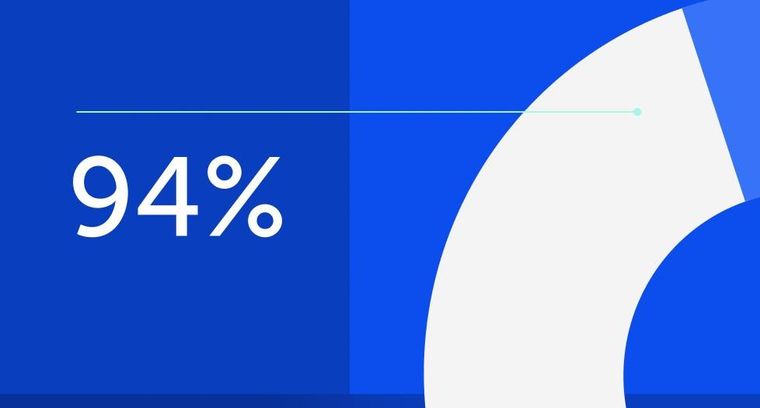
94% of researchers rate our articles as excellent or good
Learn more about the work of our research integrity team to safeguard the quality of each article we publish.
Find out more
BRIEF RESEARCH REPORT article
Front. Earth Sci., 08 April 2022
Sec. Volcanology
Volume 10 - 2022 | https://doi.org/10.3389/feart.2022.827252
This article is part of the Research TopicLarge Explosive Volcanic Eruptions (LEVEs)View all 5 articles
The Youngest Toba Tuff (YTT) supereruption from Toba Caldera in Sumatra at ca. 74,000 years BP is the largest volcanic event recorded in the Pleistocene. Intriguingly, recent radioisotopic dating of the near antipodal Los Chocoyos (LCY) supereruption from the Atitlán caldera in Guatemala finds an identical age within uncertainties to that of YTT. This opens the question of whether these synchronous supereruptions may be a coincidence or could be a consequence of each other? Using the known eruptive record from the past 2 Myr, we find that the likelihood of having two near antipodal supereruptions (>1,000 km3 tephra volume) within centuries (<400 years), as suggested by volcanic proxies and annual counting layer chronology in the ice core records, is very small (0.086%), requiring a non-random cause and effect. Considering this analysis, we speculate that one potential physical mechanism that could explain the temporal relationship between these supereruptions is that seismic energy released during YTT eruption focused on the antipodal region, where concentrated stresses ultimately promoted the eruption of the perched LCY magma system (or vice versa). This supereruption “double-whammy” may thus be the more compelling source of the significant environmental impacts often attributed individually to the YTT supereruption. Improving the existing age information of YTT and LCY, and a better understanding of caldera collapse events will enable further testing of the hypothesis that synchronous supereruptions do not result by pure chance.
Supereruptions are low-frequency but large-magnitude and unpredictable events capable of explosively ejecting ≥1 × 1015 kg (≥450 km3 dense rock equivalent, DRE) of silica-rich magma at geologically instantaneous timescales (magnitude (M) ≥ 8; Pyle, 2015). The recorded and expected disruptive impacts of such supereruptions range from local to global in scale (Miller and Wark, 2008; Self, 2015; Brenna et al., 2021) and may have devastating consequences to human civilization (Sparks et al., 2005). Moreover, their rarity hinders meaningful eruptions forecasting. Adding to this challenge is that whereas the trigger mechanisms of small-scale eruptions are relatively well understood (e.g., recharge, overpressure from magmatic volatile exsolution), what drives magmatic systems to generate supereruptions is still much debated (Wilson et al., 2021). It has been suggested that supereruptions and smaller-scale eruptions may be triggered by fundamentally different processes that influence their frequency and erupted magma volume (Mason et al., 2004; Deligne et al., 2010; Caricchi et al., 2014; de Silva and Gregg, 2014; Gregg et al., 2015). As such, supereruptions are extreme, enigmatic, and unpredictable natural “black swan” events that society needs to understand and anticipate (Aven, 2013).
In the last 2 Myr, 13 supereruptions are known globally (Crosweller et al., 2012) with an estimated recurrence interval of ca. 150 kyr, a timescale shorter than the frequency of large meteorite impacts (>0.6 Myr, ≥20 km crater diameter; Bland, 2005) that could have potentially similar environmental consequences (Rampino, 2002). If only the well-preserved eruption record of the last ca. 100 kyr is considered, a much shorter recurrence interval of ca. 17 kyr is obtained (Rougier et al., 2018). This is because established eruption databases are incomplete due to preservation biases (Crosweller et al., 2012), especially going back in geologic times. Thus, these recurrence intervals could be considered maxima, and a temporal coincidence of supereruptions is not a priori unlikely. Synchronous, paired or clustered, large (M7 to M8) eruptions have been proposed within various volcanic regions (e.g., de Silva et al., 2006; Gravley et al., 2007), but synchroneity of eruptions ≥ M8 on a global scale is hitherto unknown. The discovery of two apparently synchronous recent supereruptions, the ca. 74 ka Youngest Toba Tuff (YTT; ∼5,300 km3 DRE; Costa et al., 2014), Sumatra, and Los Chocoyos (LCY), Guatemala (∼730 km3 DRE; Kutterolf et al., 2016; Cisneros de León et al., 2021), has implications for the global record of supereruptions and warrants an evaluation of the randomness of paired eruptions at the colossal scale. This study contributes to this discussion and lays out future research directions.
The Toba caldera (100 × 30 km) in Sumatra and Atitlán caldera (18 × 12 km) in Guatemala are both the outcome of continental subduction-related magmatism (Rose et al., 1987; Chesner et al., 1991). These multi-cyclic calderas have produced large silicic eruptions from which the YTT and LCY stand out. The YTT supereruption represents the largest event in the Quaternary period, discharging more than 8,600 km3 tephra (M9.1; Costa et al., 2014; Figure 1A), whereas the LCY represents the largest eruption in the American continent in the past ∼100 kyr (∼1,220 km3; Cisneros de León et al., 2021). According to deep-sea tephra settling model estimations, the total duration of the YTT was ∼9–14 days, whereas the LCY lasted for ∼20–27 days (Ledbetter and Sparks, 1979). The tephra record for the YTT lacks evidence for an initial Plinian-style eruption but distal deposits were attributed to co-ignimbrite deposits generated in ring-caldera fractures (Chesner, 1998). In contrast, the LCY presents proximal and distal tephra fallout deposits at the base of its sequence with characteristics suggesting an ultra-Plinian initial eruption (>40 km high eruptive column) followed by thick non-welded ignimbrite deposits (Newhall et al., 1987; Rose et al., 1987). The YTT supereruption is considered to be the consequence of progressive thermal maturation of its magmatic system and surrounding crust by prolonged magma influx over timescales of hundreds of thousands to millions of years (Liu et al., 2021). Zircon geochronology from the LCY also points to the protracted accumulation of silicic magmas prior to eruption (>80 kyr; Cisneros de León et al., 2021). The generation of silicic magmas associated with explosive eruptions is usually attributed to extensive mineral fractionation and melt extraction/segregation from crystal-rich (mush) domains (Bachmann and Bergantz, 2008). The evidence of protracted zircon crystallization with diverse physicochemical signatures as well as variable glass compositions in the YTT magmas suggests mush-derived melt heterogeneity in an extensive upper crustal magma reservoir (Reid and Vazquez, 2017; Tierney et al., 2019).
FIGURE 1. Spatial and geochronological information for the YTT and LCY projected over climate and volcanic proxy signals from the northern and southern hemisphere ice core records. (A) Location map of the Toba (Sumatra) and Atitlán calderas (Guatemala), their respective antipodes (hexagons) and approximate tephra distribution. (B) Synchronization of the YTT and LCY radioisotopic ages (±1σ) with the NGRIP oxygen isotope and sulfate concentration records around the Greenland Interstadial 20 (GI-20) and the Greenland Stadial (GS-20) as well as the sulfur isotopic compositions from the EPICA Dronning Maud Land (EDML, Antarctica), and the EPICA dome C (EDC, Antarctica) ice core records (Crick et al., 2021). Dashed gray lines indicate the candidate sulfate anomalies for the YTT supereruption in the NGRIP and Antarctic ice cores (Supplementary Figure S1). We only consider that bipolar S spikes are candidates for an equatorial supereruption.
The potential release of significant amounts of sulfur gases during the YTT supereruption has been linked to a major global climatic downturn that may have challenged the survival of modern humans (Ambrose, 1998; Figure 1B). Although this hypothesis is debated (Oppenheimer, 2002; see Supplementary Material), new evidence is tilting the opinion back towards a significant environmental impact around the time of the YTT supereruption (Black et al., 2021; Osipov et al., 2021). The LCY supereruption has not been studied for direct climate proxies but modelling connotes a strong potential in forcing climate change, especially by previously unconsidered sulfur coupled to halogen loading to the atmosphere (Krüger et al., 2015; Kutterolf et al., 2015; Brenna et al., 2020; Brenna et al., 2021).
The exact location of the SO42− spike related to the YTT in ice cores remains highly ambiguous due to the lack of volcanic glass shards in both northern and southern hemisphere archives (Oppenheimer, 2002; Robock et al., 2009; Williams, 2012). Nevertheless, a prominent sulfate anomaly occurring in bipolar ice-core records has been tentatively correlated to the YTT (e.g., T2 sulfate spike, Figure 1B and Supplementary Figure S1; Svensson et al., 2013). However, eight other significant volcanic-derived sulfate anomalies from unknown sources (T1-T9; Figure 1B and Supplementary Figure S1; Svensson et al., 2013) also occur within uncertainty of the currently accepted radioisotopically determined eruption ages for the YTT between 73.9 ± 0.3 ka BP (1σ; 40Ar/39Ar in sanidine; Storey et al., 2012) and 75.0 ± 0.9 ka BP (1σ; 40Ar/39Ar in sanidine and biotite; Mark et al., 2014). An updated 40Ar/39Ar age for the YTT at 73.7 ± 0.3 ka (Mark et al., 2017) has been recently calculated by integrating the age data from Storey et al. (2012) and Mark et al. (2014) (two different laboratories) based on a new optimization model from Niespolo et al. (2017). Moreover, recent (U-Th)/He zircon dating of YTT has shown indistinguishable age results (75.7 ± 4.1 and 75.5 ± 4.7 ka, 1σ; Mucek et al., 2021) from those of 40Ar/39Ar ages, demonstrating the consistency of both dating methods, although (U-Th)/He zircon ages are less precise than 40Ar/39Ar ages.
A recent study indicates that the LCY supereruption (Cisneros de León et al., 2021) is a potential source for one of these significant sulfate spikes. The age of the LCY was initially estimated from δ18O stratigraphy at 84 ± 5 ka BP (Drexler et al., 1980) and remained radioisotopically untested for several decades due to the lack of sanidine and the ubiquitous presence of altered biotite that precluded reliable 40Ar/39Ar dating (Rose et al., 1999). However, recent radioisotopic dating applying (U-Th)/He in zircon has produced the first radiometric age for LCY of 74.8 ± 1.8 ka BP (1σ; Cisneros de León et al., 2021). This age is strikingly close to that of YTT (overlapping within 1σ error). Adding another dimension to these potential connections is the fact that Atitlán caldera is nearly antipodal (opposite side of Earth’s sphere) to Toba caldera (Figure 1A).
Based on the size and magnitude of the YTT and LCY supereruptions, significant deposition of sulfate on bipolar ice sheets could be expected, especially given their tropical vent locations (YTT = 35–3,500 Mt; Chesner and Luhr, 2010; Costa et al., 2014; LCY = 523 ± 94 Mt; Brenna et al., 2020). However, these sulfur masses are only minima due to the unknown sulfur mass that was partitioned into a fluid phase prior to the eruption and potentially vented during the onset of eruption. Thus, we propose that two of the nine sulfate signals are likely genetically related to the YTT and LCY (Figure 1B and Supplementary Figure S1). A potential relative time difference between the two supereruptions can be estimated by counting the ice-deposition annual layers (Svensson et al., 2013; Figure 1B). This yields a maximum of ca. 2,000 years (T1 to T9 spikes) and a minimum of ca. 25 years (T5 to T6 spikes; Svensson et al., 2013). We note that sulfate spikes T1 to T4 show relatively large-magnitude sulfur mass-independent fractionation (S-MIF) isotopic signatures (Figure 1B; Crick et al., 2021), which are indicative of large eruptions from tropical locations, the plumes of which reached altitudes at or above the ozone layer in the stratosphere. If only such spikes are considered, the potential timespan between the YTT and LCY can be further constrained to ca. 400 and 87 years, respectively; orders of magnitude shorter than the estimated recurrence interval of supereruptions (∼15–100 kyr). If we consider the two peaks with the largest sulfate and S-MIF signals in EPICA Dronning Maud Land (EDML; peaks T1 and T2) as derived from the LCY and YTT supereruptions, the relative time between the two eruptions is ∼87 ± 6 years. Because the amplitude of the sulfate peaks is variable across the different ice cores due to spatial variation in the deposition, the relative ordering differs slightly between different datasets (see Supplementary Figure S1 for other records). The temporal proximity of the YTT and LCY raises the question of whether there is a causal relationship between these two geologically concurrent events.
We evaluate whether the temporal clustering of large eruptions is purely random using the timing of >400 km3 bulk volume (>M7) Quaternary eruptions (LaMEVE; Crosweller et al., 2012) that produced well-preserved deposits in the geological record. We chose the >400 km3 threshold to increase the sample size to n = 28 for statistical analysis to avoid bias from having two coeval supereruptions (LCY and YTT) out of 13 in the past ca. 2 Myr (∼10%). To assess any temporal eruption clustering in the geological record spanning the last ca. 2 Myr we calculated the coefficient of variation value (CV: the ratio of the standard deviation and the mean value for the time between two successive volcanic eruptions) for the reported eruption record (n = 28). Furthermore, we used a Monte Carlo simulation to generate 50,000 different possible synthetic eruption histories after the reported eruption record and their 1σ uncertainties (for details see Supplementary Material).
We find that the median value of the CV is ∼1.035 indicating an approximately random distribution (Supplementary Figure S2) while the median value of the mean time between eruptions is 76.3 kyr (28 eruptions in 2.054 Myr). Using the CV values obtained from the synthetic sequences of n = 28, we find that our >400 km3 bulk volume LaMEVE distribution lies within the 5–95th percentile for a random distribution (inset Figure 2). Thus, the LaMEVE dataset does not display any significant non-randomness/clustering at the 95% confidence limit. This conclusion is enhanced by the clear difference in the CV value between the LaMEVE dataset and synthetic eruption histories with either periodically spaced eruptions or close eruption pairs (∼5% of the average time between eruption groups, Supplementary Figure S2). We describe additional results of our statistical analysis in the Supplementary Material.
FIGURE 2. Cumulative number of eruptions (>400 km3 bulk tephra volume) through the last ca. 2.2 Myr (LaMEVE). The color and size of the symbols represent the magnitude of the eruptions. Black bars through symbols are 1σ age uncertainties. The upper inset plot shows the probability density curves for the coefficient of variation (CV). Values of CV > 1 indicate clustering of eruptions and CV < 1 periodic eruptions. The lower inset histogram shows the number of eruption histories (among 50,000 synthetic eruptions histories assuming that eruptions are randomly distributed) that contain paired eruptions within 80–400 years and at least one supereruption (>1,000 km3) divided by the number of eruption pairs. A paired supereruption with YTT-LCY characteristics would be represented by “1.5” or “2.0” (either 1 or 2 eruption pairs) on the x-axis. On the other hand, if only one of the two closely spaced eruptions is a supereruption, it would be represented by the “0.5” or “1” (either 1 or 2 eruption pairs) bin in the x-axis. The numbers on each histogram show the percentage probability of being in that bin based on the synthetic eruptive histories.
Although our statistical analysis shows the randomness of large eruptions in the LaMEVE dataset as a whole, the probability of two supereruptions occurring within only 80–400 years may not be random by itself. Among 50,000 synthetic histories with random spacing between eruptions and volumes sampled from our LaMEVE dataset, we find that only 1.73% of the synthetic histories have an eruption pair that matches the YTT-LCY characteristics (Inset Figure 2). Moreover, even if we assume that the LaMEVE database is only complete for the last 100 kyr as suggested by Rougier et al. (2018) (6 eruptions with >400 km3 in last 100 kyr, recurrence time of ca. 17 kyr), there is still only a 4.2% probability of a YTT-LCY type eruption pair (Supplementary Figure S6). Thus, the statistical likelihood for two closely spaced supereruptions is small. In order to incorporate the near-antipodal nature of YTT-LCY in our statistical analysis, we assign, for each synthetic history, an eruption location randomly from amongst the events in the LaMEVE catalog (see Figure 3). We find a very low probability of 0.086% (for the full LaMEVE dataset time period, Supplementary Figure S7) when taking into account the synthetic eruption pairs with a time separation of 80–400 years and a <3,000 km distance between the antipodal location of the first eruption in the eruption pair and the second eruption’s location (comparable to Toba and Atitlán source calderas as shown in Figure 3).
FIGURE 3. Large volcanic eruptions (>400 km3 bulk tephra volume) from the LaMEVE database over the last 2.1 Myr showing vanishingly small chance of supereruptions occurring at antipodal locations. Eruption magnitudes (bulk tephra volume) are represented by the size and color of the symbol. The antipodal location for each eruption is shown as blue circles. It is noteworthy that the main potentially antipodal relationship between two supervolcano eruptions also close in time is the YTT and LCY eruption pair (∼2,200 km between the Atitlán caldera and the antipodal location of the Toba caldera). Note that Toba has sourced two Quaternary supereruptions, with YTT represented by the larger circle (id. Taupo).
Our statistical model demonstrates that the probability of quasi-synchronous supereruptions within millennial timescales at near antipodal locations is extremely low (0.086%), indicating that a “pure-chance” scenario as highly unlikely. A short recurrence interval between two supereruptions is unique, even considering present dating uncertainties (mainly due to the ±1.8 ka uncertainty for LCY). At present, it can only be speculated that a physical connection is strictly limited to dynamic far-field stresses since any static stress change associated with caldera collapse and eruptive venting will be restricted to the near-source region (Manga and Brodsky, 2006). In the absence of better constraints, we present a working hypothesis that accounts for the near antipodal location of Toba and Atitlán calderas and the known effects of antipodal seismic focusing, as explained in detail below. We present this as a challenge to the community hoping that future studies might shed light on this hypothesis.
Geological effects including extensive crustal fracturing and surface disruption have been reported in antipodal locations after major meteorite impacts on Mercury, Mars, Pluto, and the Moon resulting from spherical focusing of impact-generated seismic energy (Schultz and Gault, 1975; Watts et al., 1991; Williams and Greeley, 1994; Denton et al., 2021). On Mars, volcanism has been linked to the antipodal focusing of seismic energy after meteorite impacts by creating a deeply antipodal fractured region that acted as magma pathways into the surface (Williams and Greeley, 1994). The idea behind antipodal deformation following shock events is that spherical seismic waves propagate through the planet and focus on the antipode, producing enhanced near-surface stress gradients leading to deformation features (Hood and Artemieva, 2008; Bowling et al., 2013). On Earth, antipodal effects from meteorite impacts have been potentially associated with triggering or enhancing volcanic activity (Hagstrum, 2005; Meschede et al., 2011; Richards et al., 2015).
Over the past few decades, there have been several observations illustrating interactions (eruption or unrest) between nearby magmatic systems (<200 km; Linde and Sacks, 1998; Biggs et al., 2016). The primary proposed physical mechanism is the effect of evacuation of the magma reservoir on other magmatic systems through either direct elastic stress changes or poro-visco-elastic response of crustal and asthenospheric mush zones related to these other reservoirs (Gonnermann et al., 2012; Tarasewicz et al., 2012; Bégué et al., 2014; Walter et al., 2014; Sulpizio and Massaro, 2017). Large-magnitude tectonically-generated earthquakes have also been associated with antipodal seismic focusing (O’Malley et al., 2018). There is clear observational evidence of focusing of seismic surface waves near Atitlán caldera from large earthquakes in Sumatra (e.g., Dec 2004, M9.1 and April 2012, M8.7; Supplementary Figure S8) although this focusing is not exactly antipodal due to Earth’s internal heterogeneity (e.g, subduction zones, mid-ocean ridges). An estimate for the total elastic energy released during the YTT supereruption is on the order of 1019 [J] (Gudmundsson, 2014), which is the same order of magnitude as the largest instrumentally recorded earthquake, the M9.5 Valdivia (Chile) earthquake (Gudmundsson, 2016). The YTT seismic estimate should be considered an integrated estimate since the total energy released from the caldera collapse could have occurred over hours to days rather than an instantaneous rupture event (Stix and Kobayashi, 2008). However, it is noteworthy that there is potential for constructive wave interferences from a long-lived event (or multiple events) especially close to an antipodal location that may enhance the ground motion and local deformation (Supplementary Figure S8). However, the potential causal relationship between seismic energy released and initiation of volcanic eruptions hundreds of kilometers away remains poorly constrained (Hill et al., 2002; Seropian et al., 2021). It has been documented for only 0.4% of historical eruptions, though this probability may increase to 10% when considering a 2-year window between a leading large earthquake and a subsequent explosive eruption (Sawi and Manga, 2018). Causal effects are further supported by a temporal link between large magnitude earthquakes and volcanic activity at a global scale as proposed for the M9.1 Sumatra earthquake (Hill-Butler et al., 2020). The peak ground motion and dynamic stresses induced by passing seismic waves (particularly long-period surface waves) have been linked to the onset of multiple magmatic processes, affecting the host-rock, magma chamber, or associated hydrothermal system that could ultimately lead to an eruption (Davis et al., 2007; Seropian et al., 2021).
Large supereruption-feeding magma systems are thought to be buffered and “perched” at a critical threshold for extended periods and then externally triggered (de Silva and Gregg, 2014; Wilson et al., 2021). Long residence in a melt-present buffered state for both the YTT and LCY supervolcanic magmatic systems is suggested by their protracted zircon crystallization records. Notably, both the LCY and YTT exhibit strikingly similar thermochemical histories based on the crystallization ages of zircon (Figure 4A) which is sensitive to changes in magma chemistry and temperature. Magma accumulation timescales inferred from zircon ages are on the order of tens of thousands of years prior to both supereruptions, with a coincident maximum at ca. 96 ka. This suggests that the main phase of silicic magma differentiation and segregation of a melt-dominated magma body for YTT and LCY likely occurred within a similar time window of ca. 20 kyr before eruption. The maximum apparent rates of crystallization in magma bodies as indicated by zircon age maxima in the probability-density curve (Figure 4A) have been shown to occur over a wide range of timescales prior to eruption in different volcanic systems and are still not well understood (4–247 kyr; Simon et al., 2008; Wilson and Charlier, 2009). A rough correlation has been observed between older pre-eruption zircon age maxima (tens of kyr) and magmatic systems showing lower temperatures and higher water contents (Tierney et al., 2019). The exact significance of coincident zircon age spectra and crystallization maxima in YTT and LCY magmatic systems requires further investigation, yet enhanced zircon crystallization is usually preceded by significant recharge events (Klemetti and Clynne, 2014). However, if a coincident recharge event in both systems occurred shortly before the ca. 96 ka crystallization maxima, excursions in zircon trace elements (e.g., elevated Ti-in-zircon) are expected, which is presently unsupported. Consequently, whereas a similar temporal evolution based on the zircon ages is suggestive of synchronous magmatic evolution towards a critical state, the available data do not permit a non-unique interpretation.
FIGURE 4. (A) Ranked order plot and probability-density curves for the YTT and LCY zircon rim crystallization ages. Vertical bars represent radioisotopic ages for the YTT and LCY eruptions, with colored-bar thicknesses representing corresponding 1σ uncertainty. Data from 1Mucek et al. (2017), 2Storey et al. (2012), and 3Cisneros de León et al. (2021). (B) Potential YTT-eruption-induced seismic-triggering mechanisms influencing Atitlán caldera sub-volcanic system prior to the LCY supereruption. We propose that these processes may promote new migration pathways or induce instabilities in the magma reservoir and crust ultimately leading to eruption.
At present, the eruption order between YTT and LCY is unresolved given existing dating uncertainties. Nevertheless, we propose that the YTT preceding LCY is the more likely scenario since the larger YTT supereruption would have produced stronger seismic perturbations (Zürn and Widmer, 1996). The passage of long-period (tens of seconds) Rayleigh seismic waves generated during the YTT catastrophic eruption and caldera collapse through a crystal-mush-dominated LCY reservoir may have affected the system’s stability ultimately culminating in a supereruption on a decadal-century timescale (Figure 4B). At these seismic wave frequencies (10–20 s periods), the surface Rayleigh waves have peak depth sensitivities of ∼5–30 km (upper crust; O'Donnell et al., 2019) and thus are ideally suited to influence crustal magma reservoirs. We posit that the enhanced attenuation of the seismic surface waves in a granular mushy LCY magma reservoir could have increased the local melt permeability due to mush dilation, enabling rapid assembly of an eruptive magma body on decadal timescales (Winkler and Nur, 1982; O’Donovan et al., 2016).
Alternatively, the surface wave attenuation could have enhanced local pore pressure leading to small-scale fracturing/micro-seismicity, enhanced nucleation and growth of bubbles (Linde and Sacks, 1998; Hill et al., 2002; Nishimura, 2017), and overall promoting liquefaction (transformation of a solid where it behaves fluid dynamically as a liquid; Weinberg et al., 2021) in the crystalline mush (Müller et al., 2010; Sharma, 2012; Waymel et al., 2018; Zaccherini et al., 2020; Chapman et al., 2021; Wang et al., 2021; Yuan et al., 2021). Since mush domains behave as permeable materials, these spatio-temporal changes in melt and stress changes as well as crystal reorganization through decompaction, rotation/local shearing, or dissolution-reprecipitation of crystals lead to liquefaction and accumulation of eruptible magma (Léopoldès et al., 2013; Giacco et al., 2018; Brum et al., 2019; Dörfler and Kenkmann, 2020; Weinberg et al., 2021; Li et al., 2022). For the LCY system (or conversely YTT), the timescales necessary for the magmatic system to reach a critically perched state leading to a supereruption will depend on several parameters including crustal thermal state, magma reservoir(s) size and spatial distribution, volatile content, crustal stress state, and material properties in addition to magma composition. Nevertheless, since permeable melt flow is a slow process (compared to flow in open fractures), a lag in triggering a supereruption from another is to be expected. Thus, a decadal to a centennial time delay between the YTT and LCY eruption pair is physically plausible (Figure 4B). The reverse triggering of YTT by LCY would also be possible through similar physical effects of LCY associated long-period seismic waves.
Assuming that the seismic energy localization effect for the antipodal magma reservoir is valid, we posit that specific requirements still need to be fulfilled in order to trigger a (super)eruption doublet, including a sufficient number of magma bodies at a critical state and a sufficiently large magnitude of the sequence of volcanic-seismic events itself. This represents the “advance-clock” hypothesis wherein the seismic energy helped synchronize two eruptions that may still have occurred independently but at different times (Gomberg et al., 1997; Sawi and Manga, 2018). Failing to fulfill any of the above-mentioned requirements will preclude a correlated eruption. This scenario can also potentially explain why known supereruptions lack corresponding antipodal-triggered eruptions in the LaMEVE catalog (except YTT-LCY). Since there is significant uncertainty about the physical processes associated with the seismic source during a large caldera collapse eruption (e.g., instantaneous versus prolonged duration; efficiency of body versus surface wave generation), a detailed wave propagation model is beyond the scope of the present work. We can, however, outline one consequence of our proposal of supereruptions triggering other eruptions, namely that the YTT or LCY event may have also primed smaller volcanic systems, especially close to their respective locations. However, these less-significant eruptions are likely poorly preserved in the geologic record and/or remained unstudied. An exception could be the Arce tephra erupted from Coatepeque caldera in El Salvador, which produced two large silicic eruptions (∼26 and 41 km3 tephra volume) separated only by a couple of hundreds of years (Kutterolf et al., 2019) with an age of 72 ± 2 ka (Rose et al., 1999) that overlaps that of the YTT and LCY.
Resolving whether the time-space relationship between the YTT and LCY was not purely random but influenced by external factors would critically benefit from refining the absolute dating for both supereruptions (and other close supereruption pairs), preferentially by applying the same geochronological method, and from high-fidelity stratigraphic records where both eruptions could have deposited tephra. This also holds for assessing the climatic consequences of such paired supereruptions (Brenna et al., 2020; Black et al., 2021; Osipov et al., 2021) connoting that in combination both supereruptions would potentially have more impact on global climate than each eruption on its own (see further discussion in Supplementary Material). We think, however, that ultimately determining the precise time-lapse between the YTT and LCY would come from the identification of volcanic glass shards from both supereruptions within the ice-core layers or high-resolution paleoproxy records that could permit high-precision dating. We deem such an endeavor promising because glass compositions from the YTT and LCY tephra are unambiguously distinct in trace element abundances (Supplementary Figure S9).
Publicly available datasets were analyzed in this study. This data can be found here: https://www2.bgs.ac.uk/vogripa/searchVOGRIPA.cfc?method=searchForm.
Conceptualization AC, TM, SdeS, SS, AS, and SK; Methodology: TM and AC; Formal analysis: TM; Investigation: AC and TM; Visualization: AC and TM; Writing—original draft: AC, TM; Writing—review and editing: AC, TM, SdeS, SS, AS, and SK.
AC acknowledges funding support from the Deutsche Forschungsgemeinschaft (DFG) grants SCHM 2521/6-1 and KU 2685/7-1; TM acknowledges funding support from the Crosby Postdoc Fellowship at MIT; SdeS acknowledges support from National Science Foundation grant EAR 1551187 for studies of the Toba caldera.
The authors declare that the research was conducted in the absence of any commercial or financial relationships that could be construed as a potential conflict of interest.
All claims expressed in this article are solely those of the authors and do not necessarily represent those of their affiliated organizations, or those of the publisher, the editors and the reviewers. Any product that may be evaluated in this article, or claim that may be made by its manufacturer, is not guaranteed or endorsed by the publisher.
We thank editor Valerio Acocella for handling this manuscript. The differing opinions and perspectives of the reviewers have been crucial in challenging our thinking and for this, and we are very appreciative of journal reviews RC, TW and John Stix; not all who agree with our speculations herein. Nick Petford and Darren Mark are also thanked for their comments on an earlier version of this work.
The Supplementary Material for this article can be found online at: https://www.frontiersin.org/articles/10.3389/feart.2022.827252/full#supplementary-material
Ambrose, S. H. (1998). Late Pleistocene Human Population Bottlenecks, Volcanic winter, and Differentiation of Modern Humans. J. Hum. Evol. 34 (6), 623–651. doi:10.1006/jhev.1998.0219
Aven, T. (2013). On the Meaning of a Black Swan in a Risk Context. Saf. Sci. 57, 44–51. doi:10.1016/j.ssci.2013.01.016
Bachmann, O., and Bergantz, G. W. (2008). Rhyolites and Their Source Mushes across Tectonic Settings. J. Petrol. 49 (12), 2277–2285. doi:10.1093/petrology/egn068
Bégué, F., Deering, C. D., Gravley, D. M., Kennedy, B. M., Chambefort, I., Gualda, G. A. R., et al. (2014). Extraction, Storage and Eruption of Multiple Isolated Magma Batches in the Paired Mamaku and Ohakuri Eruption, Taupo Volcanic Zone, New Zealand. J. Petrol. 55 (8), 1653–1684. doi:10.1093/petrology/egu038
Biggs, J., Robertson, E., and Cashman, K. (2016). The Lateral Extent of Volcanic Interactions during Unrest and Eruption. Nat. Geosci. 9 (4), 308–311. doi:10.1038/ngeo2658
Black, B. A., Lamarque, J.-F., Marsh, D. R., Schmidt, A., and Bardeen, C. G. (2021). Global Climate Disruption and Regional Climate Shelters after the Toba Supereruption. Proc. Natl. Acad. Sci. 118 (29), e2013046118. doi:10.1073/pnas.2013046118
Bland, P. A. (2005). The Impact Rate on Earth. Phil. Trans. R. Soc. A. 363, 2793–2810. doi:10.1098/rsta.2005.1674
Bowling, T. J., Johnson, B. C., Melosh, H. J., Ivanov, B. A., O'Brien, D. P., Gaskell, R., et al. (2013). Antipodal Terrains Created by the Rheasilvia basin Forming Impact on Asteroid 4 Vesta. J. Geophys. Res. Planets 118 (9), 1821–1834. doi:10.1002/jgre.20123
Brenna, H., Kutterolf, S., Mills, M. J., and Krüger, K. (2020). The Potential Impacts of a Sulfur- and Halogen-Rich Supereruption Such as Los Chocoyos on the Atmosphere and Climate. Atmos. Chem. Phys. 20 (11), 6521–6539. doi:10.5194/acp-20-6521-2020
Brenna, H., Kutterolf, S., Mills, M. J., Niemeier, U., Timmreck, C., and Krüger, K. (2021). Decadal Disruption of the QBO by Tropical Volcanic Supereruptions. Geophys. Res. Lett. 48 (5), e2020GL089687. doi:10.1029/2020gl089687
Brum, J., Gennisson, J. L., Fink, M., Tourin, A., and Jia, X. (2019). Drastic Slowdown of the Rayleigh-like Wave in Unjammed Granular Suspensions. Phys. Rev. E 99 (4), 042902. doi:10.1103/PhysRevE.99.042902
Caricchi, L., Annen, C., Blundy, J., Simpson, G., and Pinel, V. (2014). Frequency and Magnitude of Volcanic Eruptions Controlled by Magma Injection and Buoyancy. Nat. Geosci. 7 (2), 126–130. doi:10.1038/ngeo2041
Chapman, S., Borgomano, J. V., Quintal, B., Benson, S. M., and Fortin, J. (2021). Seismic Wave Attenuation and Dispersion Due to Partial Fluid Saturation: Direct Measurements and Numerical Simulations Based on X‐Ray CT. J. Geophys. Res. Solid Earth 126 (4), e2021JB021643. doi:10.1029/2021jb021643
Chesner, C. A., and Luhr, J. F. (2010). A Melt Inclusion Study of the Toba Tuffs, Sumatra, Indonesia. J. Volcanol. Geothermal Res. 197 (1-4), 259–278. doi:10.1016/j.jvolgeores.2010.06.001
Chesner, C. A., Rose, W. I., Deino, A., Drake, R., and Westgate, J. A. (1991). Eruptive History of Earth's Largest Quaternary Caldera (Toba, Indonesia) Clarified. Geol 19 (3), 200–203. doi:10.1130/0091-7613(1991)019<0200:ehoesl>2.3.co;2
Chesner, C. A. (1998). Petrogenesis of the Toba Tuffs, Sumatra, indonesia. J. Petrol. 39 (3), 397–438. doi:10.1093/petroj/39.3.397
Cisneros de León, A., Schindlbeck‐Belo, J. C., Kutterolf, S., Danišík, M., Schmitt, A. K., Freundt, A., et al. (2021). A History of Violence: Magma Incubation, Timing and Tephra Distribution of the Los Chocoyos Supereruption (Atitlán Caldera, Guatemala). J. Quat. Sci. 36 (2), 169–179. doi:10.1002/jqs.3265
Costa, A., Smith, V. C., Macedonio, G., and Matthews, N. E. (2014). The Magnitude and Impact of the Youngest Toba Tuff Super-eruption. Front. Earth Sci. 2, 16. doi:10.3389/feart.2014.00016
Crick, L., Burke, A., Hutchison, W., Kohno, M., Moore, K. A., Savarino, J., et al. (2021). New Insights into the ∼ 74 Ka Toba Eruption from Sulfur Isotopes of Polar Ice Cores. Clim. Past 17 (5), 2119–2137. doi:10.5194/cp-17-2119-2021
Crosweller, H. S., Arora, B., Brown, S. K., Cottrell, E., Deligne, N. I., Guerrero, N. O., et al. (2012). Global Database on Large Magnitude Explosive Volcanic Eruptions (LaMEVE). J. Appl. Volcanol. 1 (1), 1–13. doi:10.1186/2191-5040-1-4
Davis, M., Koenders, M., and Petford, N. (2007). Vibro-agitation of Chambered Magma. J. Volcanol. Geothermal Res. 167 (1-4), 24–36. doi:10.1016/j.jvolgeores.2007.07.012
de Silva, S. L., and Gregg, P. M. (2014). Thermomechanical Feedbacks in Magmatic Systems: Implications for Growth, Longevity, and Evolution of Large Caldera-Forming Magma Reservoirs and Their Supereruptions. J. Volcanol. Geothermal Res. 282, 77–91. doi:10.1016/j.jvolgeores.2014.06.001
de Silva, S., Zandt, G., Trumbull, R., Viramonte, J. G., Salas, G., and Jiménez, N. (2006). Large Ignimbrite Eruptions and Volcano-Tectonic Depressions in the Central Andes: a Thermomechanical Perspective. Geol. Soc. Lond. Spec. Publ. 269 (1), 47–63. doi:10.1144/gsl.sp.2006.269.01.04
Deligne, N., Coles, S., and Sparks, R. (2010). Recurrence Rates of Large Explosive Volcanic Eruptions. J. Geophys. Res. Solid Earth 115 (B6), 1–16. doi:10.1029/2009jb006554
Denton, C. A., Johnson, B. C., Wakita, S., Freed, A. M., Melosh, H. J., and Stern, S. A. (2021). Pluto's Antipodal Terrains Imply a Thick Subsurface Ocean and Hydrated Core. Geophys. Res. Lett. 48 (2), e2020GL091596. doi:10.1029/2020gl091596
Dörfler, M. A., and Kenkmann, T. (2020). Central Uplift Collapse in Acoustically Fluidized Granular Targets: Insights from Analog Modeling. Meteorit Planet. Sci. 55 (2), 441–456. doi:10.1111/maps.13442
Drexler, J. W., Rose, W. I., Sparks, R. S. J., and Ledbetter, M. T. (1980). The Los Chocoyos Ash, Guatemala: a Major Stratigraphic Marker in Middle America and in Three Ocean Basins. Quat. Res. 13 (3), 327–345. doi:10.1016/0033-5894(80)90061-7
Giacco, F., De Arcangelis, L., Ciamarra, M. P., and Lippiello, E. (2018). Synchronized Oscillations and Acoustic Fluidization in Confined Granular Materials. Phys. Rev. E 97 (1), 010901. doi:10.1103/PhysRevE.97.010901
Gomberg, J., Blanpied, M. L., and Beeler, N. (1997). Transient Triggering of Near and Distant Earthquakes. Bull. Seismol. Soc. Am. 87 (2), 294–309.
Gonnermann, H. M., Foster, J. H., Poland, M., Wolfe, C. J., Brooks, B. A., and Miklius, A. (2012). Coupling at Mauna Loa and Kīlauea by Stress Transfer in an Asthenospheric Melt Layer. Nat. Geosci. 5 (11), 826–829. doi:10.1038/ngeo1612
Gravley, D., Wilson, C., Leonard, G., and Cole, J. (2007). Double Trouble: Paired Ignimbrite Eruptions and Collateral Subsidence in the Taupo Volcanic Zone, New Zealand. Geol. Soc. Am. Bull. 119 (1-2), 18–30. doi:10.1130/b25924.1
Gregg, P. M., Grosfils, E. B., and de Silva, S. L. (2015). Catastrophic Caldera-Forming Eruptions II: The Subordinate Role of Magma Buoyancy as an Eruption Trigger. J. Volcanol. Geothermal Res. 305, 100–113. doi:10.1016/j.jvolgeores.2015.09.022
Gudmundsson, A. (2014). Elastic Energy Release in Great Earthquakes and Eruptions. Front. Earth Sci. 2, 10. doi:10.3389/feart.2014.00010
Gudmundsson, A. (2016). The Mechanics of Large Volcanic Eruptions. Earth Sci. Rev. 163, 72–93. doi:10.1016/j.earscirev.2016.10.003
Hagstrum, J. T. (2005). Antipodal Hotspots and Bipolar Catastrophes: Were Oceanic Large-Body Impacts the Cause? Earth Planet. Sci. Lett. 236 (1-2), 13–27. doi:10.1016/j.epsl.2005.02.020
Hill, D. P., Pollitz, F., and Newhall, C. (2002). Earthquake-volcano Interactions. Phys. Today 55 (11), 41–47. doi:10.1063/1.1535006
Hill-Butler, C., Blackett, M., Wright, R., and Trodd, N. (2020). The Co-incidence of Earthquakes and Volcanoes: Assessing Global Volcanic Radiant Flux Responses to Earthquakes in the 21st century. J. Volcanol. Geothermal Res. 393, 106770. doi:10.1016/j.jvolgeores.2020.106770
Hood, L. L., and Artemieva, N. A. (2008). Antipodal Effects of Lunar basin-forming Impacts: Initial 3D Simulations and Comparisons with Observations. Icarus 193 (2), 485–502. doi:10.1016/j.icarus.2007.08.023
Klemetti, E. W., and Clynne, M. A. (2014). Localized Rejuvenation of a crystal Mush Recorded in Zircon Temporal and Compositional Variation at the Lassen Volcanic Center, Northern California. PloS one 9 (12), e113157. doi:10.1371/journal.pone.0113157
Krüger, K., Kutterolf, S., and Hansteen, T. H. (2015). “Halogen Release from Plinian Eruptions and Depletion of Stratospheric Ozone,” in Volcanism and Global Environmental Change. Editors A. Schmidt, K. Fristad, and L. Elkins-Tanton (Cambridge: Cambridge University Press), 244–259.
Kutterolf, S., Hansteen, T. H., Freundt, A., Wehrmann, H., Appel, K., Krüger, K., et al. (2015). Bromine and Chlorine Emissions from Plinian Eruptions along the Central American Volcanic Arc: From Source to Atmosphere. Earth Planet. Sci. Lett. 429, 234–246. doi:10.1016/j.epsl.2015.07.064
Kutterolf, S., Schindlbeck, J. C., Anselmetti, F. S., Ariztegui, D., Brenner, M., Curtis, J., et al. (2016). A 400-ka Tephrochronological Framework for Central America from Lake Petén Itzá (Guatemala) Sediments. Quat. Sci. Rev. 150, 200–220. doi:10.1016/j.quascirev.2016.08.023
Kutterolf, S., Schindlbeck- Belo, J. C., Rohr, I., Rademacher, M., Cisneros de León, A., Eisele, S., et al. (2019). The Arce Tephra: Two Subsequent Paroxysmal Plinian Eruptions from Coatepeque Caldera (El Salvador). J. Volcanol. Geothermal Res. 390, 106673. doi:10.1016/j.jvolgeores.2019.106673
Léopoldès, J., Conrad, G., and Jia, X. (2013). Onset of Sliding in Amorphous Films Triggered by High-Frequency Oscillatory Shear. Phys. Rev. Lett. 110 (24), 248301. doi:10.1103/physrevlett.110.248301
Ledbetter, M. T., and Sparks, R. S. J. (1979). Duration of Large-Magnitude Explosive Eruptions Deduced from Graded Bedding in Deep-Sea Ash Layers. Geol 7 (5), 240–244. doi:10.1130/0091-7613(1979)7<240:doleed>2.0.co;2
Li, Y., Luo, C., Zhang, J.-M., Liu, F., and Wang, R. (2022). Rayleigh Wave-Shear Wave Coupling Mechanism for Large Lateral Deformation in Level Liquefiable Ground. Comput. Geotech. 143, 104631. doi:10.1016/j.compgeo.2022.104631
Linde, A. T., and Sacks, I. S. (1998). Triggering of Volcanic Eruptions. Nature 395 (6705), 888–890. doi:10.1038/27650
Liu, P.-P., Caricchi, L., Chung, S.-L., Li, X.-H., Li, Q.-L., Zhou, M.-F., et al. (2021). Growth and thermal Maturation of the Toba Magma Reservoir. Proc. Natl. Acad. Sci. 118 (45), e2101695118. doi:10.1073/pnas.2101695118
Manga, M., and Brodsky, E. (2006). Seismic Triggering of Eruptions in the Far Field: Volcanoes and Geysers. Annu. Rev. Earth Planet. Sci. 34, 263–291. doi:10.1146/annurev.earth.34.031405.125125
Mark, D. F., Petraglia, M., Smith, V. C., Morgan, L. E., Barfod, D. N., Ellis, B. S., et al. (2014). A High-Precision 40Ar/39Ar Age for the Young Toba Tuff and Dating of Ultra-distal Tephra: Forcing of Quaternary Climate and Implications for Hominin Occupation of India. Quat. Geochronol. 21, 90–103. doi:10.1016/j.quageo.2012.12.004
Mark, D. F., Renne, P. R., Dymock, R. C., Smith, V. C., Simon, J. I., Morgan, L. E., et al. (2017). High-precision 40Ar/39Ar Dating of Pleistocene Tuffs and Temporal Anchoring of the Matuyama-Brunhes Boundary. Quat. Geochronol. 39, 1–23. doi:10.1016/j.quageo.2017.01.002
Mason, B. G., Pyle, D. M., and Oppenheimer, C. (2004). The Size and Frequency of the Largest Explosive Eruptions on Earth. Bull. Volcanol. 66 (8), 735–748. doi:10.1007/s00445-004-0355-9
Meschede, M. A., Myhrvold, C. L., and Tromp, J. (2011). Antipodal Focusing of Seismic Waves Due to Large Meteorite Impacts on Earth. Geophys. J. Int. 187 (1), 529–537. doi:10.1111/j.1365-246x.2011.05170.x
Miller, C. F., and Wark, D. A. (2008). Supervolcanoes and Their Explosive Supereruptions. Elements 4 (1), 11–15. doi:10.2113/gselements.4.1.11
Müller, T. M., Gurevich, B., and Lebedev, M. (2010). Seismic Wave Attenuation and Dispersion Resulting from Wave-Induced Flow in Porous Rocks - A Review. Geophysics 75 (5), 75A147–75A164. doi:10.1190/1.3463417
Mucek, A. E., Danišík, M., de Silva, S. L., Miggins, D. P., Schmitt, A. K., Pratomo, I., et al. (2021). Resurgence Initiation and Subsolidus Eruption of Cold Carapace of Warm Magma at Toba Caldera, Sumatra. Commun. Earth Environ. 2 (1), 1–9. doi:10.1038/s43247-021-00260-1
Newhall, C. G., Paull, C. K., Bradbury, J. P., Higuera-Gundy, A., Poppe, L. J., Self, S., et al. (1987). Recent Geologic History of lake Atitlán, a Caldera lake in Western Guatemala. J. Volcanol. Geothermal Res. 33 (1), 81–107. doi:10.1016/0377-0273(87)90055-2
Niespolo, E. M., Rutte, D., Deino, A. L., and Renne, P. R. (2017). Intercalibration and Age of the Alder Creek Sanidine 40Ar/39Ar Standard. Quat. Geochronol. 39, 205–213. doi:10.1016/j.quageo.2016.09.004
Nishimura, T. (2017). Triggering of Volcanic Eruptions by Large Earthquakes. Geophys. Res. Lett. 44 (15), 7750–7756. doi:10.1002/2017gl074579
O’Donovan, J., Ibraim, E., O’sullivan, C., Hamlin, S., Wood, D. M., and Marketos, G. (2016). Micromechanics of Seismic Wave Propagation in Granular Materials. Granular Matter 18 (3), 1–18. doi:10.1007/s10035-015-0599-4
O'Malley, R. T., Mondal, D., Goldfinger, C., and Behrenfeld, M. J. (2018). Evidence of Systematic Triggering at Teleseismic Distances Following Large Earthquakes. Sci. Rep. 8 (1), 11611–11612. doi:10.1038/s41598-018-30019-2
O'Donnell, J. P., Brisbourne, A. M., Stuart, G. W., Dunham, C. K., Yang, Y., Nield, G. A., et al. (2019). Mapping Crustal Shear Wave Velocity Structure and Radial Anisotropy beneath West Antarctica Using Seismic Ambient Noise. Geochem. Geophys. Geosyst. 20 (11), 5014–5037. doi:10.1029/2019gc008459
Oppenheimer, C. (2002). Limited Global Change Due to the Largest Known Quaternary Eruption, Toba≈ 74 Kyr BP? Quat. Sci. Rev. 21 (14-15), 1593–1609. doi:10.1016/s0277-3791(01)00154-8
Osipov, S., Stenchikov, G., Tsigaridis, K., LeGrande, A. N., Bauer, S. E., Fnais, M., et al. (2021). The Toba Supervolcano Eruption Caused Severe Tropical Stratospheric Ozone Depletion. Commun. Earth Environ. 2 (1), 1–7. doi:10.1038/s43247-021-00141-7
Pyle, D. M. (2015). “Sizes of Volcanic Eruptions,” in The Encyclopedia of Volcanoes. Editors H. Sigurdsson (Amsterdam: Academic Press), 2nd Edition, 257–264.
Rampino, M. (2002). Supereruptions as a Threat to Civilizations on Earth-like Planets. Icarus 156 (2), 562–569. doi:10.1006/icar.2001.6808
Reid, M. R., and Vazquez, J. A. (2017). Fitful and Protracted Magma Assembly Leading to a Giant Eruption, Youngest Toba Tuff, Indonesia. Geochem. Geophys. Geosyst. 18 (1), 156–177. doi:10.1002/2016gc006641
Richards, M. A., Alvarez, W., Self, S., Karlstrom, L., Renne, P. R., Manga, M., et al. (2015). Triggering of the Largest Deccan Eruptions by the Chicxulub Impact. GSA Bull. 127 (11-12), 1507–1520. doi:10.1130/b31167.1
Robock, A., Ammann, C. M., Oman, L., Shindell, D., Levis, S., and Stenchikov, G. (2009). Did the Toba Volcanic Eruption of ∼74 Ka BP Produce Widespread Glaciation? J. Geophys. Res. Atmospheres 114 (D10), 1–9. doi:10.1029/2008jd011652
Rose, W. I., Newhall, C. G., Bornhorst, T. J., and Self, S. (1987). Quaternary Silicic Pyroclastic Deposits of Atitlán Caldera, Guatemala. J. Volcanol. Geothermal Res. 33 (1-3), 57–80. doi:10.1016/0377-0273(87)90054-0
Rose, W. I., Conway, F. M., Pullinger, C. R., Deino, A., and McIntosh, W. C. (1999). An Improved Age Framework for Late Quaternary Silicic Eruptions in Northern Central America. Bull. Volcanol. 61 (1-2), 106–120. doi:10.1007/s004450050266
Rougier, J., Sparks, R. S. J., Cashman, K. V., and Brown, S. K. (2018). The Global Magnitude-Frequency Relationship for Large Explosive Volcanic Eruptions. Earth Planet. Sci. Lett. 482, 621–629. doi:10.1016/j.epsl.2017.11.015
Sawi, T. M., and Manga, M. (2018). Revisiting Short-Term Earthquake Triggered Volcanism. Bull. Volcanol. 80 (7), 1–9. doi:10.1007/s00445-018-1232-2
Schultz, P. H., and Gault, D. E. (1975). Seismic Effects from Major basin Formations on the Moon and Mercury. Moon 12 (2), 159–177. doi:10.1007/bf00577875
Self, S. (2015). “Explosive Super-Eruptions and Potential Global Impacts,” in Volcanic Hazards, Risks and Disasters. Editors J. F. Shroder, and P. Papale (Boston: Elsevier), 399–418.
Seropian, G., Kennedy, B. M., Walter, T. R., Ichihara, M., and Jolly, A. D. (2021). A Review Framework of How Earthquakes Trigger Volcanic Eruptions. Nat. Commun. 12 (1), 1004–1013. doi:10.1038/s41467-021-21166-8
Sharma, M. D. (2012). Rayleigh Waves in Dissipative Poro-Viscoelastic Media. Bull. Seismol. Soc. Am. 102 (6), 2468–2483. doi:10.1785/0120120003
Simon, J. I., Renne, P. R., and Mundil, R. (2008). Implications of Pre-eruptive Magmatic Histories of Zircons for U–Pb Geochronology of Silicic Extrusions. Earth Planet. Sci. Lett. 266 (1), 182–194. doi:10.1016/j.epsl.2007.11.014
Sparks, S., Self, S., Pyle, D., Oppenheimer, C., Rymer, H., and Grattan, J. (2005). Super-eruptions: Global Effects and Future Threats. London: Geological Society of London, 28.
Stix, J., and Kobayashi, T. (2008). Magma Dynamics and Collapse Mechanisms during Four Historic Caldera‐forming Events. J. Geophys. Res. Solid Earth 113 (B9), 1–14. doi:10.1029/2007jb005073
Storey, M., Roberts, R. G., and Saidin, M. (2012). Astronomically Calibrated 40Ar/39Ar Age for the Toba Supereruption and Global Synchronization of Late Quaternary Records. Proc. Natl. Acad. Sci. U.S.A. 109 (46), 18684–18688. doi:10.1073/pnas.1208178109
Sulpizio, R., and Massaro, S. (2017). Influence of Stress Field Changes on Eruption Initiation and Dynamics: A Review. Front. Earth Sci. 5, 18. doi:10.3389/feart.2017.00018
Svensson, A., Bigler, M., Blunier, T., Clausen, H. B., Dahl-Jensen, D., Fischer, H., et al. (2013). Direct Linking of Greenland and Antarctic Ice Cores at the Toba Eruption (74 Ka BP). Clim. Past 9 (2), 749–766. doi:10.5194/cp-9-749-2013
Tarasewicz, J., Brandsdóttir, B., White, R. S., Hensch, M., and Thorbjarnardóttir, B. (2012). Using Microearthquakes to Track Repeated Magma Intrusions beneath the Eyjafjallajökull Stratovolcano, Iceland. J. Geophys. Res. Solid Earth 117 (B9), 1–13. doi:10.1029/2011jb008751
Tierney, C. R., Reid, M. R., Vazquez, J. A., and Chesner, C. A. (2019). Diverse Late-Stage Crystallization and Storage Conditions in Melt Domains from the Youngest Toba Tuff Revealed by Age and Compositional Heterogeneity in the Last Increment of Accessory Phase Growth. Contrib. Mineral. Petrol. 174 (4), 31. doi:10.1007/s00410-019-1566-6
Walter, T. R., Shirzaei, M., Manconi, A., Solaro, G., Pepe, A., Manzo, M., et al. (2014). Possible Coupling of Campi Flegrei and Vesuvius as Revealed by InSAR Time Series, Correlation Analysis and Time Dependent Modeling. J. Volcanol. Geothermal Res. 280, 104–110. doi:10.1016/j.jvolgeores.2014.05.006
Wang, Z., Gu, B. W., Yuan, Q., and Lv, Z. (2021). Wave Dissipation Performance of Granular Media Based on Three-Dimensional Meso-Model. IEEE Sensors J. 21 (14), 15607–15613. doi:10.1109/jsen.2020.3016600
Watts, A. W., Greeley, R., and Melosh, H. J. (1991). The Formation of Terrains Antipodal to Major Impacts. Icarus 93 (1), 159–168. doi:10.1016/0019-1035(91)90170-x
Waymel, R. F., Wang, E., Awasthi, A., Geubelle, P. H., and Lambros, J. (2018). Propagation and Dissipation of Elasto-Plastic Stress Waves in Two Dimensional Ordered Granular media. J. Mech. Phys. Sol. 120, 117–131. doi:10.1016/j.jmps.2017.11.007
Weinberg, R. F., Vernon, R. H., and Schmeling, H. (2021). Processes in Mushes and Their Role in the Differentiation of Granitic Rocks. Earth-Science Rev. 220, 103665. doi:10.1016/j.earscirev.2021.103665
Williams, D. A., and Greeley, R. (1994). Assessment of Antipodal-Impact Terrains on Mars. Icarus 110 (2), 196–202. doi:10.1006/icar.1994.1116
Williams, M. (2012). The ∼73 Ka Toba Super-eruption and its Impact: History of a Debate. Quat. Int. 258, 19–29. doi:10.1016/j.quaint.2011.08.025
Wilson, C. J. N., and Charlier, B. L. A. (2009). Rapid Rates of Magma Generation at Contemporaneous Magma Systems, Taupo Volcano, New Zealand: Insights from U-Th Model-Age Spectra in Zircons. J. Petrol. 50 (5), 875–907. doi:10.1093/petrology/egp023
Wilson, C. J. N., Cooper, G. F., Chamberlain, K. J., Barker, S. J., Myers, M. L., Illsley-Kemp, F., et al. (2021). No Single Model for Supersized Eruptions and Their Magma Bodies. Nat. Rev. Earth Environ. 2 (9), 610–627. doi:10.1038/s43017-021-00191-7
Winkler, K. W., and Nur, A. (1982). Seismic Attenuation: Effects of Pore Fluids and Frictional‐sliding. Geophysics 47 (1), 1–15. doi:10.1190/1.1441276
Yuan, Q., Kong, X., Zhang, J., Fang, Q., and Hong, J. (2021). Three-dimensional Mesoscopic Modelling of Shock Wave Propagation and Attenuation in Gravel Granular Filter. Powder Technol. 394, 838–852. doi:10.1016/j.powtec.2021.08.095
Zaccherini, R., Palermo, A., Marzani, A., Colombi, A., Dertimanis, V., and Chatzi, E. (2020). Mitigation of Rayleigh-like Waves in Granular media via Multi-Layer Resonant Metabarriers. Appl. Phys. Lett. 117 (25), 254103. doi:10.1063/5.0031113
Keywords: Atitlán caldera, Toba caldera, Los Chocoyos, Youngest Toba Tuff, Antipode
Citation: Cisneros de León A, Mittal T, de Silva SL, Self S, Schmitt AK and Kutterolf S (2022) On Synchronous Supereruptions. Front. Earth Sci. 10:827252. doi: 10.3389/feart.2022.827252
Received: 01 December 2021; Accepted: 22 March 2022;
Published: 08 April 2022.
Edited by:
Valerio Acocella, Roma Tre University, ItalyReviewed by:
Raffaello Cioni, University of Florence, ItalyCopyright © 2022 Cisneros de León, Mittal, de Silva, Self, Schmitt and Kutterolf. This is an open-access article distributed under the terms of the Creative Commons Attribution License (CC BY). The use, distribution or reproduction in other forums is permitted, provided the original author(s) and the copyright owner(s) are credited and that the original publication in this journal is cited, in accordance with accepted academic practice. No use, distribution or reproduction is permitted which does not comply with these terms.
*Correspondence: Alejandro Cisneros de León, YWNpc25lcm9zQHNlb2Uuc2MuZWR1
†Present address: Alejandro Cisneros de León, School of the Earth, Ocean and Environment, University of South Carolina, Columbia, SC, United States
‡These authors have contributed equally to this work
Disclaimer: All claims expressed in this article are solely those of the authors and do not necessarily represent those of their affiliated organizations, or those of the publisher, the editors and the reviewers. Any product that may be evaluated in this article or claim that may be made by its manufacturer is not guaranteed or endorsed by the publisher.
Research integrity at Frontiers
Learn more about the work of our research integrity team to safeguard the quality of each article we publish.