- 1CAS Key Laboratory of Crust-Mantle Materials and Environments, School of Earth and Space Sciences, University of Science and Technology of China, Hefei, China
- 2CAS Center of Excellence in Comparative Planetology, Hefei, China
- 3Laboratory for Isotopes in Economic Geology and its Environment (LIEGE), Department of Mineral Resources and Engineering, School of Earth Sciences, China University of Geosciences (Wuhan), Wuhan, China
- 4School of Resources and Environmental Engineering, Hefei University of Technology, Hefei, China
Lithium isotope geochemical studies of oceanic-type eclogites benefited from previous investigation of altered oceanic crust; however, processes of lithium isotope exchange operating on altered protoliths of continental-type eclogites have remained unclear. Here, the Li concentration and isotopic composition of fresh and altered continental basalts from the South China Block were measured to decipher Li and its isotopic fractionation during subaerial alteration processes. The results show that altered South China basalts have higher Li concentration (11.4–117.7 ppm) and lower δ7Li values (−4.3–+0.5‰) than fresh basalts (Li: 5.0 ppm and δ7Li: ∼3‰). It is found that hydrothermal alteration played an important role in increasing the Li concentration of altered basalts, which is supported by the relation of loss on ignition and Li concentration. Subsequently, continental weathering acted on chlorite-bearing basalts, as evidenced by the occurrence of minor kaolinite and illite and weathering trend of δ7Li values in these basalts. Detailed petrography and in situ geochemical analyses are used to show that chlorite is the chief repository for Li in weathered basalts, supporting that it was responsible for decreasing δ7Li value by Rayleigh distillation during weathering. Because of the lower δ7Li values of continental altered basalts than oceanic altered crust, it is concluded that, in addition to the dehydration of subducted slabs and possible Li diffusion, the extremely light Li isotopic values of continental-type eclogites worldwide partly result from isotope fractionation caused by the continental weathering of their protoliths.
Introduction
As a fluid-mobile element, lithium and its isotopes are deemed to represent powerful tracers of different types of fluid–rock reactions, including those operating in subduction zones, hydrothermal alteration, continental weathering, and altered oceanic crusts (AOCs) (Tomascak et al., 2016; Penniston-Dorland et al., 2017). The high- or ultrahigh-pressure (HP/UHP) metamorphic rocks found in subduction zones provide a direct window through which subduction-related fluids may be investigated (Bebout, 2014; Schmidt and Poli, 2014). Eclogites, as common HP-UHP metamorphic rocks from subduction zones, were found to have higher Li concentrations ([Li]) and lower δ7Li values than their MORB-type protoliths (Zack et al., 2003; Marschall et al., 2007; Penniston-Dorland et al., 2010; Simons et al., 2010; Halama et al., 2011; Xiao et al., 2011; El Korh et al., 2019; Liu et al., 2019). Because of the limited Li isotopic fractionation occurring during the dehydration of the subducted oceanic crust (Marschall et al., 2007), most models for Li isotopic fractionation have focused on the metasomatism of the AOC and/or the diffusion of Li from country rocks or fluids during exhumation (Marschall et al., 2007; Simons et al., 2010; Halama et al., 2011; Liu et al., 2019) and attempted to explain the increase in [Li] and the decrease in δ7Li values regardless of whether the eclogites were oceanic or continental. However, the behavior of Li isotopes in fluid–rock interaction differs significantly in ocean floor alteration and continental weathering. First, seawater (31.0 ± 0.1‰; Millot et al., 2004) and submarine hydrothermal fluids (+8.0 ± 1.9‰; Verney-Carron et al., 2015) have relatively constant δ7Li values, compared to the magmatic, metamorphic, sedimentary, and meteoric fluids present in continental settings and which vary significantly in [Li] and δ7Li values (12–653 ppm, -1.4–41.3‰; Richard et al., 2018). Second, previous studies showed that the δ7Li values of the AOC generally increase with decreasing fluid temperature and increasing fluid/rock ratios along the ocean drilling profiles (Chan et al., 2002; Gao et al., 2012; Coogan et al., 2017) and the addition of mobile elements (Li, Rb, K, Cs, etc.) in the AOC (Staudigel, 2014). However, most weathered continental basalts show lower δ7Li values than their fresher equivalents and simultaneously lose mobile elements (Ca, Na, K, and Li) and accumulate immobile elements (Nb and Ti) as evidenced by weathering profiles (Kısakürek et al., 2004; Rudnick et al., 2004; Liu et al., 2013; Tong et al., 2021). Therefore, using the AOC to model continental eclogites is proving to be inadequate, and it is indispensable to investigate the Li isotopic compositions of continental altered basaltic rocks for deciphering variations in [Li] and δ7Li in continental-type eclogites. Here, detailed investigations of mineralogy, major and trace element concentrations, and Li isotopic compositions were executed on fresh and altered basalts from the South China Block in order to assess the behavior of Li isotopes during hydrothermal alteration and weathering. Our results demonstrate that hydrothermal alteration brought the high [Li] to the altered basalts, while chlorite-bearing altered basalts subsequently underwent weathering, which resulted in a decrease in their δ7Li values. Different styles of lithium isotopic fractionation were found in altered basalts from continental and oceanic settings, providing a new prospective of protolith on explaining the formation of light Li in continental-type eclogites.
Geological Background and Samples
The South China Block is composed of the Yangtze Block to the northwest and the Cathaysia Block to the southeast, both of which are separated by the Neoproterozoic Jiangnan orogenic belt (Chen et al., 2008). The widely developed granitoids in the South China Block were coeval with bimodal volcanic suites consisting mostly of felsic rocks with minor amounts of basalts, which are scattered throughout the granitoids (Figure 1A). The Cenozoic and Mesozoic intraplate basalts are sporadically scattered in the Cathaysia Block. These basalts are spatially and temporally associated with the subduction of the Paleo-Pacific Plate and have mixed enriched-mantle Ⅱ (EM2) and depleted mantle sources (Ho et al., 2003; Chen et al., 2008; Zeng et al., 2016). However, compared to fresh Cenozoic basalts, most Mesozoic basalts underwent varying degrees of chloritization and epidotization (Meng et al., 2012; Cen et al., 2016), thus providing an opportunity to study the Li isotopic behavior during low-temperature alteration processes. Sampling was focused in the Fujian Province (Figure 1A), and samples were divided into fresh South China basalts (fresh SCBs, n = 13) and altered South China basalts (altered SCBs, n = 13).
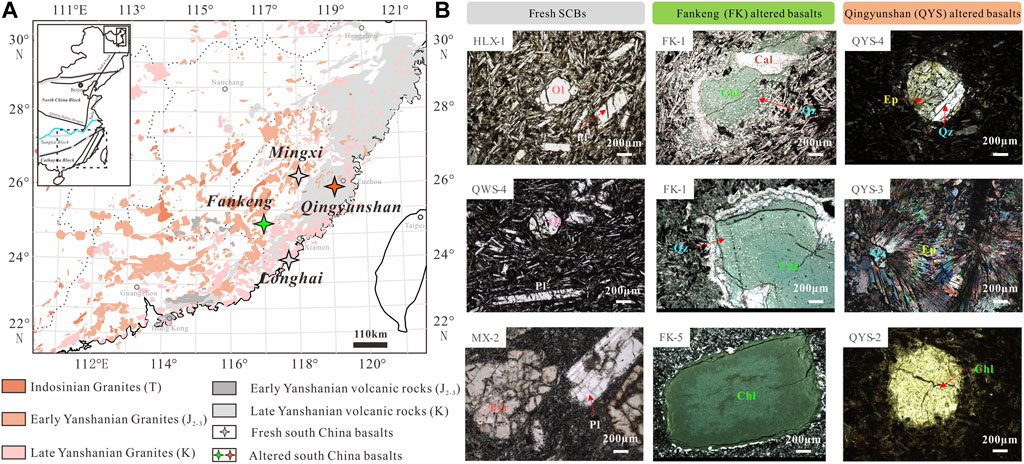
FIGURE 1. (A) Simplified geological map of the distribution of granite-volcanic rocks in South China (modified from the work of Zhou et al. (2006)). (B) Polarized photomicrograph of main mineral phases in fresh and altered basalts (QYS and FK basalts). Ol, olivine; Pyr, pyroxene; Pl, plagioclase; Chl, chlorite; Cal, calcite; Qz, quartz; and Ep, epidote.
The fresh SCBs group includes seven samples from Mingxi and six from Longhai, which erupted at 1.2–2.2 Ma and 14.9–17.1 Ma, respectively (Ho et al., 2003). The basalts include mainly olivine and plagioclase and rarely pyroxene phenocrysts, whereas the groundmass is composed of microcrystalline plagioclase, fine-grained pyroxene, and magnetite (Figure 1B). The altered SCBs group includes seven Qingyunshan (QYS) basalts from the 81–85 Ma bimodal volcanic Yongtai region (Meng et al., 2012) and six Fankeng (FK) basalts from the ∼98 Ma bimodal volcanic Fankeng formation (Chen et al., 2008), respectively. The QYS basalts, which were sampled from the fresh outcrop, underwent epidotization and partial chloritization, while FK basalts, which were sampled from the riverbed, underwent variable degrees of chloritization. The QYS basalts with amygdaloidal textures are interspersed with veins consisting of epidote and quartz, whereas the FK basalts have large-grained chlorite occasionally surrounded by quartz and calcite (Figure 1B). Chlorite is the dominant secondary mineral for this study. For comparison with the altered SCBs, the chlorite-bearing hydrothermally altered basalts (HABs) from Hengill, SW Iceland (Verney-Carron et al., 2015), and weathered diabase dyke (WDD) developed on chlorite-bearing diabase from South Carolina, United States (Rudnick et al., 2004), were selected. Both sets of samples were selected because their low-T meteoric alteration is well documented. Within the WDD sample suite, only those located at >6 m depth were considered because they preserved basaltic compositions.
Analytical Methods
Mineral Identification
Sample mineralogy was determined at the CAS Key laboratory of Crust-Mantle Materials and Environments, University of Science and Technology of China (USTC). Aliquots of the FK basalts were subsequently crushed and pulverized to <200 mesh for analysis by MapAHF X-ray diffraction (XRD) using a Rigaku Smartlab X-ray diffractometer equipped with a Cu tube, at 2°/min and 0.02° step width, from 3° to 80° 2θ.
Whole Rock Major and Trace Elements
Rock powders were mixed with Li tetraborate in Pt crucibles with release agents to produce glass discs. The glass discs were analyzed using a Rigaku ZSX Primus II WD X-ray fluorescence spectrometer (XRF) at USTC. Analytical precision for each major element was better than 1%. Loss on ignition (LOI) was measured by gravimetric methods in a muffle furnace. For trace element analysis, sample powders were dissolved in Teflon bombs by heating for 2 days at 200°C in mixed ultra-pure HF, HNO3 and HClO4. After adding Rh as the internal standard, the analyses were performed using an ELAN DRC II quadrupole inductively coupled plasma-mass spectrometer (ICP-MS) at USTC. The samples were carried by 0.85 L/min high-purity argon gas. The analytical uncertainty was better than 5%.
Analyses of Chlorite
The analyses of major and trace elements in chlorite were conducted at USTC. The major elements were determined on a JEOL JXA-8530 electron microprobe under 15 kV accelerating voltage, 10 nA beam current, and 5 μm beam size. The analytical uncertainty was <1%. The trace element composition of chlorite from the FK basalts was determined using a Coherent ArF excimer UV 193 nm wavelength GeoLas procoupled with an Agilent 7700e quadrupole mass spectrometer (LA-ICP-MS). The sampling spot size was 60 μm. The laser was operated at a frequency of 10 Hz, and ablation times were 40 s. Detailed instrument parameters and analytical methods are given in the work of Hou et al. (2020).
Lithium Isotope Analysis
Rock powders were digested in a 1:1 mixture of ultra-pure concentrated HF (24 M) and HNO3 (16 M) for 24 h at 150°C in Teflon vials, then remobilized in 2 mL 8 M HCl, and refluxed in 3 mL 0.2 M HCl to prepare for cation exchange chromatograph. Detailed liquid chromatography procedures for Li and other ion separation were from the work of Gao and Casey (2012) and Sun et al. (2016). The Li isotopic compositions were measured on a Thermo Scientific Neptune Plus MC-ICP-MS at USTC. The results are expressed as δ7Li = {[(7Li/6Li)sample/(7Li/6Li)L-SVEC -1]×1000}. The long-term external precision of Li isotopic standard L-SVEC and laboratory Li standard solutions (QC) was better than 0.3‰. Repeated determinations of international rock standards (BHVO-2 and BCR-2) showed consistency with previous studies (Supplementary Table S2).
Results
Mineral Characteristics
Optical microscope observations from fresh basalts show phenocrysts of olivines, plagioclases, and pyroxenes, while radial epidote, quartz, and minor chlorite are present in the altered QYS basalts (Figure 1B). In the FK basalts, some chlorite pseudomorphs retained the shape of primary ferromagnesian minerals, and tiny blobs of quartz and carbonate are located at crystal edges or are distributed within the matrix (Figure 1B). The different proportion of chlorite and other minerals in the FK basalts was estimated based on the quantitative analysis of MDI Jade Easy Quant XRD software (Supplementary Table S1).
Major and Trace Element Compositions
The major and trace element composition of basalts is presented in Supplementary Table S2. All major elements in altered South China basalts (SCBs) are recalculated to 100% without volatiles. The altered and fresh SCBs are similar in major elements and REE pattern (Supplementary Figure S3). The [Li] was not associated with MgO but related with LOI in the altered SCBs (Figures 2A,B). Relative to fresh SCBs, the altered SCBs have higher Cs, Pb and Li (Figure 2C). Comparing to the QYS basalts, the FK basalts show lower content of U and K and different variation trends of Nb versus Ba (Figures 2C,D).
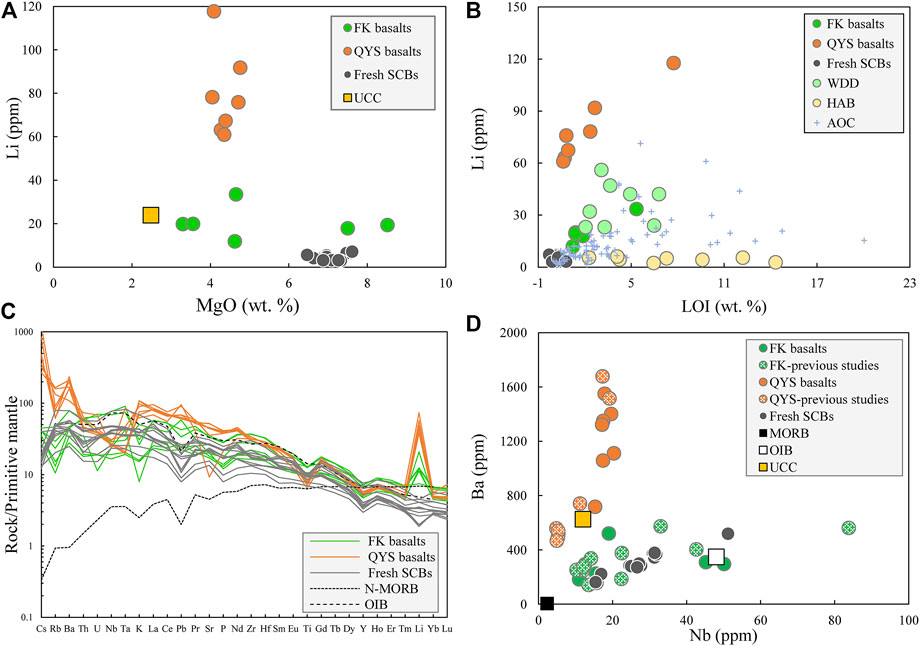
FIGURE 2. Geochemical plots of fresh and altered South China basalts (SCBs) showing (A) MgO vs. Li and (B) LOI vs. Li. The weathered diabase dykes from South Carolina (WDD) below a 6 m boundary and hydrothermally altered basalts (HABs) from Hengill in SW Iceland are from the work of Rudnick et al. (2004) and Verney-Carron et al. (2015), and data of the altered oceanic crust (AOC) are compiled in Supplementary Table S3. (C) Primitive mantle normalized trace element pattern for the fresh and altered SCBs. The data of primitive mantle are from the work of McDonough and Sun (1995), and MORB/OIB data are from the work of Sun and McDonough (1989). (D) Nb vs. Ba. The previous dataset for Fankeng (FK) basalts is from the work of Zhou et al. (2005) and Chen et al. (2008), and Qingyunshan (QYS) basalts are from the work of Chen et al. (2008) and Meng et al. (2012). The upper continental crust (UCC) is from the work of Rudnick and Gao (2014).
Chlorite Composition
The major and trace element compositions of chlorite in altered SCBs are integrated in Supplementary Tables S4, S5, respectively. The WinCacc program coded by Yavuz et al. (2015) was used for the classification of chlorite. All chlorites from altered SCBs are clinochlore (Mg-chlorite) in discrimination diagrams (Supplementary Figures S2A,B). The whole rock-normalized trace elements in chlorites from the QYS and FK basalts show that clinochlore in altered SCBs have higher [Li] than their corresponding whole rocks (Supplementary Figure S2C). According to the chlorite geothermometer in the WinCacc program, the formation temperature of chlorite varies from 156 to 243°C in the FK basalts and 114–253°C in the QYS basalts (Supplementary Table S6).
Lithium Isotope Composition
The δ7Li values and (Li) of fresh and altered SCBs are provided in Supplementray Table S2. The altered SCBs have higher [Li] (11.9–117.7 ppm) and lower δ7Li values than fresh SCBs (5.0 ppm; +3.0‰) (Figures 3A,B). The δ7Li values of most QYS basalts (+0.4 to +0.5‰) are homogeneous, with two samples having some chlorites (QYS-2 and QYS-3) and lower δ7Li values (−1.6‰ and −0.8‰). It is worth noting that the FK basalts have higher (Li) and lower δ7Li values than the altered oceanic crust at any given LOI and [Li] (Supplmentary Table S3) (Figures 3A,B). The FK basalts host more chlorite than most QYS basalts, and the lighter δ7Li in the FK basalts are associated with greater amounts of chlorite and their formation temperature (Figure 3C).
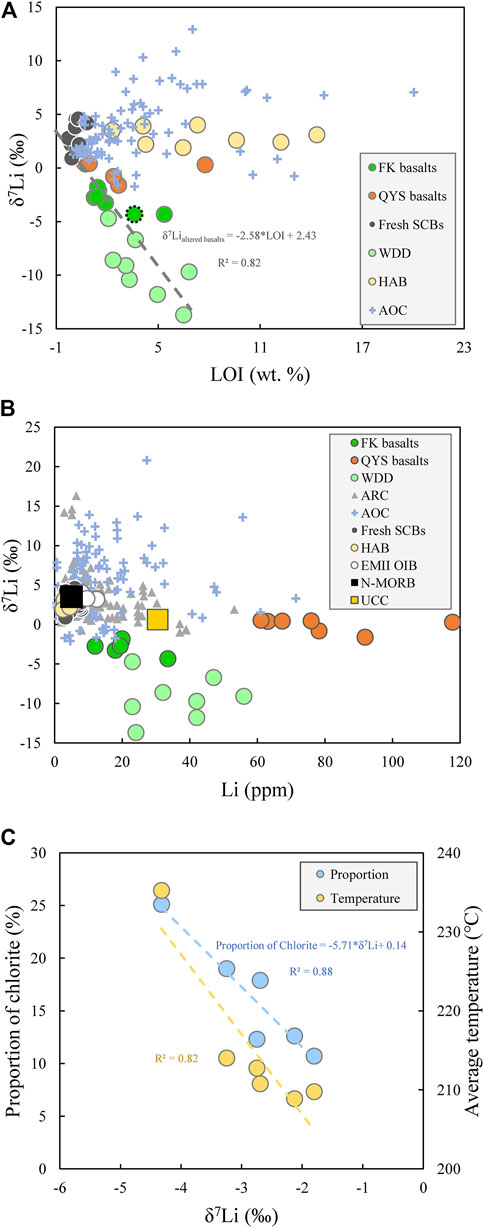
FIGURE 3. Geochemical plots showing (A) LOI vs. δ7Li. The fitted function is established on fresh SCBs, FK basalts, and WDD. For eliminating the effect of carbonates, the LOI of one sample (FK-1) was calibrated to the dotted green circle (LOI*≈LOI-fcalcite*44). (B) [Li] vs. δ7Li. The fresh MORB are from the work of Marschall et al. (2017). The ocean island basalts (OIBs) from the EM2 mantle reservoir and the upper continental crust (UCC) are from the work of Krienitz et al. (2012) and Teng et al. (2008). The data sources of the altered oceanic crust (AOC) and arc lavas are summarized in Supplmentary Table S3. (C) The formation temperature and proportion of chlorite as a function of δ7Li values for the FK basalts: proportion of chlorite = -5.71*δ7Li+0.14, R2 = 0.88. The higher chlorite formation temperatures are associated with lower δ7Li values and greater proportions of chlorite.
Discussion
Origin of High Li Concentrations in South China Basalts
The altered SCBs, especially the QYS basalts, have higher Li concentration ([Li]) than fresh SCBs and the altered oceanic crust (AOC) (Figures 2A,B). Due to its moderate incompatibility in mafic rocks, the [Li] generally increases with basaltic magma differentiation (Ryan and Kyle, 2004). However, such signature is not found in altered SCBs (Figure 1B). Crustal assimilation occurred in the QYS basalts based on low Nb/La ratios and negative εNd (t) values (Meng et al., 2012) and negligible crustal assimilation by the FK basalts based on the relation of SiO2 and εNd (t) (Cen et al., 2016). Notwithstanding the low Nb/U ratio and high [Li] of the upper continental crust (Nb/UUCC 4.4 and [Li]UCC 24 ± 5 ppm, Rudnick and Gao 2014), it is impossible to obtain the extremely high (Li) of QYS basalts (on average, Nb/UQYS-10 11.0 and [Li] QYS-10 117.7 ppm) by mixing fresh basalt with the upper continental crust (Supplementary Figure S3D).
The dominant secondary mineral is epidote in QYS basalts and chlorite in FK basalts, whereas no secondary minerals are observed in all fresh SCBs (Figure 1B). The chlorites from QYS and FK basalts are classified as Mg-chlorite (Supplementary Figure S2), and most Mg-chlorite and epidote form at temperatures >150°C (Beaufort et al., 2015; Bird and Spieler 2004), in agreement with chlorite formation temperatures in our study (Supplementary Table S6). Numerous studies of fluid–rock reactions between fluids and olivine or basalt show the precipitation of chlorite precipitating in the vicinity of carbonates and quartz at ≥ 150°C (Gysi and Stefánsson 2012; Li et al., 2018), which is akin to the scene observed in altered SCBs (Figure 1B). The correlation between LOI and [Li] suggests that hydrothermal alteration played an important role in increasing [Li] in the QYS and FK basalts (Figure 2B). Chlorite readily incorporates Li from fluid phases (Xiao and Chen 2020). The higher [Li] in chlorite than in whole rocks indicates that chlorite is the primary reservoir for Li in altered SCBs (Supplementary Figure S2C). The partition coefficient of Li between chlorite and fluid
Origin of Low δ7Li Values in South China Basalts
The altered SCBs, especially the FK basalts, have lower δ7Li values than the fresh SCBs, altered oceanic crust (AOC), and MORB (Figures 3A,B). It has been unequivocally demonstrated that Li isotopic fractionation is insignificant at magmatic temperatures (Tomascak et al., 1999; Jeffcoate et al., 2007), except possibly during the latest-, fluid-rich stages of evolution (Ellis et al., 2018; Neukampf et al., 2019, 2020). Additionally, the δ7Li values of fresh SCBs are similar to those of OIB and MORB, ruling out the possibility that the light δ7Li values of altered SCBs were derived from the EM2 mantle reservoir (Figure 3B). Assimilation of continental crustal rocks during emplacement of magma would make it impossible to achieve such low δ7Li values for the altered SCBs, due to their higher content of Li and lower δ7Li values than UCC (Figure 3B).
Hydrothermally altered basalts (HABs) from Iceland display very little variation in δ7Li values controlled by chlorite (Figure 3B), suggesting that hydrothermal alteration merely involved with seawater or meteoric water did not induce any significant changes in δ7Li values of whole rock. Additionally, the higher [Li] and relatively lower δ7Li values in altered SCBs than HAB suggest that such metasomatic fluid was different from seawater or meteoric water and was capable of increasing the basalts’ [Li] and decreasing their δ7Li values. In combination with the increase in [Li] during alteration, it appears likely that a crustal fluid with a low δ7Li value, similar to the fluid exsolving from the Variscan granites of Southwest England reported above (−1.4∼+0.9‰ from Richard et al., 2018), was found, thereby enriching their [Li] and lowering their δ7Li values. Although both the QYS and the FK basalts underwent hydrothermal alteration (Figure 1B), the FK basalts have lower content of U, Ba, and K than QYS basalts (Figure 2C). In contrast to the δ7Li values of most QYS basalts (0.3–0.5), the FK basalts have even lower δ7Li values (−4.3∼−1.8) (Figure 3B). These differences between the QYS and FK basalts suggest that there must have been differences in the low-temperature processes.
The FK basalts were sampled from the riverbed, where the dominant hydrothermal mineral is chlorite and minor weathered clays, such as kaolinite and illite (Supplementary Table S1), were found, suggesting that they underwent both hydrothermal alteration and slight weathering. Additionally, the [Li] and its isotope composition of FK basalts fall between fresh SCBs and weathered diabase dyke (WDD) (Figure 3B), suggesting weathering has occurred in FK basalts. Eliminating the effect of other volatile-rich minerals on LOI, the fitted function between LOI and δ7Li of fresh and weathered basalts imply that the clay minerals modified the δ7Li of weathered rocks (Figure 3A). The good correlation between δ7Li and the content of chlorite supports that it is chlorite that controlled the Li isotopic composition of the FK basalts (Figure 3C). The lower δ7Li values in FK basalts than in fresh basalts can, thus, be explained by the preferential incorporation of 6Li over 7Li in chlorite’s octahedral site during weathering, which is corroborated by extremely large Li isotopic fractionation between clays and fluid (Δ7Li∼20°Cclays-solution up to -23‰) found in abundant experimental studies of weathering (Wunder et al., 2006; Millot et al., 2010; Hindshaw et al., 2019; Pogge von Strandmann et al., 2019), calculation model (Fairén et al., 2015), and natural weathered basaltic rocks (δ7Li as low as -20.2‰; Rudnick et al., 2004). The δ7Li value (0.02‰) of FK basalts before weathering can be estimated by fitted function when the proportion of chlorites is zero, corresponding to an inactivated fractionation effect of chlorite (Figure 3C). At any given [Li], the FK basalts and the WDD have lower δ7Li values than the AOC (Figures 3A,B), suggesting that continental weathering had a stronger effect in lowering the δ7Li values of basaltic rocks than low-T alteration on the ocean floor.
Hydrothermal Alteration and Weathering of the South China Basalts
It appears that chlorite served as an “element pool” for imposing restrictions on the mobility of Li during the hydrothermal alteration. Such process set the stage for subsequent weathering to effect even lower δ7Li values in weathered basalts. In our model, the magmatic fluids from the Variscan granites of Southwest England are selected to represent the hydrothermal fluids which metasomatized the fresh SCBs. The [Li] and δ7Li values of the QYS basalts were, thus, modeled as mixtures of fresh SCBs and metasomatic fluids which is consistent with the presence of abundant epidote and high LOI in QYS basalts (Figure 3A). According to this model, the QYS basalts correspond to a mixture of fresh SCBs with 20–40% metasomatic fluids, while the FK basalts appear to have experienced lower degrees of metasomatism (4–15%). The overestimated fluid addition of QYS basalts can be attributed to two aspects: 1) crustal contamination increases the [Li] in QYS basalts; 2) the [Li] of real metasomatic fluid is underestimated as evidenced by the high [Li] in chlorite (299.0–545.6 ppm) and low Dchlorite-fluid (∼0.8 at 150°C).
The interaction of FK basalts with subaerial water during weathering was modeled by Rayleigh distillation in an open system (Rudnick et al., 2004; Liu et al., 2013) superimposed on previously hydrothermally altered basalts. Using the following equations allows obtaining the [Li] (Csolid) and δ7Lisolid values of weathered rocks:
where C0 and δ7Li0 are the [Li] and δ7Li values of hydrothermally altered basalts, F is the fraction of riverine water relative to the solid, f is the fraction of Li remaining in the weathered rocks, and D and α are the bulk partition coefficient and fractionation factor. Berger et al. (1988) reported that
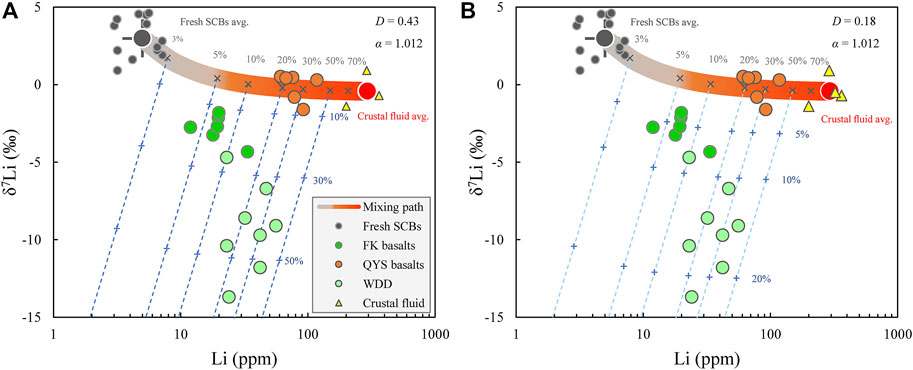
FIGURE 4. Conceptual model for the genesis of QYS and FK basalts. The two end members of the mixing path as hydrothermal alteration are the average fresh SCBs (Li: 5.0 ± 1.5 ppm; δ7Li: 3.0 ± 1.2‰, n = 13, 1 σ) and the average magmatic-hydrothermal fluid from the Variscan granites, Southwest England (Li: 294 ± 60 ppm; δ7Li: 0.43 ± 0.8‰, n = 4, 1 σ) (Richard et al., 2018). The dashed blue lines represent weathering, modeled using Rayleigh distillation, and was applied to hydrothermally altered basalts using Eqs 1, 2 with α = 1.012, and D = 0.18 (A) and D = 0.42 (B). The numbers next to the dashed blue lines in (A) and (B) are the fraction of riverine water relative to the weathering rocks, which represent the weathering degree of rocks.
Implication for the Origin of Lower δ7Li Values in Continental-Type Eclogites
The weathered basaltic rocks have the trend of higher [Li] and lower δ7Li values than the fresh basalts and altered oceanic crust (AOC) (Figure 3B). Coincidentally, such feature agrees with the overarching goal of increasing [Li] and decreasing δ7Li in orogenic eclogites from previous studies. For example, the light δ7Li values (−12.9 ∼ +4.6) of oceanic-type eclogites are generally ascribed to processes operating on the AOC during subduction and exhumation, such as dehydration (Zack et al., 2003), metasomatism (Simons et al., 2010), and diffusion of Li between country rocks/fluids and subducting slabs (Marschall et al., 2007). By contrast, continental-type eclogites have lighter and more scattered δ7Li values (−21.9∼+5.4) (Supplementary Figure S4). Most crustal fluids interacting with orogenic eclogites generally have heavy Li isotopic compositions (Simons et al., 2010), while fluid activity in continental collision zones is generally deficient and episodic compared to that in oceanic subduction zones (Zheng and Chen 2016), so crustal fluid metasomatism alone is unlikely to be at the origin of the low δ7Li values of continental-type eclogites. Although diffusion of Li from surrounding rocks or fluids into eclogites could contribute to the Li isotopic signature of continental eclogites (Marschall et al., 2007), the contribution from weathered protoliths of continental eclogites should not be overlooked. Zhang et al. (2016) found metamorphic zircons in continental-type eclogites from North Qaidam yielding δ18O values higher than those of relict magmatic zircons, which was ascribed to low-T altered protoliths. This low-T process is known to form secondary minerals such as chlorite in continental basalts. Furthermore, chlorite is a common mineral in the subduction zone and an equally important reservoir for Li akin to phengite, possibly keeping Li concentration and isotopic ratios unchanged during prograde metamorphism (Bebout 2014; Barnes et al., 2019). Therefore, weathering must be taken into consideration when trying to understand the origin of extremely low δ7Li values of continental-type eclogites.
Conclusion
Compared to fresh SCBs, the higher Li concentration in altered SCBs from the Qingyunshan (QYS) and the Fankeng (FK) formations originated from their hydrothermal alteration by magmatic fluids having high [Li]. This magmatic-hydrothermal alteration also recrystallized primary igneous ferromagnesian silicate minerals to chlorite, which is the main repository for Li in the altered SCBs and, thus, controls their Li isotopic compositions. Given that chlorite preferentially incorporates 6Li over 7Li, this magmatic-hydrothermal alteration lowered the rocks’ δ7Li values. Subsequent weathering of the chlorite-bearing FK basalts further lowered their δ7Li values, in contrast to the hydrothermally altered QYS basalts. In contrast to previous studies, the weathered basalts, thus, acquired δ7Li values lower than those of altered oceanic crust, and it becomes clear that continental weathering needs to be considered instead of ocean floor alteration when investigating the origin of low δ7Li values in continental-type eclogites.
Data Availability Statement
The original contributions presented in the study are included in the article/Supplementary Material, further inquiries can be directed to the corresponding author.
Author Contributions
C-LY: conceptualization, data curation, dormal analysis, funding acquisition, investigation, methodology, visualization, and writing—original draft. YX: conceptualization, investigation, methodology, and writing—review and editing. Y-YW: investigation and writing—review and editing. ON: writing—review and editing. HS: writing—review and editing. H-QW: writing—review and editing. D-YL: writing—review and editing. X-XW: writing—review and editing. D-BT: writing—review and editing.
Funding
This study was supported by the National Natural Science Foundation of China (42073003), National Key R&D Program of China (2018YFA0702701) and the Strategic Priority Research Program (B) of the Chinese Academy of Sciences (XDB41000000).
Conflict of Interest
The authors declare that the research was conducted in the absence of any commercial or financial relationships that could be construed as a potential conflict of interest.
Publisher’s Note
All claims expressed in this article are solely those of the authors and do not necessarily represent those of their affiliated organizations, or those of the publisher, the editors, and the reviewers. Any product that may be evaluated in this article, or claim that may be made by its manufacturer, is not guaranteed or endorsed by the publisher.
Acknowledgments
We thank Zhen-Hui Hou and Feng-Tai Tong for their help during geological analyses. We thank associate editor Jean-Louis Vigneresse and reviewers of Kwan-Nang Pang and Michael Rowe for their thorough and helpful reviews and suggestions.
Supplementary Material
The Supplementary Material for this article can be found online at: https://www.frontiersin.org/articles/10.3389/feart.2022.844353/full#supplementary-material
References
Barnes, J. D., Penniston-Dorland, S. C., Bebout, G. E., Hoover, W., Beaudoin, G. M., and Agard, P. (2019). Chlorine and Lithium Behavior in Metasedimentary Rocks during Prograde Metamorphism: A Comparative Study of Exhumed Subduction Complexes (Catalina Schist and Schistes Lustrés). Lithos 336-337, 40–53. doi:10.1016/j.lithos.2019.03.028
Beaufort, D., Rigault, C., Billon, S., Billault, V., Inoue, A., Inoué, S., et al. (2015). Chlorite and Chloritization Processes through Mixed-Layer mineral Series in Low-Temperature Geological Systems–A Review. Clay minerals 50, 497–523. doi:10.1180/claymin.2015.050.4.06
Bebout, G. E. (2014). “Chemical and Isotopic Cycling in Subduction Zones,” in Treatise on Geochemistry. Second Edition (Elsevier), 703–747. doi:10.1016/B978-0-08-095975-7.00322-3
Berger, G., Schott, J., and Guy, C. (1988). Behavior of Li, Rb and Cs during basalt Glass and Olivine Dissolution and Chlorite, Smectite and Zeolite Precipitation from Seawater: Experimental Investigations and Modelization between 50° and 300°C. Chem. Geology. 71, 297–312. doi:10.1016/0009-2541(88)90056-3
Bird, D. K., and Spieler, A. R. (2004). Epidote in Geothermal Systems. Rev. Mineralogy Geochem. 56, 235–300. doi:10.2138/gsrmg.56.1.235
Cen, T., Li, W.-x., Wang, X.-c., Pang, C.-j., Li, Z.-x., Xing, G.-f., et al. (2016). Petrogenesis of Early Jurassic Basalts in Southern Jiangxi Province, South China: Implications for the thermal State of the Mesozoic Mantle beneath South China. Lithos 256-257, 311–330. doi:10.1016/j.lithos.2016.03.022
Chan, L.-H., Alt, J. C., and Teagle, D. A. H. (2002). Lithium and Lithium Isotope Profiles through the Upper Oceanic Crust: a Study of Seawater-basalt Exchange at ODP Sites 504B and 896A. Earth Planet. Sci. Lett. 201, 187–201. doi:10.1016/s0012-821x(02)00707-0
Chen, C.-H., Lee, C.-Y., and Shinjo, R. (2008). Was There Jurassic Paleo-Pacific Subduction in South China?: Constraints from 40Ar/39Ar Dating, Elemental and Sr-Nd-Pb Isotopic Geochemistry of the Mesozoic Basalts. Lithos 106, 83–92. doi:10.1016/j.lithos.2008.06.009
Coogan, L. A., Gillis, K. M., Pope, M., and Spence, J. (2017). The Role of Low-Temperature (off-axis) Alteration of the Oceanic Crust in the Global Li-Cycle: Insights from the Troodos Ophiolite. Geochimica et Cosmochimica Acta 203, 201–215. doi:10.1016/j.gca.2017.01.002
El Korh, A., Deloule, E., Luais, B., Boiron, M.-C., Bastian, L., and Vigier, N. (2019). Lithium Behaviour and Isotope Fractionation During Fluid-Rock Interactions in Variscan Oceanic Suture Zones: Limousin Ophiolite and Ile de Groix High-pressure Terrane (France). J. Pet. 60, 1963–1990. doi:10.1093/petrology/egz060
Ellis, B. S., Szymanowski, D., Magna, T., Neukampf, J., Dohmen, R., Bachmann, O., et al. (2018). Post-eruptive Mobility of Lithium in Volcanic Rocks. Nat. Commun. 9, 3228–3229. doi:10.1038/s41467-018-05688-2
Fairén, A. G., Losa-Adams, E., Gil-Lozano, C., Gago-Duport, L., Uceda, E. R., Squyres, S. W., et al. (2015). Tracking the Weathering of Basalts on M Ars Using Lithium Isotope Fractionation Models. Geochem. Geophys. Geosyst. 16, 1172–1197. doi:10.1002/2015gc005748
Gao, Y., and Casey, J. F. (2012). Lithium Isotope Composition of Ultramafic Geological Reference Materials JP-1 and DTS-2. Geostandards Geoanalytical Res. 36, 75–81. doi:10.1111/j.1751-908x.2011.00117.x
Gao, Y., Vils, F., Cooper, K. M., Banerjee, N., Harris, M., Hoefs, J., et al. (2012). Downhole Variation of Lithium and Oxygen Isotopic Compositions of Oceanic Crust at East Pacific Rise, ODP Site 1256. Geochem. Geophys. Geosystems 13, Q10001. doi:10.1029/2012gc004207
Gysi, A. P., and Stefánsson, A. (2012). Mineralogical Aspects of CO2 Sequestration during Hydrothermal basalt Alteration - an Experimental Study at 75 to 250°C and Elevated pCO2. Chem. Geology. 306-307, 146–159. doi:10.1016/j.chemgeo.2012.03.006
Halama, R., John, T., Herms, P., Hauff, F., and Schenk, V. (2011). A Stable (Li, O) and Radiogenic (Sr, Nd) Isotope Perspective on Metasomatic Processes in a Subducting Slab. Chem. Geology. 281, 151–166. doi:10.1016/j.chemgeo.2010.12.001
Hindshaw, R. S., Tosca, R., Goût, T. L., Farnan, I., Tosca, N. J., and Tipper, E. T. (2019). Experimental Constraints on Li Isotope Fractionation during clay Formation. Geochimica et Cosmochimica Acta 250, 219–237. doi:10.1016/j.gca.2019.02.015
Ho, K.-S., Chen, J.-c., Lo, C.-H., and Zhao, H.-l. (2003). 40Ar-39Ar Dating and Geochemical Characteristics of Late Cenozoic Basaltic Rocks from the Zhejiang-Fujian Region, SE China: Eruption Ages, Magma Evolution and Petrogenesis. Chem. Geology. 197, 287–318. doi:10.1016/s0009-2541(02)00399-6
Hou, Z., Xiao, Y., Shen, J., and Yu, C. (2020). In Situ rutile U-Pb Dating Based on Zircon Calibration Using LA-ICP-MS, Geological Applications in the Dabie Orogen, China. J. Asian Earth Sci. 192, 104261. doi:10.1016/j.jseaes.2020.104261
Jeffcoate, A. B., Elliott, T., Kasemann, S. A., Ionov, D., Cooper, K., and Brooker, R. (2007). Li Isotope Fractionation in Peridotites and Mafic Melts. Geochimica et Cosmochimica Acta 71, 202–218. doi:10.1016/j.gca.2006.06.1611
Kısakürek, B., Widdowson, M., and James, R. H. (2004). Behaviour of Li Isotopes during continental Weathering: the Bidar Laterite Profile, India. Chem. Geology. 212, 27–44. doi:10.1016/j.chemgeo.2004.08.027
Krienitz, M.-S., Garbe-Schönberg, C.-D., Romer, R. L., Meixner, A., Haase, K. M., and Stroncik, N. A. (2012). Lithium Isotope Variations in Ocean Island Basalts-Implications for the Development of Mantle Heterogeneity. J. Pet. 53, 2333–2347. doi:10.1093/petrology/egs052
Li, Z.-X., and Li, X.-H. (2007). Formation of the 1300-km-wide Intracontinental Orogen and Postorogenic Magmatic Province in Mesozoic South China: A Flat-Slab Subduction Model. Geology 35, 179. doi:10.1130/g23193a.1
Li, Z., Qiu, J.-S., and Yang, X.-M. (2014). A Review of the Geochronology and Geochemistry of Late Yanshanian (Cretaceous) Plutons along the Fujian Coastal Area of southeastern China: Implications for Magma Evolution Related to Slab Break-Off and Rollback in the Cretaceous. Earth-Science Rev. 128, 232–248. doi:10.1016/j.earscirev.2013.09.007
Li, Z., Guo, J., Dong, Z., and Chen, J. (2018). Insight into Interactions of Olivine-scCO2-Water System at 140 °C and 15 MPa during CO2 mineral Sequestration. Geosci. Front. 9, 1945–1955. doi:10.1016/j.gsf.2017.12.008
Liu, X.-M., Rudnick, R. L., McDonough, W. F., and Cummings, M. L. (2013). Influence of Chemical Weathering on the Composition of the continental Crust: Insights from Li and Nd Isotopes in bauxite Profiles Developed on Columbia River Basalts. Geochimica et Cosmochimica Acta 115, 73–91. doi:10.1016/j.gca.2013.03.043
Liu, H., Sun, H., Xiao, Y., Wang, Y., Zeng, L., Li, W., et al. (2019). Lithium Isotope Systematics of the Sumdo Eclogite, Tibet: Tracing Fluid/rock Interaction of Subducted Low-T Altered Oceanic Crust. Geochimica et Cosmochimica Acta 246, 385–405. doi:10.1016/j.gca.2018.12.002
Marschall, H. R., Pogge von Strandmann, P. A. E., Seitz, H.-M., Elliott, T., and Niu, Y. (2007). The Lithium Isotopic Composition of Orogenic Eclogites and Deep Subducted Slabs. Earth Planet. Sci. Lett. 262, 563–580. doi:10.1016/j.epsl.2007.08.005
Marschall, H. R., Wanless, V. D., Shimizu, N., von Strandmann, P. A. P., Elliott, T., and Monteleone, B. D. (2017). The Boron and Lithium Isotopic Composition of Mid-Ocean Ridge Basalts and the Mantle. Geochim. Cosmochim. Acta 207, 102–138. doi:10.1016/j.gca.2017.03.028
McDonough, W. F., and Sun, S.-S. (1995). The Composition of the Earth. Chem. Geology. 120, 223–253. doi:10.1016/0009-2541(94)00140-4
Meng, L., Li, Z.-X., Chen, H., Li, X.-H., and Wang, X.-C. (2012). Geochronological and Geochemical Results from Mesozoic Basalts in Southern South China Block Support the Flat-Slab Subduction Model. Lithos 132-133, 127–140. doi:10.1016/j.lithos.2011.11.022
Millot, R., Guerrot, C., Vigier, N., and Research, G. (2004). Accurate and High-Precision Measurement of Lithium Isotopes in Two Reference Materials by MC-ICP-MS. Geostand Geoanalyt Res. 28, 153–159. doi:10.1111/j.1751-908x.2004.tb01052.x
Millot, R., Scaillet, B., and Sanjuan, B. (2010). Lithium Isotopes in Island Arc Geothermal Systems: Guadeloupe, Martinique (French West Indies) and Experimental Approach. Geochimica et Cosmochimica Acta 74, 1852–1871. doi:10.1016/j.gca.2009.12.007
Neukampf, J., Ellis, B. S., Laurent, O., Steinmann, L. K., Ubide, T., Oeser, M., et al. (2020). Time Scales of Syneruptive Volatile Loss in Silicic Magmas Quantified by Li Isotopes. Geology 49, 125–129. doi:10.1130/g47764.1
Neukampf, J., Ellis, B. S., Magna, T., Laurent, O., and Bachmann, O. (2019). Partitioning and Isotopic Fractionation of Lithium in mineral Phases of Hot, Dry Rhyolites: The Case of the Mesa Falls Tuff, Yellowstone. Chem. Geology. 506, 175–186. doi:10.1016/j.chemgeo.2018.12.031
Penniston-Dorland, S. C., Sorensen, S. S., Ash, R. D., and Khadke, S. V. (2010). Lithium Isotopes as a Tracer of Fluids in a Subduction Zone Mélange: Franciscan Complex, CA. Earth Planet. Sci. Lett. 292, 181–190. doi:10.1016/j.epsl.2010.01.034
Penniston-Dorland, S., Liu, X.-M., and Rudnick, R. L. (2017). Lithium Isotope Geochemistry. Rev. Mineralogy Geochem. 82, 165–217. doi:10.2138/rmg.2017.82.6
Pogge von Strandmann, P. A. E., Fraser, W. T., Hammond, S. J., Tarbuck, G., Wood, I. G., Oelkers, E. H., et al. (2019). Experimental Determination of Li Isotope Behaviour during basalt Weathering. Chem. Geology. 517, 34–43. doi:10.1016/j.chemgeo.2019.04.020
Richard, A., Banks, D. A., Hendriksson, N., and Lahaye, Y. (2018). Lithium Isotopes in Fluid Inclusions as Tracers of Crustal Fluids: An Exploratory Study. J. Geochemical Exploration 184, 158–166. doi:10.1016/j.gexplo.2017.10.017
Rudnick, R., and Gao, S. (2014). Composition of the Continental Crust in Treatise on Geochemistry Editors-In-Chief: Heinrich Holland and Karl Turekian. doi:10.1016/B978-0-08-095975-7.00301-6
Rudnick, R. L., Tomascak, P. B., Njo, H. B., and Gardner, L. R. (2004). Extreme Lithium Isotopic Fractionation during continental Weathering Revealed in Saprolites from South Carolina. Chem. Geology. 212, 45–57. doi:10.1016/j.chemgeo.2004.08.008
Ryan, J. G., and Kyle, P. R. (2004). Lithium Abundance and Lithium Isotope Variations in Mantle Sources: Insights from Intraplate Volcanic Rocks from Ross Island and Marie Byrd Land (Antarctica) and Other Oceanic Islands. Chem. Geology. 212, 125–142. doi:10.1016/j.chemgeo.2004.08.006
Schmidt, M. W., and Poli, S. (2014). Devolatilization during Subduction. Treatise Geochem. 4, 669–701. doi:10.1016/b978-0-08-095975-7.00321-1
Simons, K. K., Harlow, G. E., Brueckner, H. K., Goldstein, S. L., Sorensen, S. S., Hemming, N. G., et al. (2010). Lithium Isotopes in Guatemalan and Franciscan HP-LT Rocks: Insights into the Role of Sediment-Derived Fluids during Subduction. Geochimica et Cosmochimica Acta 74, 3621–3641. doi:10.1016/j.gca.2010.02.033
Staudigel, H. (2014). “Chemical Fluxes from Hydrothermal Alteration of the Oceanic Crust,” in Treatise on Geochemistry. Editors H. D. Holland, and K. K. Turekian (2nd Edn) 4 583–606 doi:10.1016/b978-0-08-095975-7.00318-1
Sun, H., Gao, Y., Xiao, Y., Gu, H.-O., and Casey, J. F. (2016). Lithium Isotope Fractionation during Incongruent Melting: Constraints from post-collisional Leucogranite and Residual Enclaves from Bengbu Uplift, China. Chem. Geology. 439, 71–82. doi:10.1016/j.chemgeo.2016.06.004
Sun, S.-S., and McDonough, W. F. (1989). Chemical and Isotopic Systematics of Oceanic Basalts: Implications for Mantle Composition and Processes. Geol. Soc. Lond. Spec. Publications 42, 313–345. doi:10.1144/gsl.sp.1989.042.01.19
Teng, F.-Z., Li, W.-Y., Rudnick, R. L., and Gardner, L. R. (2010). Contrasting Lithium and Magnesium Isotope Fractionation during continental Weathering. Earth Planet. Sci. Lett. 300, 63–71. doi:10.1016/j.epsl.2010.09.036
Teng, F.-Z., Rudnick, R. L., McDonough, W. F., Gao, S., Tomascak, P. B., and Liu, Y. (2008). Lithium Isotopic Composition and Concentration of the Deep continental Crust. Chem. Geology. 255, 47–59. doi:10.1016/j.chemgeo.2008.06.009
Tomascak, P. B., Tera, F., Helz, R. T., and Walker, R. J. (1999). The Absence of Lithium Isotope Fractionation during basalt Differentiation: New Measurements by Multicollector Sector ICP-MS. Geochimica et Cosmochimica Acta 63, 907–910. doi:10.1016/s0016-7037(98)00318-4
Tomascak, P. B., Magna, T., and Dohmen, R. (2016). Advances in Lithium Isotope Geochemistry. Springer. Available from: https://rd.springer.com/book/10.1007%2F978-3-319-01430-2.
Tong, F. T., Xiao, Y., Sun, H., Wang, Y., Wan, H., Gou, L.-F., et al. (2021). Lithium Isotopic Features of Quaternary Basaltic Saprolite, Zhanjiang, China: Atmospheric Input and clay-mineral Adsorption. Sci. Total Environ. 785, 147235. doi:10.1016/j.scitotenv.2021.147235
Verney-Carron, A., Vigier, N., Millot, R., and Hardarson, B. S. (2015). Lithium Isotopes in Hydrothermally Altered Basalts from Hengill (SW Iceland). Earth Planet. Sci. Lett. 411, 62–71. doi:10.1016/j.epsl.2014.11.047
Vigier, N., Decarreau, A., Millot, R., Carignan, J., Petit, S., and France-Lanord, C. (2008). Quantifying Li Isotope Fractionation during Smectite Formation and Implications for the Li Cycle. Geochimica et Cosmochimica Acta 72, 780–792. doi:10.1016/j.gca.2007.11.011
Wunder, B., Meixner, A., Romer, R. L., and Heinrich, W. (2006). Temperature-dependent Isotopic Fractionation of Lithium between Clinopyroxene and High-Pressure Hydrous Fluids. Contrib. Mineral. Petrol. 151, 112–120. doi:10.1007/s00410-005-0049-0
Xiao, B., and Chen, H. (2020). Elemental Behavior during Chlorite Alteration: New Insights from a Combined EMPA and LA-ICPMS Study in Porphyry Cu Systems. Chem. Geology. 543, 119604 doi:10.1016/j.chemgeo.2020.119604
Xiao, Y., Hoefs, J., Hou, Z., Simon, K., and Zhang, Z. (2011). Fluid/rock Interaction and Mass Transfer in continental Subduction Zones: Constraints from Trace Elements and Isotopes (Li, B, O, Sr, Nd, Pb) in UHP Rocks from the Chinese Continental Scientific Drilling Program, Sulu, East China. Contrib. Mineral. Petrol. 162, 797–819. doi:10.1007/s00410-011-0625-4
Yavuz, F., Kumral, M., Karakaya, N., Karakaya, M. Ç., and Yıldırım, D. K. (2015). A Windows Program for Chlorite Calculation and Classification. Comput. Geosciences 81, 101–113. doi:10.1016/j.cageo.2015.04.011
Zack, T., Tomascak, P. B., Rudnick, R. L., Dalpé, C., and McDonough, W. F. (2003). Extremely Light Li in Orogenic Eclogites: the Role of Isotope Fractionation during Dehydration in Subducted Oceanic Crust. Earth Planet. Sci. Lett. 208, 279–290. doi:10.1016/s0012-821x(03)00035-9
Zeng, G., He, Z. Y., Li, Z., Xu, X. S., and Chen, L. H. (2016). Geodynamics of Paleo‐Pacific Plate Subduction Constrained by the Source Lithologies of Late Mesozoic Basalts in southeastern China. Geophys. Res. Lett. 43 (10), 189–110. doi:10.1002/2016gl070346
Zhang, L., Chen, R.-X., Zheng, Y.-F., Li, W.-C., Hu, Z., Yang, Y., et al. (2016). The Tectonic Transition from Oceanic Subduction to continental Subduction: Zirconological Constraints from Two Types of Eclogites in the North Qaidam Orogen, Northern Tibet. Lithos 244, 122–139. doi:10.1016/j.lithos.2015.12.003
Zheng, Y.-F., and Chen, Y.-X. (2016). Continental versus Oceanic Subduction Zones. Natl. Sci. Rev. 3, 495–519. doi:10.1093/nsr/nww049
Zhou, J., Jiang, S., Wang, X., Yang, J., and Zhang, M. (2005). Re-Os Isochron Age of Fankeng Basalts from Fujian of SE China and its Geological Significance. Geochem. J. 39, 497–502. doi:10.2343/geochemj.39.497
Keywords: Li isotopes, chlorite, weathering, altered basalts, eclogites
Citation: Yu C-, Xiao Y, Wang Y-, Nadeau O, Sun H, Wan H-, Li D-, Wang X- and Tan D- (2022) Lithium Isotopic Compositions of Mesozoic and Cenozoic Basalts From South-Eastern China: Implications for Extremely Low δ7Li of Continental-Type Eclogites. Front. Earth Sci. 10:844353. doi: 10.3389/feart.2022.844353
Received: 28 December 2021; Accepted: 24 February 2022;
Published: 17 March 2022.
Edited by:
Jean-Louis Vigneresse, Université de Lorraine, FranceReviewed by:
Kwan-Nang Pang, Academia Sinica, TaiwanMichael Rowe, The University of Auckland, New Zealand
Copyright © 2022 Yu, Xiao, Wang, Nadeau, Sun, Wan, Li, Wang and Tan. This is an open-access article distributed under the terms of the Creative Commons Attribution License (CC BY). The use, distribution or reproduction in other forums is permitted, provided the original author(s) and the copyright owner(s) are credited and that the original publication in this journal is cited, in accordance with accepted academic practice. No use, distribution or reproduction is permitted which does not comply with these terms.
*Correspondence: Yilin Xiao, eWx4aWFvQHVzdGMuZWR1LmNu