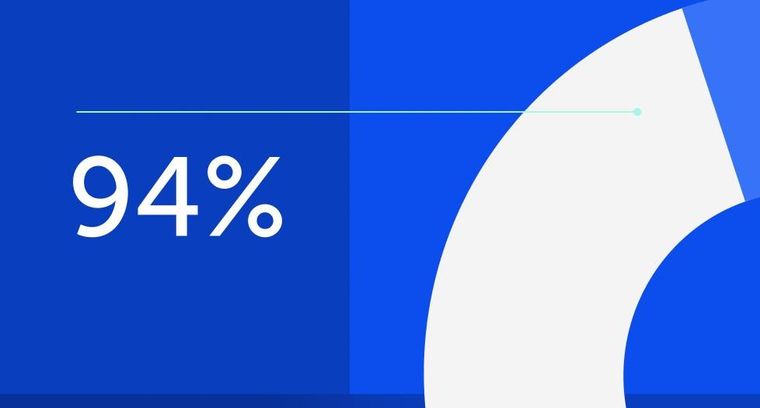
94% of researchers rate our articles as excellent or good
Learn more about the work of our research integrity team to safeguard the quality of each article we publish.
Find out more
ORIGINAL RESEARCH article
Front. Earth Sci., 23 May 2022
Sec. Structural Geology and Tectonics
Volume 10 - 2022 | https://doi.org/10.3389/feart.2022.864548
This article is part of the Research TopicFluid and Heat Transfer in Unconventional ReservoirsView all 15 articles
Thermophysical properties of rock and soil are important parameters that affect the efficiency of shallow geothermal energy utilization. This paper analyzes the thermophysical parameters (specific heat capacity and thermal conductivity) and their influencing factors based on 6467 sample data in 24 provincial capitals, China. The statistical distributions of thermophysical properties are analyzed based on histogram plots. Linear regression analysis is conducted to investigate the correlation between thermophysical properties and lithology (classified as metamorphic rocks, igneous rocks, chemical sedimentary rocks, clastic sedimentary rocks, and loose sediments in this study)), density, and water content. The results show that the thermophysical properties are influenced predominantly by lithology. The measured values of thermophysical properties generally show normal distribution characteristics. The specific heat capacity of loose sediments is relatively high, the thermal conductivity of other four types are relatively high. The specific heat capacity is negatively correlated with density, positively correlated with water content, whereas thermal conductivity is positively correlated with density, and negatively correlated with water content. The findings obtained in this study provide evidence and guidelines for the investigation, evaluation, and development of shallow geothermal resources.
Shallow geothermal energy is renewable and clean energy, which is characterized by being environmentally friendly, economical and applicable, widely distributed, convenient to use, and has great potential (Wang et al., 2012; Ran et al., 2014). For decades, the utilization of shallow geothermal energy has developed rapidly with the innovation of ground source heat pump technology. Since the United States established the first ground source heat pump system in the 1940s, universities in China began to study heat pump technology in the 1960s. Since the 1970s, the demonstration projects of shallow geothermal energy have been successively established in Switzerland, Netherlands and other countries. In the 1990s, the application of ground source heat pump systems was gradually progressed in China. At the beginning of the 21st century, ground source heat pump technology has developed rapidly. The annual growth rate of ground-source heat pump market in China exceeds 30% since 2004, which is much higher than the world’s average growth rate of 20–22% in the same period. It has now ranked second in the world (Zheng et al., 2010). According to the WGC 2020, geothermal (ground-source) heat pumps have the largest geothermal use worldwide, accounting for 71.6% of the installed capacity and 59.2% of the annual energy use. The number of countries with installations is 54, and over twice the number of units reported in 2010. The leaders in installed units (MWt) are China, United States, Sweden, Germany and Finland accounting for 77.4% of these units. Wang et al. (2017) conducted a new evaluation of the potential of shallow geothermal energy resources in China and the results showed that the shallow geothermal energy resources in 336 important cities could be extracted in an amount equivalent to 700 million t of standard coal per year. According to the survey, the actual heating and cooling area by shallow geothermal energy reached 1.6 billion m3 in 2020 (Lund and Toth, 2020).
The capability of storing and conducting heat of the rock and soil depends on their thermal conductivity, specific heat capacity, and thermal diffusivity, which are collectively referred to as thermophysical parameters. Clauser and Huenges (1995) studied the thermal conductivity characteristics of different rocks. Their results showed that porosity is an important factor affecting the thermal conductivity of igneous and sedimentary rocks. Thermophysical parameters are also a key factor affecting the engineering design of ground source heat pumps (Lin et al., 2012). Many studies have carried out related topics (e.g., Middleton, 1994; Hartmann et al., 2008; Fuchs and Förster, 2014; Cheng and Yu, 2017; McDaniel et al., 2018; Naranjo-Mendoza et al., 2018; Ohta et al., 2018; Song et al., 2018; Miranda et al., 2021; Zeng et al., 2022). Midttømme et al. (1998) studied the thermal conductivity and its influencing factors of claystone and mudstone. The results showed that there is a correlation between grain size and thermal conductivity. Goto and Matsubayashi (2009) investigated the relation of the thermophysical properties to the porosity and mineral composition of clay and sandy sediments in the Juan de Fuca Ridge and built models that fitted with the observed data. Popov et al. (2011) analyzed the thermophysical property measurements on cores from the scientific drilling well Yaxcopoil-1 in Mexico and established correlations between the thermal conductivity and elastic wave velocities measured in the laboratory. Jorand et al. (2015) analyzed statistically the petrophysical properties of rocks of the northeastern Rhenish Massif and the Lower Rhine Embayment in Germany and found that the mineralogical compositions and water-filled pore volume control the thermophysical properties of rocks. Song et al. (2019) studied the characteristics and influencing factors of thermophysical properties of different rocks in the main strata in Guizhou Province, China. They found that the thermophysical properties of rocks are related to factors such as mineral composition, structure, and water content.
Such contributions are greatly helpful to understanding the characteristics of thermophysical parameters of rock and soil. However, the rock samples used in the related analysis are relatively small distributed in limited areas. Furthermore, the thermophysical parameters in most studies focus on thermal conductivity. Currently, few studies have focused on the distribution of thermophysical properties and their influencing factors nationwide in China. This study collected 6467 data from the survey and evaluation of shallow geothermal energy in 24 provincial capitals in China. The rock heat capacity and thermal conductivity are analyzed statistically. Their influencing factors are also analyzed and their correlation with rock type, rock density, and water content is investigate. The results provide a basis for the investigation, evaluation, development, and utilization of shallow geothermal energy.
In 2014–2019, the shallow geothermal energy exploration and evaluation work had been completed in 31 provincial capital cities in China including Beijing, Tianjin, and Shanghai. To investigate the overall distribution characteristics of rock thermophysical parameters and their influencing factors in provincial capital cities, the sample data are checked and filtered, which are distributed in 24 provincial capital cities in China. It is mainly collected in strata of Carboniferous, Permian, Jurassic, Neogene, and Quaternary. The sampling depth is basically within 200 m. There are a total of 6467 samples. The number of rock samples for different cities is listed in Table 1.
The thermophysical properties are tested in the laboratory. The method of testing is followed by the Geotechnical Test Method Standard, GB_T50123-1999, China (GB/T 50123-1999; Ministry of Construction of the People’s Republic of China, 1999). Samples were obtained from the different formations using a borehole sampler. After collecting rock and soil samples from the boreholes and sending them to the laboratory, and use special testing equipment to conduct thermophysical properties of rocks are tested by specific equipment and the testing results can thus be obtained directly. The microscopic characteristics of soil sample grains are observed by the optical stereo microscope method (magnification 30 -100). The rock density is measured by the volume ring gauge method (electronic balance 0.01 g, volume ring gauge, etc.). The specific gravity of rocks is measured by the standard pycnometer method plus vacuum pumping. The water content is evaluated by the standard drying method (electronic balance 0.1 mg, standard drying oven) 105°C, etc.). The thermal conductivity, specific heat, thermal diffusivity are measured by the unsteady heat transfer method, in line with ASTM D5334-00 standard.
The thermophysical properties of rock and soil are related to the origin and geological time when they formed, and the geological environment where they are located. These aspects are generally intuitively reflected in their lithology, mineral composition, texture, structure, density, porosity, water content, saturation, pressure, temperature, degree of weathering, etc (Kim et al., 2002; Hartmann et al., 2005; Muhieddine et al., 2012; Jorand et al., 2015; Oktay et al., 2015; Fuchs, 2018; Pimienta et al., 2018). Therefore, the thermophysical characteristics can be further investigated regarding these related factors.
The classification and the number of samples are shown in Table 2. The loose sediments and clastic sedimentary rocks accounted for most of samples, roughly 83% of the total number of samples. A small portion of residual slope soil and fill soil is classified as silt. Nearly 92% of the metamorphic rocks are slate and gneiss. Limestone accounts for a higher proportion of chemical sedimentary rocks, nearly 88%. For igneous rocks, granite accounts for the highest proportion of 69%.
The histograms of thermophysical parameters, including thermal conductivity and specific heat of rock and soil for various rock types are shown in Figure 1, showing a relatively centered distribution in general. The specific heat capacity for loose sediments is higher than those of metamorphic and igneous rocks. Metamorphic rocks, chemical sedimentary rocks, and igneous rocks have a higher thermal conductivity than clastic sedimentary rocks and loose sediments. As can be seen from Table 2, the values of the thermophysical parameters of these groups of rocks are strongly influenced by those of the prevalent lithotype, such as of the slate and gneiss among the metamorphic, rocks, sandstone and mudstone among the clastic sedimentary rocks, sandstone and mudstone among the clastic sedimentary rocks, limestone among the chemical sedimentary rocks, granite among the igneous rocks, clay and sand among the loose sediments. (Figure 1).
FIGURE 1. Histograms of heat capacity (A) and thermal conductivity (B) of different rock and soil mass samples. (The y axis displays the percentage given by the samples number within the specified interval over the total number of the rock and soil samples.).
Lithology is a general concept, which is a comprehensive manifestation of rock’s mineral composition, texture, physical and chemical properties. Rock lithology is comprehensively affected by their minerals, and the thermophysical parameters of different minerals vary (Wang et al., 1979; Han et al., 2017; Tiwari et al., 2021). The physical properties vary with the rock and soil types. For samples from 24 provincial capitals in China, based on the basic type of metamorphic rocks, sedimentary rocks, igneous rocks, and loose sediments, samples are further classified according to their lithology (Table 2). Further statistical analysis of the thermophysical parameters for different rocks types is conducted as shown in Figure 2, which gives clues for the influences of rock lithology on their thermophysical parameters. The median specific heat capacity of different types ranges roughly from 0.5 to 1.5 KJ/(kg•K), and the median thermal conductivity is from 1 to 4 W/(m•K) (Figures 2A,B). In this study, most selected measure data are the average values for the specified rock type in a certain study area, the anisotropic effects are diminished in data processing. The object of this study is to investigate the relative magnificent of heat conductivity and capacity of different type of rocks and their influence factors. Hence, the anisotropic effect are omitted.
FIGURE 2. Boxplots of heat capacity (A) and thermal conductivity (B) of rock and soil samples, having different lithology. (Boxes represent the quartile, red lines the median, whiskers–maximum data range, black circles the outliers.).
Rocks, including igneous rocks, sedimentary rocks, and metamorphic rocks, have a higher thermal conductivity than loose sediments being characterized by lower porosity (Figure 2B). For metamorphic rocks, the thermal conductivity for phyllite is highest, with a median value of 3.9 W/(m•K), while it is the lowest for skarn, with a median value of 1.1 W/(m•K). The variation of rock thermal conductivity is small for slate, with a median value of 2.39 W/(m•K). The median thermal conductivity of sedimentary rocks ranges from 1.8 to 2.8 W/(m•K). The chemical sedimentary rocks (limestone and dolomite) have a higher thermal conductivity than clastic sedimentary rocks (sandstone, mudstone, etc.). Sandstone and mudstone are the most widely distributed rock samples for clastic sedimentary rocks. The median thermal conductivity of sandstone is 1.86 W/(m•K) and mudstone is 1.79 W/(m•K). For igneous rocks, the rock thermal conductivity for granite is highest of 2.4 W/(m•K), the gabbro is lowest of 1.8 W/(m•K). The rock thermal conductivity for andesite has a larger variation range of 2–3 W/(m•K) than other igneous rocks. The median thermal conductivity of loose sediments is 1–2.1 W/(m•K), in which gravel is higher, sand and silt are in the middle, mud and clay are lower, shell layer is the lowest, and the thermal conductivity of gravel has a larger variation. The thermal conductivity of loose sediments generally shows an increasing trend with grain size, which result is consistent with the result of Midttømme et al. (1998).
The variations of specific heat capacity with rock types are opposite to those of thermal conductivity, looser sediments have higher specific heat capacities and rocks, including igneous rocks, sedimentary rocks, and metamorphic rocks (Figure 2A). Clay, mud, and silt have the highest specific heat capacity, which is about 1.35KJ/(kg•K). Sand, pebbles, and shells have a relatively low specific heat capacity of about 1.18 KJ/(kg•K). The median value of clastic sedimentary rocks is relatively stable, ranging from 0.8 to 1.1 KJ/(kg•K), and the specific heat capacity of mudstone is relatively low. However, there are certain abnormal values in sandstone and mudstone, with the lowest of 0.2 KJ/(kg•K) and the highest of 3.5 KJ/(kg•K).
For igneous rocks, gabbro’s specific heat capacity is the lowest of 0.46KJ/(kg•K), and the granite’s specific heat capacity varied greatly from 0.7 to 1.2 KJ/(kg•K), followed by the diorite ranges from 0.5 to 0.7 KJ/(kg•K). The specific heat capacity of limestone is higher than that of dolomite, about 1 KJ/(kg•K), and the variation range of dolomite is relatively larger (has a bigger box in the plot) than that of limestone with 0.35–0.75 KJ/(kg•K). The specific heat capacity of metamorphic rocks has a large variation ranging from 0.3 to 1.4 KJ/(kg•K), which is the lowest for skarn, and the highest for gneiss. The specific heat capacity of phyllite varies greatly from 0.7 to 1.35 KJ/(kg•K).
In general the finer the particles of the loose sediment, the greater the porosity. Most samples are collected below the hydrostatic surface, so it can be assumed saturated with water whose thermal conductivity is lower and specific heat capacity is higher than most minerals. The comparisons of thermophysical parameters for different rocks show that the denser the rock-soil structure, the greater the thermal conductivity; the looser, the smaller the thermal conductivity, which indicating the combined effects of porosity and water content. The specific heat capacity is opposite, as it is generally larger for Quaternary loose layer than that for bedrock, and it is generally larger for clay than that for sandy soil and pebble layer.
The specific heat capacity of each soil body in the Quaternary loose overburden is quite different. Because the clay has a high porosity and water content, its specific heat capacity is relatively higher than that of the gravel and pebbles. It shows that silt and clay have a great ability to absorb or release heat. The thermal conductivity of pebble and sandy soil is better than that of silt and clay (Figure 2). These characteristics indicate that the heat absorption effect of the rock is better than that of the loose sediments, and the soil layer has a better heat storage capability than the rock layer due to the larger water content. Coarse-grained rock and soil have a better heat-releasing effect, but poor heat storage capability. On the contrary, fine-grained sediment has a better heat storage effect, but a poor heat-releasing effect. From the perspective of the development and utilization of shallow geothermal energy resources, the rock and soil are required to have good heat release performance for improving the efficiency of conducting heat.
Density is the basic physical property of rock and soil, which are composed of solid, liquid, and gas phases with a decreasing trend in density. Experience data shows that among the three-phase composition, the thermal conductivity of solid is the largest, followed by that of liquid, and that of gas is the smallest. Histograms of density for different rocks types are shown in Figure 3, column A. The density of metamorphic rocks, chemical sedimentary rocks, and igneous rocks are relatively higher than those of clastic sedimentary rocks and loose sediments. Whereas few samples of metamorphic rocks, chemical sedimentary rocks, and igneous rocks (row 1, 3, and 4) have low measured densities due to weathering. As a large number of rock samples for clastic sedimentary rocks and loose sediments, they show highly centered distributions.
FIGURE 3. Histograms of density (A) and its regression analysis with respect to heat capacity (B) and thermal conductivity (C) of rock and soil samples.
By correlating the thermophysical properties, thermal conductivity and specific heat capacity, and density of all rock types, it shows that the thermal conductivity is positively related to rock density (black dashed lines in Figure 3, Column 3) but the specific heat capacity is negative to rock density (black dashed lines in Figure 3, Column 2). These results are in line with the previous studies (e.g., Hartmann et al., 2005; Oktay et al., 2015). The rock type is further classified according to its lithology. The regression analysis was conducted and the fitted parameters are shown in Table 3. The correlation between thermophysical properties and density for each rock type keep similar (colored dashed lines in Figure 3, Columns 2 and 3). For a given type of rock and soil, the thermal conductivity is positively correlated with the density. It is inversely proportional to the density (Figure 3, colored dashed line).
For metamorphic rocks, the specific heat capacity and thermal conductivity of slate vary slightly with density (a small slope), and the variation of gneiss is relatively large (a big slope). The changes of thermophysical properties with density are relatively similar for clastic sedimentary rocks. Compared with the specific heat capacity, the thermal conductivity has a strong correlation with density (a relatively high R2). The density of chemical sedimentary rocks concentrates between 2.6 and 2.8 g/m3, and the weathered rocks with low density may slightly change the slopes. For igneous rocks, the specific heat of diorite varied obviously with density (the slope and R2 are large), and the thermal conductivity of granite changes apparently with density. Similar to clastic sedimentary rocks, the thermophysical parameters of different loose sediments varied similarly with the density changes. The thermophysical parameters of clay (including specific heat and thermal conductivity) show a strong correlation with density.
For a specific rock type, higher density usually implies lower porosity if their mineralogy are identical, hence their thermal conductivity has an increasing trend with the density. Since the thermal conductivity is closely related to the grain configurations, the closely compacted mineral grains i.e., low porosity, increase the capability of conducting heat and thus a high thermal conductivity. The main effect of density on the difference in thermophysical properties lies in the “degree of compactness”. For a given saturated soil, if the mineral composition and texture are basically the same, the difference in density results from the difference in the structure, porosity, water content, and other factors. Accordingly, the varied density may reflect the characteristics of porosity and water content to some extent and thus result in a change in thermophysical properties.
The water content is calculated as the percentage of the mass of free water in the total mass of the rock and soil (Li et al., 2007; Jorand et al., 2011; Kim and Oh, 2019). The water content of metamorphic rocks, chemical sedimentary rocks, and igneous rocks are small in general, less than 10% overall, and mostly concentrated between 0 and 5%, except for a few weathered rocks with high water content. The water content of clastic sedimentary rocks ranges roughly from 0 to 30%. As the water content increases, the number of samples gradually decreases. The water content of loose rocks concentrates around 20% and shows a nearly normal distribution (Figure 4).
FIGURE 4. Histograms of water content (A) and its regression analysis with respect to heat capacity (B) and thermal conductivity (C) of rock and soil samples.
For metamorphic rocks, the correlation between thermophysical parameters and water content for slate is not obvious compared to gneiss, which is similar to the previous results. For clastic sedimentary rocks, compared to sandstone, thermophysical parameters have a strong correlation with water content (a high R2 in Table 4), and there are many mudstone samples with low specific heat capacity and low thermal conductivity. The thermophysical parameters of chemical sedimentary rocks have relatively low correlations with water content and exhibit a small change (small slopes as listed in Table 4).
Analysis of all the rock sample data shows that the specific heat capacity is directly proportional to water content (Figure 4 and Table 4), which is consistent with the results of Kim and Oh (2019). For igneous rocks, the water content greatly influences the thermal conductivity of granite and specific heat capacity of diorite. For loose sediments, the thermal conductivity and specific heat capacity of clay are greatly influenced by water content, while slit and sand are relatively unaffected by water content. As the particle size of the different sediments decreases, their thermal conductivity decreases and their thermal energy storage capacity increases.
The specific heat capacity mainly depends on the content of water and air. Since the specific heat capacity of water is much larger than that of air, the greater the water content in the rock and soil, the greater its specific heat capacity and vice versa. The data above indicates that specific heat capacity is positively correlated with water content, consistent with other studies.
In addition, some studies show that thermal conductivity is directly proportional to water content (Kim and Oh, 2019). Nevertheless, the analysis in this study shows that the thermal conductivity is negatively correlated with the water content (Figure 4; Table 4). The main reason may lie in that the relationship between thermophysical parameters and water content is not a simple linear relationship, and their correlation is also restricted by other factors such as lithology, density, etc. For example, considering silt and clay with large porosity, their density tends to be small, and yield a high water content. Their small contact area between the soil skeleton, and thus more pore water involved in transferring the heat, which results in a small thermal conductivity and a large specific heat capacity. Since the soils have different degree of cementation, the effect of porosity on the thermal properties is more complex, which is reflected in the different statistical patterns of these properties of sand and bedrock.
In terms of spatial distribution, except for structural factors, the distribution of thermophysical parameters could also be influenced by geological formation conditions and hydrogeological conditions. Tectonic faults affect heat transfer for crystalline rocks, due to the complexity and lack of commonality of these mechanisms, this aspect is not discussed in this paper.
Because the thermal conductivity of rocks is generally greater than that of loose sediments, the thicker and the larger the underlying bedrock, the higher thermal conductivity and the lower the specific heat capacity for the area. On the contrary, if the greater the thickness and area of loose sediments, e.g., silt and clay, the lower the thermal conductivity and the higher the specific heat capacity.
From the viewpoint of geomorphology, the high thermal conductivity area tends to be distributed in the mountains and the area where the bedrock is shallowly buried. By contrast, the thermal conductivity of the plain area is generally lower than that of the mountainous area (This part will be discussed further in the next section). Furthermore, the granite, dolomite, limestone, and shale areas in the bedrock area are also larger than the sandstone and mudstone areas. In the plain area, the thermal conductivity is slightly higher in river terraces and alluvial fans (where the lithology of strata is mainly coarse sand and gravel sand) than in the wind and slope deposition areas (where the lithology of strata is mainly clay and silty clay). The distribution characteristics of high and low specific heat capacity are roughly opposite to that of the thermal conductivity.
Hydrogeological conditions and temperature region are closely related to the thermophysical parameters of rock and soil. Different temperature regions have different climates and different weathering processes in the surface layer, affecting parameters such as lithology, grain size and water content of the loose sediments, influencing the thermophysical parameters in turn. The distribution of thermal conductivity of samples collected in most cities is correlated with the hydraulic characteristics. For instance, the high thermal conductivity area in Hohhot distributes in the pre-mountain plain area near the mountain, where is in well hydraulic conditions with coarse grains in strata; the thermal conductivity is low in the Daheihe plain area where is in poor hydraulic conditions with fine grains in strata. For the same rock formation in the urban area of Jinan, the better the groundwater runoff conditions, the stronger the water richness, and the greater the thermal conductivity. The high-value thermal conductivity is distributed in the south of Haikou City where is the groundwater recharge area, and the low-value is in the northern coastal plain, where is the groundwater discharge area. The distribution of specific heat capacity is opposite to that of the thermal conductivity. This reflects the influence of hydrogeological conditions on thermophysical parameters. Accordingly, the main factor that affects the thermophysical parameters is the hydrodynamic conditions. Areas with good hydrodynamic conditions generally have the characteristics of large water volume and rapid flow rate, which has a positive effect on heat transfer, whereas, in areas with poor hydrodynamic conditions, the groundwater moves slow and thermal transfer tend to small.
Data from the clay and sand of Harbin, Taiyuan and Fuzhou, from the cold temperate, mesothermal and subtropical zones, are listed in Table 5. There are small differences in water content and density regularity of clay, without meaningful trend. The thermal conductivity of the samples elevate with increase of regional temperature and the specific heat capacity shows a roughly decreasing trend. For sand in areas with high regional temperatures, the samples had higher water content, density and thermal conductivity and lower specific heat capacity. Overall, among samples with small differences in water content and density, the thermal conductivity of the rock/soil is positively correlated with the regional temperature and precipitation, while the specific heat capacity is the opposite.
The thermal conductivity of clay in the Huang-Huai-hai Plain is small, but the median value tends to be the same in other regions, except for very large and very small values, which were mainly caused by the change of water content. The mean thermal conductivity of clay is 1.48 W/mK. In sand and clay, the thermal conductivity reflects regional differences. It is lower in the Songliao Plain, higher in the hilly mountains of eastern and central China, and the mean values in the Huang- Huai-hai Plain and the Loess Plateau of Inner Mongolia and Shaanxi-Gansu are relatively close to each other, with the median mean values ranging from 1.60 to 2.00 W/mK from the clay to the sands. The variability of regional thermal conductivity in bedrock is more evident, with distributions between 1.46 and 3.00 W/mK. The density, porosity and permeability of rocks in different regions, the nature and content of fluids in the pores of rocks, as well as the differences in temperature and pressure, all have an effect on the thermal conductivity (Table 6).
TABLE 6. Statistical results of thermal and physical parameters of each lithology in different regions.
The regional variation of specific heat capacity is not clear. In the clay, slit, sand and shale in Quaternary, Neoproterozoic and Paleoproterozoic strata, the specific heat capacity in the karst hills of southwest China is generally lower than that in other areas of the earth, and that in the hills of east and central China is slightly higher than that in other areas. The median values range from 0.88 to 1.48 kJ/kgK. Among the rocks, the median specific heat capacity is concentrated around 1.00 kJ/kgK. The specific heat capacity of mudstone in the Songliao Plain is high, with a median value of 1.30 kJ/kgK, and the specific heat capacity of shale in southeast and south China marine climate is higher, with a median value of 1.27 kJ/kgK.
The thermal conductivity and heat capacity of the clastic sedimentary rock in plains, plateaus, mountains and hills are approximately similar. About the mudstone, the lowest thermal conductivity is found in the loess plateau, maybe due to the low water content. The thermal conductivity of sandstone in Tropical coastal plain area is 1.7 times of those in Songliao Plain, while the specific heat capacity is only 1/2. Similar features are found of the conglomerate, the formation of sedimentary strata containing smaller particles is more favorable in the Songliao Plain than in the coastal areas.
For the chemical sedimentary rock, the characteristics of the thermal and physical parameters tend to be consistent and do not correlate significantly with their locations.
For the igneous rocks, data are only available in Huang-Huai-Hai Plain, East China - Central China Hilly Mountainous Region and Tropical coastal plain area. The thermal conductivity of the granite in Tropical coastal plain area is 3.14, the highest value among those of the region.
Loose sediment is very heterogeneous, including clay, silt and sand widely distribute in seven different areas. For clay, only the thermal conductivity and specific heat capacity of Northwest Interior Basin are significantly lower than those of other areas, while the other six areas are basically the same. For silt, the thermal conductivity and specific heat capacity of Southwest karst hills are significantly lower than those of East China–Central China Hilly Mountainous Region and Tropical coastal plain area, and the thermal conductivity of sand shows a trend of gradually decreasing from mountain–plateau–plain (Figure 5).
FIGURE 5. Relationship between the thermal and physical parameters of each lithology in different regions.
Generally speaking, from the hilly areas to the middle of the basin or plain, as the altitude decreases, the lithology gradually changes from sand, pebbles and gravels to silt and clay interlayers. The lithology change is accompanied by a gradual decrease of the thermal conductivity and increase of heat capacity, according to the gradual grain size decrease and porosity increase of the rock/soil’s.
In this study, the thermophysical properties of 6467 rock and soil samples collected from 24 provincial capitals in China are analyzed and correlated with the lithology, density, water content. The thermal conductivity of the bedrock is generally greater than that of the Quaternary loose sediments. Dolomite, shale, and granite have a higher thermal conductivity than gabbro, sandstone, and mudstone for the bedrock. For the loose sediments, the thermal conductivity of pebble and gravel is larger than that of the clay and silt, which shows a decreasing trend with the grain sizes. For the specific heat capacity, which is opposite to the thermal conductivity, it is generally lower in the bedrock than that in the Quaternary loose sediments. Density and water content affect thermophysical parameters, thermal conductivity increases with density and decrease with water content, while specific heat capacity decreases with density and increase with water content.
To summarize, during the development and utilization of shallow geothermal energy, it is necessary to consider the local conditions such as geological formation, hydrogeological settings to representatively collect measurement samples to determine thermophysical properties, in order to assess the applicable resource amount, optimize ground source heat pumps systems to maximize the utilization of shallow geothermal energy. The finding of this study provides data basis and variation analysis for the resource assessments and modeling, provides insights for the site selections of shallow geothermal energy development and utilization.
The raw data supporting the conclusions of this article will be made available by the authors, without undue reservation.
XZ Collected and collated the data listed in the manuscript and completed the main sections of the manuscript, ZG Provided methods of data analysis, TC analysed data and produced graphs, WW collected and collated primary data, CL provided methods for data analysis, QZ reviewed the literature and collated the current state of research.
This study was financially supported by the Natural Science Foundation of Hebei Province, China (Grant No. D2021504041), The National Key Research and Development Program of China (Grant No. 2019YFB1504101), Geological Survey Project (DD20221676).
The authors declare that the research was conducted in the absence of any commercial or financial relationships that could be construed as a potential conflict of interest.
All claims expressed in this article are solely those of the authors and do not necessarily represent those of their affiliated organizations, or those of the publisher, the editors and the reviewers. Any product that may be evaluated in this article, or claim that may be made by its manufacturer, is not guaranteed or endorsed by the publisher.
The authors acknowledge the reviewer for carefully reading and giving critical comments on this manuscript.
Cheng, C., and Yu, W. (2017). Research Progress and Development Tendency about Thermal Physical Properties of Rocks. Adv. Earth Sci. 32 (10), 1072–1083. (in Chinese with English abstract). doi:10.11867/j.issn.1001-8166.2017.10.1072
Clauser, C., and Huenges, E. (1995). “Thermal Conductivity of Rocks and Minerals,” in Rock Physics and Phase Relations: A Handbook of Physical Constants. Editor T.-J Ahrens (Hannover, Germany: American Geophysical Union), 3, 105–126.
Fuchs, S., and Förster, A. (2014). Well-log Based Prediction of Thermal Conductivity of Sedimentary Successions: A Case Study from the North German Basin. Geophys. J. Int. 196 (1), 291–311. doi:10.1093/gji/ggt382
Fuchs, S. (2018). The Variability of Rock Thermal Properties in Sedimentary Basins and the Impact on Temperature Modelling - A Danish Example. Geothermics 76, 1–14. doi:10.1016/j.geothermics.2018.06.006
Goto, S., and Matsubayashi, O. (2009). Relations between the Thermal Properties and Porosity of Sediments in the Eastern Flank of the Juan de Fuca Ridge. Earth Planet Sp. 61 (7), 863–870. doi:10.1186/bf03353197
Han, J., Sun, Q., Xing, H., Zhang, Y., and Sun, H. (2017). Experimental Study on Thermophysical Properties of Clay after High Temperature. Appl. Therm. Eng. 111, 847–854. doi:10.1016/j.applthermaleng.2016.09.168
Hartmann, A., Pechnig, R., and Clauser, C. (2008). Petrophysical Analysis of Regional-Scale Thermal Properties for Improved Simulations of Geothermal Installations and Basin-Scale Heat and Fluid Flow. Int. J. Earth Sci. (Geol Rundsch) 97 (2), 421–433. doi:10.1007/s00531-007-0283-y
Hartmann, A., Rath, V., and Clauser, C. (2005). Thermal Conductivity from Core and Well Log Data. Int. J. Rock Mech. Min. Sci. 42 (7-8), 1042–1055. doi:10.1016/j.ijrmms.2005.05.015
Jorand, R., Clauser, C., Marquart, G., and Pechnig, R. (2015). Statistically Reliable Petrophysical Properties of Potential Reservoir Rocks for Geothermal Energy Use and Their Relation to Lithostratigraphy and Rock Composition: The NE Rhenish Massif and the Lower Rhine Embayment (Germany). Geothermics 53, 413–428. doi:10.1016/j.geothermics.2014.08.008
Jorand, R., Fehr, A., Koch, A., and Clauser, C. (2011). Study of the Variation of Thermal Conductivity with Water Saturation Using Nuclear Magnetic Resonance. J. Geophys. Res. 116 (B8), 1–12. doi:10.1029/2010jb007734
Kim, D., and Oh, S. (2019). Relationship between the Thermal Properties and Degree of Saturation of Cementitious Grouts Used in Vertical Borehole Heat Exchangers. Energy Build. 201, 1–9. doi:10.1016/j.enbuild.2019.07.017
Kim, S., Kim, M. C., and Kim, K. Y. (2002). An Integral Approach to the Inverse Estimation of Temperature-Dependent Thermal Conductivity without Internal Measurements. Int. Commun. Heat Mass Transf. 29 (1), 107–113. doi:10.1016/s0735-1933(01)00329-3
Li, H. X., Xia, Z. Q., and Ma, G. H. (2007). Effects of Water Content Variation on Soil Temperature Process and Water Exchange. J. Hohai Univ. (Natural Sciences) 35 (2), 172–175. (in Chinese with English abstract). doi:10.3321/j.issn:1000-1980.2007.02.012
Lin, W. J., Wu, Q. H., and Wang, G. L. (2012). Shallow Geothermal Energy Resource Potential Evaluation and Environmental Effect in China. J. Arid Land Resour. Environ. 26 (3), 57–61. (in Chinese with English abstract).
Lund, J. W., and Toth, A. (2020). “Direct Utilation of Geothermal Energy 2020 Wordwide - 27 April, 2020,” in World Geothermal Congress 2020, Reykjavik, Iceland, 27 April, 2020.
McDaniel, A., Tinjum, J., Hart, D. J., Lin, Y.-F., Stumpf, A., and Thomas, L. (2018). Distributed Thermal Response Test to Analyze Thermal Properties in Heterogeneous Lithology. Geothermics 76, 116–124. doi:10.1016/j.geothermics.2018.07.003
Middleton, M. F. (1994). Determination of Matrix Thermal Conductivity from Dry Drill Cuttings. AAPG Bull. 78 (11), 1790–1799. doi:10.1306/a25ff297-171b-11d7-8645000102c1865d
Midttømme, K., Roaldset, E., and Aagaard, P. (1998). Thermal Conductivity Claystones and Mudstones of Selected from England. Clay Min. 33 (1), 131–145.
Ministry of Construction of the People’s Republic of China (1999). GB/T 50123-1999 Standard for Soil Test Methods. Beijing: Chinese Plan Publishing House.
Miranda, M. M., Velez Márquez, M. I., Raymond, J., and Dezayes, C. (2021). A Numerical Approach to Infer Terrestrial Heat Flux from Shallow Temperature Profiles in Remote Northern Regions. Geothermics 93, 102064. doi:10.1016/j.geothermics.2021.102064
Muhieddine, M., Canot, É., and March, R. (2012). Heat Transfer Modeling in Saturated Porous Media and Identification of the Thermophysical Properties of the Soil by Inverse Problem. Appl. Numer. Math. 62 (9), 1026–1040. doi:10.1016/j.apnum.2012.02.008
Naranjo-Mendoza, C., Wright, A. J., Oyinlola, M. A., and Greenough, R. M. (2018). A Comparison of Analytical and Numerical Model Predictions of Shallow Soil Temperature Variation with Experimental Measurements. Geothermics 76, 38–49. doi:10.1016/j.geothermics.2018.06.003
Ohta, K., Nishihara, Y., Sato, Y., Hirose, K., Yagi, T., Kawaguchi, S. I., et al. (2018). An Experimental Examination of Thermal Conductivity Anisotropy in Hcp Iron. Front. Earth Sci. 6, 176. doi:10.3389/feart.2018.00176
Oktay, H., Yumrutaş, R., and Akpolat, A. (2015). Mechanical and Thermophysical Properties of Lightweight Aggregate Concretes. Constr. Build. Mater. 96, 217–225. doi:10.1016/j.conbuildmat.2015.08.015
Pimienta, L., Klitzsch, N., and Clauser, C. (2018). Comparison of Thermal and Elastic Properties of Sandstones: Experiments and Theoretical Insights. Geothermics 76, 60–73. doi:10.1016/j.geothermics.2018.06.005
Popov, Y., Romushkevich, R., Korobkov, D., Mayr, S., Bayuk, I., Burkhardt, H., et al. (2011). Thermal Properties of Rocks of the Borehole Yaxcopoil-1 (Impact Crater Chicxulub, Mexico). Geophys. J. Int. 184 (2), 729–745. doi:10.1111/j.1365-246x.2010.04839.x
Ran, W. Y., Li, N. B., Yang, J. W., and Yu, Y. (2014). Some Enlightenment Gained from City Shallow Geothermal Energy Investigation and Evaluation. Urban Geol. 9 (Z1), 1–3. (in Chinese with English abstract). doi:10.3969/j.issn.0001-5717.2019.08.018
Song, X. Q., Jiang, M., Peng, Q., and Xiong, P. W. (2019). Thermal Property Parameters and Influencing Factor Analysis of Main Rock Strata in Guizhou Province. Atca Geol. Sin. 93 (8), 2092–2103. (in Chinese with English abstract).
Song, X., Zheng, R., Li, G., Shi, Y., Wang, G., and Li, J. (2018). Heat Extraction Performance of a Downhole Coaxial Heat Exchanger Geothermal System by Considering Fluid Flow in the Reservoir. Geothermics 76, 190–200. doi:10.1016/j.geothermics.2018.07.012
Tiwari, R., Boháč, V., Dieška, P., and Götzl, G. (2021). The Non-planar Surface of Carbonate Rock Sample Affecting the Behaviour of Thermal Response and the Measurement of Thermophysical Parameters by Pulse Transient Technique. Therm. Sci. Eng. Prog. 24, 100927. doi:10.1016/j.tsep.2021.100927
Wang, G. L., Lin, W. J., and Zhang, W. (2012). Evaluation on Utilization Potential of Shallow Geothermal Energy in Major Cities of China. Build. Sci. 28 (10), 1–3, 8 (in Chinese with English abstract). doi:10.12029/gc20170602
Wang, W. L., Wang, G. L., Zhu, X., and Liu, Z. M. (2017). Characteristics and Potential of Shallow Geothermal Resources in Provincial Capital Cities of China. Geol. China 44 (6), 1062–1073. (in Chinese with English abstract).
Wang, Y., Rajeshwar, K., Nottenburg, R. N., and Dubow, J. B. (1979). Thermophysical Properties of Oil Shale Minerals. Thermochim. Acta 30 (1-2), 141–151. doi:10.1016/0040-6031(79)85049-2
Zeng, Y., Ji, B., Zhang, Y., Feng, J., Luo, J., and Wang, M. (2022). A Fractal Model for Effective Thermal Conductivity in Complex Geothermal Media. Front. Earth Sci. 10, 786290. doi:10.3389/feart.2022.786290
Keywords: thermophysical properties, shallow geothermal energy, influencing factors, density, water content
Citation: Zhu X, Gao Z, Chen T, Wang W, Lu C and Zhang Q (2022) Study on the Thermophysical Properties and Influencing Factors of Regional Surface Shallow Rock and Soil in China. Front. Earth Sci. 10:864548. doi: 10.3389/feart.2022.864548
Received: 28 January 2022; Accepted: 02 May 2022;
Published: 23 May 2022.
Edited by:
Dawei Hu, Institute of Rock and Soil Mechanics (CAS), ChinaReviewed by:
Magdala Tesauro, Utrecht University, NetherlandsCopyright © 2022 Zhu, Gao, Chen, Wang, Lu and Zhang. This is an open-access article distributed under the terms of the Creative Commons Attribution License (CC BY). The use, distribution or reproduction in other forums is permitted, provided the original author(s) and the copyright owner(s) are credited and that the original publication in this journal is cited, in accordance with accepted academic practice. No use, distribution or reproduction is permitted which does not comply with these terms.
*Correspondence: Xi Zhu, engxOTg2MDcyN0AxNjMuY29t; Zongjun Gao, Z2Fvem9uZ2p1bkAxMjYuY29t
Disclaimer: All claims expressed in this article are solely those of the authors and do not necessarily represent those of their affiliated organizations, or those of the publisher, the editors and the reviewers. Any product that may be evaluated in this article or claim that may be made by its manufacturer is not guaranteed or endorsed by the publisher.
Research integrity at Frontiers
Learn more about the work of our research integrity team to safeguard the quality of each article we publish.