144/M46.151
Le Heron, D. P., Tofaif, S., Vandyk, T., and Ali, D. O. (2017). A Diamictite Dichotomy: Glacial Conveyor Belts and Olistostromes in the Neoproterozoic of Death Valley, California, USA. Geology 45, 31–34. doi:10.1130/G38460.1
Le Heron, D. P., Vandyk, T. M., Kuang, H., Liu, Y., Chen, X., Wang, Y., et al. (2019a). Bird's-eye View of an Ediacaran Subglacial Landscape. Geology 47, 705–709. doi:10.1130/G46285.1
Lopez-Gamundi, O., and Buatois, L. A. (2010). “Introduction: Late Paleozoic Glacial Events and Postglacial Transgressions in Gondwana,” in Late Paleozoic Glacial Events and Postglacial Transgressions in Gondwana. Editors O. Lopez-Gamundi,, and L. A. Buatois (Geological Society of America Special Paper), 468. doi:10.1130/2010.2468(0010.1130/2010.2468(00)
Margold, M., Stokes, C. R., and Clark, C. D. (2015). Ice Streams in the Laurentide Ice Sheet: Identification, Characteristics and Comparison to Modern Ice Sheets. Earth-Science Rev. 143, 117–146. doi:10.1016/j.earscirev.2015.01.011
Martin, H., and Schalk, K. (1959). Gletscherschliffe an der Wand eines U-Tales im nördlichen Kaokofeld, Südwestafrika. Geol. Rundsch 46, 571–575. doi:10.1007/bf01803042
Martin, H. (1981). “The Late Paleozoic Dwyka Group of the South Kalahari Basin in Namibia and Botswana and the subglacial valleys of the Kaokoveld in Namibia,” in Earth’s Pre-Pleistocene Glacial Record. Editors M. J. Hambrey, and W. B. Harland. Cambridge: Cambridge University Press, 61–66.
Michael, N. A., ZÜhlke, R., and Hayton, S. (2018). The Palaeo-Valley Infilling Glaciogenic Sarah Formation, an Example from Rahal Dhab Palaeo-Valley, Saudi Arabia. Sedimentology 65, 851–876. doi:10.1111/sed.12408
Möller, P., and Dowling, T. P. F. (2016). Streamlined Subglacial Bedforms on the Närke Plain, South-Central Sweden - Areal Distribution, Morphometrics, Internal Architecture and Formation. Quat. Sci. Rev. 146, 182–215. doi:10.1016/j.quascirev.2016.04.007
Moreau, J., Ghienne, J.-F., Heron, D. P. L., Rubino, J.-L., and Deynoux, M. (2005). 440 Ma Ice Stream in North Africa. Geology 33, 753–756. doi:10.1130/G21782.1
Moreau, J. (2011). The Late Ordovician Deglaciation Sequence of the SW Murzuq Basin (Libya). Basin Res. 23, 449–477. doi:10.1111/j.1365-2117.2010.00499.x
Nascimento, D. B., Ribeiro, A., Trouw, R. A. J., Schmitt, R. S., and Passchier, C. W. (2016). Stratigraphy of the Neoproterozoic Damara Sequence in Northwest Namibia: Slope to Basin Sub-marine Mass-Transport Deposits and Olistolith Fields. Precambrian Res. 278, 108–125. doi:10.1016/j.precamres.2016.03.005
Normandeau, A., MacKillop, K., Maquarrie, M., Richards, C., Bourgault, D., Campbell, D. C., et al. (2021). Submarine landslides triggered by iceberg collision with the seafloor. Nature Geoscience 14, 599–605.
Perry, W. J., and Roberts, H. G. (1968). Late Precambrian Glaciated Pavements in the Kimberley Region, Western Australia. J. Geol. Soc. Aust. 15, 51–56. doi:10.1080/00167616808728679
Playford, P. E. (2002). “Palaeokarst, Pseudokarst, and Sequence Stratigraphy in Devonian Reef Complexes of the Canning Basin, Western Australia,” in The Sedimentary Basins of Western Australia, 3. Editors M. Keep,, and S. J. Moss (Symposium, Perth, W: Petroleum Exploration Society of Australia).
Rognon, P., Biju-Duval, B., and de Charpal, O. (1972). Modelés glaciaires dans l'Ordovicien supérieur saharien: Phases d'érosion et glacio-tectonique sur la bordure N des Eglab. Revue de Géogr. Physique de Géologie Dynamique 14, 507
Siman-Tov, S., Blackburn, T., Hallet, B., Coble, M. A., and Brodsky, E. E. (2021). Siliceous Subglacial Deposits: Archives of Subglacial Processes during the Last Glacial Maximum. J. Glaciol. 67, 977–984. doi:10.1017/jog.2021.42
Siman-Tov, S., Stock, G. M., Brodsky, E. E., and White, J. C. (2017). The Coating Layer of Glacial Polish. Geology 45, 987–990. doi:10.1130/G39281.1
Śledź, S., Ewertowski, M. W., and Piekarczyk, J. (2021). Applications of Unmanned Aerial Vehicle (UAV) Surveys and Structure from Motion Photogrammetry in Glacial and Periglacial Geomorphology. Geomorphology 378, 107620. doi:10.1016/j.geomorph.2021.107620
Smart, J. (2000). “Seismic Expressions of Depositional Processes in the Upper Ordovician Succession of the Murzuq Basin, SW Libya,” in Geological Exploration of the Murzuq Basin. Editors M. A. Sola,, and D. Worsley (Amsterdam: Elsevier, 397–415. doi:10.1016/b978-044450611-5/50021-0
Spence, G. H., Le Heron, D. P., and Fairchild, I. J. (2016). Sedimentological Perspectives on Climatic, Atmospheric and Environmental Change in the Neoproterozoic Era. Sedimentology 63, 253–306. doi:10.1111/sed.12261
Steinemann, O., Ivy‐Ochs, S., Grazioli, S., Luetscher, M., Fischer, U. H., Vockenhuber, C., et al. (2020). Quantifying Glacial Erosion on a Limestone Bed and the Relevance for Landscape Development in the Alps. Earth Surf. Process. Landforms 45, 1401–1417. doi:10.1002/esp.4812
Tedesco, J., Cagliari, J., Coitinhodos, J. d. R. R., da Cunha Lopes, R., and Lavina, E. L. C. (2016). Late Paleozoic Paleofjord in the Southernmost Parana Basin (Brazil): Geomorphology and Sedimentary Fill. Geomorphology 269, 203–214. doi:10.1016/j.geomorph.2016.06.035
Thorndycraft, V. R., Cripps, J. E., and Eades, G. L. (2016). Digital Landscapes of Deglaciation: Identifying Late Quaternary Glacial Lake Outburst Floods Using LiDAR. Earth Surf. Process. Landforms 41, 291–307. doi:10.1002/esp.3780
Tofaif, S., Le Heron, D. P., and Melvin, J. (2019). Development of a Palaeovalley Complex on a Late Ordovician Glaciated Margin in NW Saudi Arabia. Geol. Soc. Lond. Spec. Publ. 475, 81–107. doi:10.1144/SP475.8
Valdez Buso, V., Di Pasquo, M., Milana, J. P., Kneller, B., Fallgatter, C., Junior, F. C., et al. (2017). Integrated U-Pb Zircon and Palynological/palaeofloristic Age Determinations of a Bashkirian Palaeofjord Fill, Quebrada Grande (Western Argentina). J. S. Am. Earth Sci. 73, 202–222. doi:10.1016/j.jsames.2016.12.009
Valdez Buso, V., Milana, J. P., Di Pasquo, M., and Espinoza Aburto, J. (2021). The Glacial Paleovalley of Vichigasta: Paleogeomorphological and Sedimentological Evidence for a Large Continental Ice-Sheet for the Mid-carboniferous over Central Argentina. J. S. Am. Earth Sci. 106, 103066. doi:10.1016/j.jsames.2020.103066
Vandyk, T. M., Kettler, C., Davies, B. J., Shields, G. A., Candy, I., and Le Heron, D. P. (2021). Reassessing Classic Evidence for Warm-Based Cryogenian Ice on the Western Laurentian Margin: The "striated Pavement" of the Mineral Fork Formation, USA. Precambrian Res., 36. 106345. doi:10.1016/j.precamres.2021.106345
Vandyk, T. M., Wu, G., Davies, B. J., Xiao, Y., Li, M., Shields, G. A., et al. (2019). Temperate Glaciation on a Snowball Earth: Glaciological and Palaeogeographic Insights from the Cryogenian Yuermeinak Formation of NW China. Precambrian Res. 331. doi:10.1016/j.precamres.2019.105362/
Visser, J. (1997). Deglaciation Sequences in the Permo-Carboniferous Karoo and Kalahari Basins of Southern Africa: a Tool in the Analysis of Cyclic Glaciomarine Basin Fills. Sedimentology 44, 507–521. doi:10.1046/j.1365-3091.1997.d01-35.x
Visser, J. J. (1990). Glacial Bedforms at the Base of the Permo-Carboniferous Dwyka Formation along the Western Margin of the Karoo Basin, South Africa. Sedimentology 37, 231–245. doi:10.1111/j.1365-3091.1990.tb00957.x
Keywords: glaciation, geomorphology, sedimentology, diamictite, striated pavement
Citation: Le Heron DP, Busfield ME, Chen X, Corkeron M, Davies BJ, Dietrich P, Ghienne J-F, Kettler C, Scharfenberg L, Vandyk TM and Wohlschlägl R (2022) New Perspectives on Glacial Geomorphology in Earth’s Deep Time Record. Front. Earth Sci. 10:870359. doi: 10.3389/feart.2022.870359
Received: 06 February 2022; Accepted: 25 April 2022;
Published: 18 May 2022.
Edited by:
John Menzies, Brock University, CanadaReviewed by:
Victoria Valdez Buso, Federal University of Paraná, BrazilRobert Bussert, Technical University of Berlin, Germany
Copyright © 2022 Le Heron, Busfield, Chen, Corkeron, Davies, Dietrich, Ghienne, Kettler, Scharfenberg, Vandyk and Wohlschlägl. This is an open-access article distributed under the terms of the Creative Commons Attribution License (CC BY). The use, distribution or reproduction in other forums is permitted, provided the original author(s) and the copyright owner(s) are credited and that the original publication in this journal is cited, in accordance with accepted academic practice. No use, distribution or reproduction is permitted which does not comply with these terms.
*Correspondence: D. P. Le Heron, ZGFuaWVsLmxlLWhlcm9uQHVuaXZpZS5hYy5hdA==