- 1State Key Laboratory of Earthquake Dynamics, Institute of Geology, China Earthquake Administration, Beijing, China
- 2Key Laboratory of High-temperature and High-pressure Study of the Earth’s Interior, Institute of Geochemistry Chinese Academy of Sciences, Guiyang, China
It has been demonstrated that the matters in the earth’s interior are subjected to isotropic hydrostatic pressure and are also extensively superimposed by the differential stress. The differential stress contributes significantly to the free energy of matters and it is the determining factor controlling the composition, structure, configuration, properties, and interaction processes of the matter system. Hence, the differential stress is one of the most fundamental thermodynamic variables governing the earth’s interior system along with the temperature and the hydrostatic pressure. Nevertheless, due to the limitations of high-temperature and high-pressure (HT-HP) setup and in situ measurement techniques as well as limited understanding of the differential stress, previous HT-HP experiments of the earth’s interior didn’t cover the role of the differential stress except for some special stress-strain mechanics experiments and piezolysis and kinetic metamorphism experiments. This makes many of the knowledge about the earth’s interior obtained from HT-HP experiments generally questionable. Currently, HT-HP experimental apparatus that can be used to simulate the temperature, hydrostatic pressure, and differential stress in the earth’s interior includes the Griggs press, the Paterson rheometer, the D-DIA press, the RDA press, and the torsional diamond anvil cell. The maximum hydrostatic pressure that can be simulated in the Griggs press at high temperatures is only about 2 GPa and there is large uncertainty in the calibration of the differential stress. The Paterson rheometer provides too low confining pressure. The D-DIA press and RDA press can simulate a wide range of temperature and pressure but the D-DIA press can achieve very small strain variables and the RDA press has very heterogeneous sample stresses. The torsional diamond anvil cell can only accept a small sample size and it is difficult to calibrate the differential stress. Also, these existing HT-HP experimental apparatus with the differential stress are not easily interfaced with in situ measurement systems for investigating the physical properties such as electrical, ultrasonic, and thermophysical properties. Hence, scholars need to invest more efforts in the research and development of HT-HP apparatus with the differential stress in the future to properly understand the composition, structure, configuration, properties, and interactions of the matter in the earth’s interior.
Introduction
The composition, structure, constructions, properties, interaction processes, and formation and evolution law of the earth’s interior are the most important research contents in the study on the earth’s inner working mechanism. Field observations such as geological, geochemical, geophysical, costeaning, probing, and drilling as well as laboratory analysis tests, theoretical calculations, and HT-HP experiments are the main tools used today to explore the inner working mechanism of the earth. However, the conclusions obtained from field observations and laboratory analysis tests require HT-HP experiments for validation. Also, the inversion of field geophysical data and theoretical computational simulations require HT-HP experiments to provide various trait parameters for the earth’s interior. The information on the earth’s interior obtained by field surveys and laboratory analysis tests are relatively limited and theoretical computational simulations are usually unable to investigate the non-equilibrium thermodynamic system and the naturally complex HT-HP matter system in the earth’s interior. In contrast, HT-HP experiments can reveal new phenomena and patterns that are difficult to reveal by field surveys, laboratory analysis tests, and theoretical computational simulations. Hence, HT-HP experiments of the earth’s interior play an important role in the exploration of the earth’s inner working mechanism (Karato, 2008, 2013).
In the stress analysis, the stress component also changes as the orientation of the stress unit body changes. There is an orientation of the stress unit body in which the shear stress component on the unit body surface is zero meaning that only the principal stress acts in the orthogonal section. When the stress values in the three principal stress directions are different, the object is subjected to the differential stress. Earth’s magnetic field, mantle convection, mantle column, plate subduction, magma intrusion, volcanoes, earthquakes, faults, folding, dynamic metamorphism, and other geological phenomena all indicate that the earth’s interior are subject to isotropic lithostatic pressure (equivalent to hydrostatic or quasi-hydrostatic pressure) or lithostatic pressure + pore/fracture fluid pressure, and there is also extensive superimposed differential stress on it (Kawamoto and Shimamoto, 1997; Niemeijer and Spiers, 2006; Imber et al., 2008; Toy et al., 2011; Rowe and Griffith, 2015). The differential stress acting on the earth’s interior system, whether it produces elastic or plastic deformation, significantly contributes to the free energy of the matter. The differential stress is the decisive factor controlling the phase composition, structure, construction, properties, and interaction processes of the material system. Hence, the differential stress, like the temperature and hydrostatic pressure, is one of the most fundamental thermodynamic variables governing the earth’s interior system. Nevertheless, previous HT-HP experiments of the earth’s interior didn’t cover the role of the differential stress except for some special stress-strain mechanics experiments and a few piezolysis and kinetic metamorphism experiments. As a result, much of what has been learned about the earth’s interior from HT-HP experiments to date is generally questionable. Hence, a proper understanding of the composition, structure, construction, properties, and interactions of the earth’s interior requires knowledge of the physicochemical properties of the earth’s interior under high temperatures, high pressures, or differential stresses.
In this study, the progress and problems in the study on the physical properties of the earth’s interior under the differential stress are briefly reviewed. Also, the existing HT-HP apparatus that can generate the differential stress are reviewed and some future research trends in this field are presented.
Literature Review
The effects of the differential stress on the phase, structure, and properties of condensed matter are well known in many fields such as condensed matter physics, materials science, and microelectronics. For instance, Ma, et al. found using their invented torsional diamond anvil cell type high-pressure device that the addition of torsional stress can greatly reduce the pressure of the phase transition of the metallic iron from α to ε (Ma and Emre, 2006; Benjamin et al., 2012; Daniel et al., 2014). The mechanism of the cold working hardening of metallic materials is the process associated with the cold working of materials to generate point defects and dislocations, to form internal stress in the crystal lattice, and eventually to induce the hardening of materials. The effects of the external differential stress and the internal stress on the phonon spectra of condensed matter and the peak positions and peak widths of X-ray diffraction spectra have long been used to measure the stress state of various materials especially for thin films and semiconductor devices (Siakavellas et al., 1997; Cao et al., 2006). The differential stress has a significant effect on the structural phase transition and physical properties of semiconductor materials (Grave et al., 2014; Budnitzki and Kuna, 2016), ferroelectric materials (Li et al., 2015; Zhu, 2014; Xu et al., 2016; Seyidov, 2016), superhard materials (Korotaev et al., 2016), and nonlinear optical materials (Mylvaganam et al., 2015). The differential stress significantly modulates the band structure of semiconductor materials and the magnetic properties and magnetic structure phase transition of ferromagnetic materials (Xu et al., 2008; Li et al., 2014; Coe et al., 2012).
In the field of HT-HP experimental studies on the composition, structure, construction, properties, and interactions of the earth’s interior, the effect of the differential stress as a fundamental thermodynamic variable on the experimental results have not been taken into account due to the limited HT-HP experimental apparatus or in situ measurement techniques for simulating high-temperature and complex stress conditions. The only exceptions are some specialized stress-strain mechanics experiments as well as piezolysis and dynamic metamorphism experiments (Tullis and Yund, 1991; Demouchy and Mainprice, 2011; Anil et al., 2012; Niwa et al., 2012; Ken et al., 2012; Liu et al., 2013; Wassmann and Stöckhert, 2013; Miyakawa and Kawabe, 2014; Kausala and Zhang, 2015; Ben-Itzhak et al., 2016; Pavel et al., 2016) which had more reports on the effect of the differential stress. Even though there have been occasional sporadic reports, the systematicity and completeness of these studies are still far from being applied to solve specific earth science problems. As a result, much of the knowledge on the earth’s interior material obtained based on the HT-HP experimental results are questionable. For instance, the effects of the differential stress at high temperatures and high (quasi) hydrostatic pressures on the phase transformation of geological materials have been reported only in the following extremely sporadic cases for pyroxene and quartz.
1) It is known that the Clinopyroxene-Orthorhombic pyroxene phase transition boundaries obtained at high temperatures and high pressures were significantly different from each other. Based on the thermodynamic analysis of previous experimental details and results, Coe (1970) proposed the effect of the shear stress on the temperature and pressure of the Clinopyroxene-Orthorhombic pyroxene phase transition. However, unfortunately, no specific experimental verification and further systematic study of this hypothesis have been carried out since then.
2) Zhou et al. (2005) studied the phase transition of quartz at the temperature of 950–1,000°C, the confining pressure of 1.3 GPa, and the axial compressive stress of 1.5–1.67 GPa. The pressure of the phase transition from α quartz to coesite was found to be closely related to the differential stress and the presence of coesite at the interface between the indenter and the sample. However, it is also unfortunate that this work was not continued later. Richter (2016) found the formation of coesite by the shear experiments at 600–900°C and 1–1.5 GPa, but only qualitatively discussed that the formation of coesite is closely related to the differential stress.
3) Singh et al. (2012) used the laser-heated diamond anvil cell synchrotron X-ray diffraction to find a significant difference in the transition pressure of stishovite from tetragonal to oblique phases in the differential stress and hydrostatic pressure environments (about 40 and 60 GPa, respectively). However, this work only found such phenomena, and the relationship between the differential stress and the phase transition pressure for a given temperature and (quasi) hydrostatic pressure could not be measured accurately in the anvil cell. Also, the essential correlation between the (quasi) hydrostatic pressure and the differential stress in the anvil cell was not investigated. So no quantitative results were available.
4) Demouchy and Mainprice (2011) used a HT-HP second-stage WC anvil apparatus using a 45° waist-cut sample assembly of the sample column to find that the shear stress had an important effect on the phase transition kinetics of forsterite-wadsleyite. However, in situ measurement of the shear stress and the essential correlation between the shear stress and the confining pressure could not be achieved. So the quantitative relationship between the temperature and the pressure of the phase transition reaction and the shear stress could not be obtained.
5) Niwa et al. (2012) used laser heating of diamond anvil cells in combination with a high-fluorescence miniaturized X-ray machine and found that the differential stress inside the diamond anvil cells could reduce the transition temperature of the silicate calixarene analog CaIrO3 from calixarene to post-calixarene to room temperature. However, it was also impossible to accurately measure the differential stress in the anvil cell and the essential correlation between the (quasi) hydrostatic pressure and the differential stress in the anvil cell. Therefore, the quantitative relationship between the differential stress and the temperature and pressure of the phase transition could not be obtained. Experimental studies on partial melting of geomaterials at high temperatures, high (quasi-) hydrostatic pressures, and high differential stresses were reported. But they focused on the relationship between the differential stress and the partial melting kinetics, the spatial distribution of the melt and melt segregation, as well as the constitutive and rheological behavior of the partially melted samples (Tumarkina et al., 2011; Holtzman and Benjamin, 2012). So the quantitative relationship between the differential stress and the temperature/pressure of the melt phase transition of the solid sample has not been reported yet. The effects of the differential stress on the physical properties of geological materials at high temperatures and high (quasi) hydrostatic pressures have been reported only for the conductivity of magnetite-bearing serpentine (Kawano et al., 2012) and polycrystalline olivine (Caricchi et al., 2011; Zhang et al., 2014). However, the experimental reports focused on the mechanism of the differential stress-sample structure-sample conductivity. There are no experimental reports on the effect of the differential stress on the core factors that directly constrain the conductivity of samples such as the electronic band structure, the lattice defect types and concentrations, and the carrier migration activation energy of the constituent minerals.
In summary, there are very few existing reports for the effects of the differential stress on the physicochemical properties of geographical materials. The majority of reports are missing quantitative results and only qualitatively discuss the contribution of the differential stress. This is mainly due to the slow research and development progress of differential stress generators.
High-Temperature and High-Pressure Experimental Apparatus Capable of Generating Differential Stress
The formation and evolution of various geological and tectonic phenomena in the earth is ultimately the result of the deformation of mineral rocks under complex physicochemical conditions. To better understand the deformation of the earth’s interior under various physical conditions such as the temperature, confining pressure, differential stress, and strain rate, as well as chemical environments such as the oxygen fugacity and fluids in the real earth’s interior, special HT-HP experimental apparatus are needed to test the mechanical properties of the earth’s interior. In the 1960s, David Griggs, a pioneer in the field of international tectonics, developed a new high-temperature, high-pressure deformation device, the Griggs rheometer (Griggs, 1967), by modifying a piston-cylinder high-pressure device as shown in Figure 1A. This device can be used to determine the stress-strain properties of geographical materials and also to qualitatively study the microstructural evolution of deformation. Based on the work of predecessors, scientists have developed a new generation of Griggs deformation apparatus, such as Ghaffari has reported on the development of an ultrasound probe array that allows us to monitor deforming samples in a high-pressure, high-temperature solid medium apparatus (Ghaffari and Pec, 2020). However, the Griggs rheometer employing a solid medium cannot obtain true isotropy of the confining pressure and the friction between the loading axis and the confining pressure medium makes the differential stress measurement quite inaccurate (Ji et al., 2008). To overcome these disadvantages of the Griggs rheometer, Paterson, et al. of the Australian National University designed the Paterson rheometer (Paterson, 1970) which is a deformation device employing a gas medium as shown in Figure 1B. However, because the Paterson rheometer uses a gaseous pressure medium, the confining pressure obtainable is very limited (≤700 MPa) and this method cannot be combined with other in situ measurement techniques. In the 21st century, Wang et al. (2003) developed the Deformation-DIA (Figure 1C) and Yamazaki and Karato (2001) developed the Rotational Deformation Apparatus (Figure 1D) for HT-HP deformation measurement. The D-DIA consists of four peripheral wedges with a trapezoidal cross-section sliding on a 45° slope and a set of upper and lower symmetrical guide blocks. The upper and lower anvils can move independently of the four surrounding anvils, thus generating the differential stress. The most important feature of the RDA device is that the upper and lower anvils can rotate relative to each other. The rotation of the anvil will cause a large frictional force between the anvil surface and the sample, thus generating large shear stress in the sample. Both D-DIA and RDA can now reach a very high confining pressure of about 25 GPa and can be used in combination with the synchrotron X-ray diffraction. So they are greatly contributing to the development of solid-state geosciences. The diamond-to-top anvil pressure chamber (Figure 1E) is another important HT-HP device that can be easily combined with synchrotron radiation. The small size of the sample chamber (in the order of microns), the uneven heating of the sample, and the incomplete separation of pressure generation and plastic deformation in the DAC experiments make rheological studies with DAC difficult, especially because of the large errors in the stress-strain measurements (Niwa et al., 2012). The temperature and pressure range of the existing differential stress apparatus is shown in Table 1.
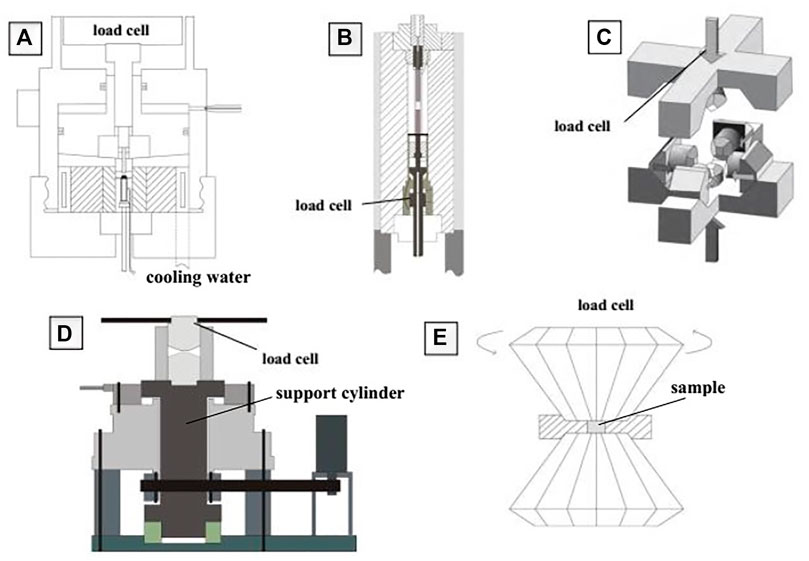
FIGURE 1. Differential stress apparatus. (A) Griggs deformation apparatus (Griggs, 1967), (B) Paterson deformation apparatus (Paterson, 1970), (C) Deformation-DIA (Wang et al., 2003), (D) Rotational Deformation Apparatus (Yamazaki and Karato, 2001), (E) Diamond anvil cell (Niwa et al., 2012).
The prominent influence of the differential stress on the material properties and mineral phase transition is gaining more and more attention, while the current technically mature differential stress experimental apparatus has their own drawbacks and shortcomings. Hence, there is an urgent need to develop a HT-HP experimental apparatus that can accurately simulate the temperature, hydrostatic pressure, and differential stress in the earth’s interior and can be easily interfaced with various in situ measurement techniques to investigate the physical properties.
Conclusion and Outlook
With the rapid development and advancement of HT-HP experimental techniques, the effects of the differential stress on the physicochemical properties of rocks and minerals have been clarified. The results of these studies are of great importance for the deep understanding of geological processes in the earth’s interior, deformation of the earth’s internal circles, plate tectonics, geodynamics, and interpretation of geophysical observations. However, due to the limitation of the equipment, there are still many kinds of researches required regarding the effect of the differential stress on the physicochemical properties of matters such as the measurement of physical properties and experiments under the differential stress which is inseparable from the development of engineering techniques.
However, the confining pressure and produced stress that can be obtained by the existent differential stress experiments are limited. With the development of synchrotron radiation technology and various large-chamber rheometer apparatus, it is hoped that this research field can be extended to the bottom of the lower mantle and even core conditions in the future. Synchrotron radiation technology should be combined with more in situ measurement techniques so that many new phenomena, new effects, and new properties of the earth’s interior under high temperatures, high pressures, and differential stresses can be further discovered and applied.
Author Contributions
All authors listed have made a substantial, direct, and intellectual contribution to the work and approved it for publication.
Funding
This project is supported by the National Natural Science Foundation of China, Project No. 42104176.
Conflict of Interest
The authors declare that the research was conducted in the absence of any commercial or financial relationships that could be construed as a potential conflict of interest.
Publisher’s Note
All claims expressed in this article are solely those of the authors and do not necessarily represent those of their affiliated organizations or those of the publisher, the editors, and the reviewers. Any product that may be evaluated in this article, or claim that may be made by its manufacturer, is not guaranteed or endorsed by the publisher.
References
Anil, S. K., Andrault, D., and Bouvier, P. (2012). X-ray Diffraction from Stishovite under Nonhydrostatic Compression to 70 GPa: Strength and Elasticity across the Tetragonal-Orthorhombic Transition. Phys. Earth Planet. Interiors 208-209, 1. doi:10.1016/j.pepi.2012.07.003
Benjamin, H. K., King, D. S. H., and Kohlstedt, D. L. (2012). Effects of Stress-Driven Melt Segregation on the Viscosity of Rocks. Earth Planet. Sci. Lett. 359-360, 184. doi:10.1016/j.epsl.2012.09.030
Ben-Itzhak, L. L., Erez, J., and Aharonov, E., (2016). Precipitation of CaCO3 in Pressure Solution Experiments: The Importance of Damage and Stress [J]. Earth and Planetary Science Letters: A Letter Journal Devoted to the Development in Time of the Earth and Planetary System 434, 30–41.
Budnitzki, M., and Kuna, M. (2016). Stress Induced Phase Transitions in Silicon. J. Mech. Phys. Sol. 95 (1), 63–91. doi:10.1016/j.jmps.2016.03.017
Cao, L. Z., Cheng, B. L., Wang, S. Y., Fu, W. Y., Ding, S., Sun, Z. H., et al. (2006). Influence of Stress on Raman Spectra in Ba1−xSrxTiO3thin Films. J. Phys. D: Appl. Phys. 39, 2819–2823. doi:10.1088/0022-3727/39/13/027
Caricchi, L., Gaillard, F., Mecklenburgh, J., and Le Trong, E. (2011). Experimental Determination of Electrical Conductivity during Deformation of Melt-Bearing Olivine Aggregates: Implications for Electrical Anisotropy in the Oceanic Low Velocity Zone. Earth Planet. Sci. Lett. 302, 81–94. doi:10.1016/j.epsl.2010.11.041
Coe, R. S., Gilder, S. A., and Wright, J. P. (2012). The Thermodynamic Effect of Nonhydrostatic Stress on the Verwey Transition. Earth Planet. Sci. Lett. 319-320, 207–217. doi:10.1016/j.epsl.2011.11.021
Coe, R. S. (1970). The Thermodynamic Effect of Shear Stress on the Ortho-Clino Inversion in Enstatite and Other Coherent Phase Transitions Characterized by a Finite Simple Shear. Contr. Mineral. Petrol. 26, 247–264. doi:10.1007/bf00373203
Daniel, G. A., Schmitt, M. P., Robinson, J. A., and Wolfe, D. E. (2014). Stress Induced Phase Transition in Gd2O3 Films by Ion Beam Assisted Reactive Electron Beam-Physical Vapor Deposition (EB-PVD). Surf. Coat. Techn. 242, 68. doi:10.1016/j.surfcoat.2014.01.020
Demouchy, S., Mainprice, D., Tommasi, A., Couvy, H., Barou, F., Frost, D. J., et al. (2011). Forsterite to Wadsleyite Phase Transformation under Shear Stress and Consequences for the Earth's Mantle Transition Zone. Phys. Earth Planet. Interiors 184, 91–104. doi:10.1016/j.pepi.2010.11.001
Ghaffari, H. O., and Pec, M. (2020). An Ultrasound Probe Array for a High-Pressure, High-Temperature Solid Medium Deformation Apparatus. Rev. Scientific Instr. 91 (8), 085117. doi:10.1063/5.0004035
Grave, J. D., Glorie, S., and Buslov, M. M., (2014). Detrital Zircon Provenance of Early Palaeozoic Sediments at the Southwestern Margin of the Siberian Craton: Insights From U–Pb geochronology [J]. J. Asian Earth Sci. 82 (3), 115–123.
Griggs, D. (1967). Hydrolytic Weakening of Quartz and Other Silicates. Geophys. J. Int. 14 (1-4), 19–31. doi:10.1126/science.152.3722.674-a
Holtzman, K., and Benjamin, , (2012). Effects of Stress-Driven Melt Segregation on the Viscosity of Rock [J]. Earth Planet. Sci. Lett. 2, 123–135.
Imber, D., Faulkner, D. R., Mitchell, T. M., and Rutter, E. H., (2008). On the Structure and Mechanical Properties of Large Strike-Slip Faults [J]. Geol. Soc. Spec. Publ. 299 (1), 139–150.
Ji, L. D., Shuwen, , and Zhang, , (2008). Jurassic Tectonic Revolution in China and New Interpretation of the “Yanshan Movement” [J]. Acta Geologica Sinica English Edition 3 (2), 236–258.
Karato, S. I. (2008). Deformation of Earth Materials: An Introduction to the Rheology of Solid Earth. Cambridge: Cambridge University Press, 1.
Kausala, M., and Zhang, L. (2015). Stress-induced Phase and Structural Changes in KDP Crystals. Comput. Mater. Sci. 109, 359. doi:10.1016/j.commatsci.2015.07.044
Kawano, S., and Yoshino, T. (2012). Electrical Conductivity of Magnetite-Bearing Serpentinite during Shear Deformation. Geophys. Res. Lett. 39, L20313. doi:10.1029/2012gl053652
Kawano, S., Yoshino, T., and Katayama, I. (2012). Electrical Conductivity of Magnetite-Bearing Serpentinite during Shear Deformation. Geophys. Res. Lett. 39 (20), 20313. doi:10.1029/2012gl053652
Kawamoto, E., and Shimamoto, T., (1997). The Strength Profile for Bimineralic Shear Zones: An Insight From High-Temperature Shearing Experiments on Calcite–Halite Mixtures [J]. Tectonophysics 295 (1), 1–14. doi:10.1016/S0040-1951(98)00112-7
Ken, N., Nobuyoshi, M., Yusuke, S., Kenya, O., Hirotada, G., and Takehiko, Y. (2012). In Situ observation of Shear Stress-Induced Perovskite to post-perovskite Phase Transition in CaIrO3 and the Development of its Deformation Texture in a diamond-anvil Cell up to 30 GPa. Phys. Earth Planet. Interiors 194-195, 10. doi:10.1016/j.pepi.2012.01.007
Korotaev, A. D., Ovchinnikov, S. V., and Pochivalov, Y. I., (2016). Structure-Phase States of the Metal Surface and Undersurface Layers After the Treatment by Powerful Ion Beams [J]. Surf. Coat. Technol. 105 (1-2), 84–90.
Li, S., Ya, G., and Nan, C. (2014). Strain-induced Modulation of Magnetic Anisotropy in Co/BaTiO3 Composite. Chin. Sci. Bull. 59, 5191–5193. doi:10.1007/s11434-014-0595-9
Li, X., Xi, Z., Liu, P., Long, W., and Fang, P. (2015). Stress and Temperature-Induced Phase Transitions and thermal Expansion in (001)-cut PMN-31PT Single crystal. J. Alloys Comp. 652, 287–291. doi:10.1016/j.jallcom.2015.08.233
Liu, M., Johnston, M. B., and Snaith, H. J., (2013). Efficient Planar Heterojunction Perovskite Solar Cells by Vapour Deposition [J]. Nature 501 (7467), 395.
Ma, Y., Selvi, E., Levitas, V. I., and Hashemi, J. (2006). Effect of Shear Strain on the α-ε Phase Transition of Iron: a New Approach in the Rotational diamond Anvil Cell. J. Phys. Condens. Matter 18, S1075–S1082. doi:10.1088/0953-8984/18/25/s14
Miyakawa, K., and Kawabe, I., (2014). Pressure Solution of Quartz Aggregates Under Low Effective Stress (0.42–0.61 MPa) at 25–45°C [J]. Applied Geochemistry 40, 61–69.
Mylvaganam, , Kausala, , and Zhang, , (2015). Effect of Crystal Orientation on the Formation of Bct-5 Silicon [J]. A. Materials science & processing 10, 62–79.
Niemeijer, A. R., and Spiers, C. J., (2006). Velocity Dependence of Strength and Healing Behaviour in Simulated Phyllosilicate-Bearing Fault Gouge [J]. Tectonophysics 1, 12–36. doi:10.1016/j.tecto.2006.03.048
Niwa, K., Miyajima, N., Seto, Y., Ohgushi, K., Gotou, H., and Yagi, T. (2012). In Situ observation of Shear Stress-Induced Perovskite to post-perovskite Phase Transition in CaIrO3 and the Development of its Deformation Texture in a diamond-anvil Cell up to 30GPa. Phys. Earth Planet. Interiors 194-195, 10–17. doi:10.1016/j.pepi.2012.01.007
Paterson, M. S. (1970). A High-Pressure, High-Temperature Apparatus for Rock Deformation. Int. J. Rock Mech. Mining Sci. Geomechanics Abstr. 7 (5), 517–526. doi:10.1016/0148-9062(70)90004-5
Pavel, K., Pavel, P., and Aleksey, Y. (2016). The Role of Non-hydrostatic Stresses in Phase Transitions in boron Carbide. Comput. Mater. Sci. 121, 106. doi:10.1016/j.commatsci.2016.04.041
Richter, , (2016). Stresses and Pressures at the Quartz-to-Coesite Phase Transformation in Shear Deformation Experiments [J]. Journal of Geophysical Research Solid Earth Jgr 1, 1312–1335.
Rowe, C. D., and Griffith, W. A., (2015). Do Faults Preserve a Record of Seismic Slip: A Second Opinion [J]. J. Struct. Geol. 78, 1–26.
Seyidov, M. Y., Mikailzade, F. A., Suleymanov, R. A., Bulut, N., and Salehli, F. (2016). The Influence of Uniaxial Compressive Stress on the Phase Transitions and Dielectric Properties of NaNO 2. J. Phys. Chem. Sol. 93, 22–26. doi:10.1016/j.jpcs.2016.02.004
Siakavellas, M., Raptis, Y. S., Anastassakis, E., and Lockwood, D. J. (1997). Strain Effects on InSb Phonons in Bulk and Superlattice Layers. J. Appl. Phys. 82, 6235–6239. doi:10.1063/1.366509
Singh, K. M., Ahir, V. B., and Tripathi, A. K., (2012). Metagenomic Analysis of Surti Buffalo (Bubalus Bubalis) Rumen: A Preliminary Study [J]. Molecular Biology Reports 39 (4), 4841–4848.
Toy, M., French, A. L., Adeyemi, O. M., and Agniel, D. M., (2011). The Association of HIV Status With Bacterial Vaginosis and Vitamin D in the United States [J]. J. Womens Health 20 (10), 1497–1503.
Tullis, J., and Yund, R. A., (1991). Diffusion Creep in Feldspar Aggregates: Experimental Evidence [J]. J. Struct. Geol. 13 (9), 987–1000.
Tumarkina, E., Misra, S., Burlini, L., and Connolly, J. A. D. (2011). An Experimental Study of the Role of Shear Deformation on Partial Melting of a Synthetic Metapelite. Tectonophysics 503, 92–99. doi:10.1016/j.tecto.2010.12.004
Wang, Y., Durham, W. B., Getting, I. C., and Weidner, D. J. (2003). The Deformation-DIA: A New Apparatus for High Temperature Triaxial Deformation to Pressures up to 15 GPa. Rev. Scientific Instr. 74 (6), 3002–3011. doi:10.1063/1.1570948
Wassmann, D., and Stöckhert, M., (2013). Seismic Visibility of a Deep Subduction Channel: Insights From Numerical Simulation of High-Frequency Seismic Waves Emitted From Intermediate Depth Earthquakes [J]. Solid Earth Discussions.
Xu, H., Jian, A., Xue, C., Chen, Y., Zhang, B., and Zhang, W. (2008). Temperature Dependence of Biaxial Strain and its Influence on Phonon and Band gap of GaN Thin Film. Chin. Phys. B 17, 2245. doi:10.1088/1674-1056/17/6/051
Xu, Y., Yan, Y., Eli Young, S., and Feng, Y. (2016). Influence of Perpendicular Compressive Stress on the Phase Transition Behavior in (Pb,La,Ba,) (Zr,Sn,Ti)O3 Antiferroelectric Ceramics. Ceramics Int. 42, 721–726. doi:10.1016/j.ceramint.2015.08.170
Yamazaki, D., and Karato, S.-i. (2001). High-pressure Rotational Deformation Apparatus to 15 GPa. Rev. Scientific Instr. 72 (11), 4207–4211. doi:10.1063/1.1412858
Zhang, B., Yoshino, T., Yamazaki, D., Manthilake, G., and Katsura, T. (2014). Electrical Conductivity Anisotropy in Partially Molten Peridotite under Shear Deformation. Earth Planet. Sci. Lett. 405, 98–109. doi:10.1016/j.epsl.2014.08.018
Zhou, Y., He, C., Song, J., Ma, S., and Ma, J. (2005). An experiment Study of Quartz-Coesite Transition at Differential Stress. Chin.Sci.Bull. 50, 446–451. doi:10.1007/bf02897461
Keywords: differential stress, differential stress generator, high temperature, high pressure, phase transition
Citation: Ren D and Li H (2022) A Review of High-Temperature and High-Pressure Experimental Apparatus Capable of Generating Differential Stress. Front. Earth Sci. 10:852403. doi: 10.3389/feart.2022.852403
Received: 11 January 2022; Accepted: 31 January 2022;
Published: 07 March 2022.
Edited by:
Vassilios Saltas, Hellenic Mediterranean University, GreeceCopyright © 2022 Ren and Li. This is an open-access article distributed under the terms of the Creative Commons Attribution License (CC BY). The use, distribution or reproduction in other forums is permitted, provided the original author(s) and the copyright owner(s) are credited and that the original publication in this journal is cited, in accordance with accepted academic practice. No use, distribution or reproduction is permitted which does not comply with these terms.
*Correspondence: Heping Li, TGloZXBpbmdAdmlwLmd5aWcuYWMuY24=