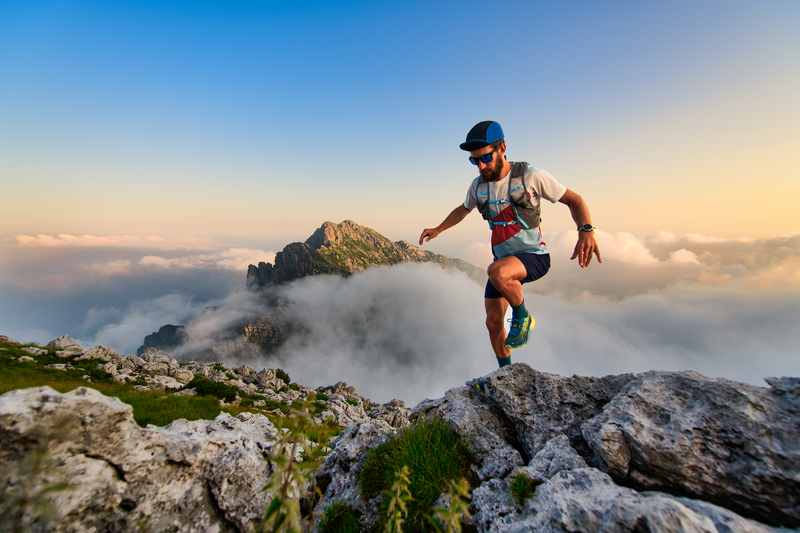
95% of researchers rate our articles as excellent or good
Learn more about the work of our research integrity team to safeguard the quality of each article we publish.
Find out more
ORIGINAL RESEARCH article
Front. Earth Sci. , 04 March 2022
Sec. Biogeoscience
Volume 10 - 2022 | https://doi.org/10.3389/feart.2022.826907
This article is part of the Research Topic Hydrobiogeochemistry of Major Asian Rivers View all 11 articles
Dissolved organic matter (DOM), particularly dissolved organic nitrogen (DON), is an important source of energy and/or organic nutrients for heterotrophic microorganisms in rivers. Although various factors controlling the quantity and quality of stream and riverine DOM have been extensively studied, DON has been under-researched compared to dissolved organic carbon (DOC). The spatial distribution of DOC and DON concentrations with respect to the C/N ratio and DOM optical properties was investigated in the Ishikari River and its tributaries in Hokkaido, northern Japan. Here, the upper reaches are forested and the middle and lower reaches are encompassed by agricultural land, in particular paddy fields. Furthermore, dark incubation experiments were conducted using filtered riverine water (<0.7 µm) to determine the bioavailability of DOC and DON (particularly due to small microorganisms) considered as a factor controlling the spatial distribution. In the mainstream, DOC and DON concentrations increased with river flow in the upper and middle reaches and remained unchanged in the lower reaches. The C/N ratio of bulk DOM decreased continuously from the upper reaches to lower reaches. The optical properties exhibited changes in the DOM characteristics in terms of higher molecular weight and higher aromaticity from the upper to middle reaches, suggesting that flooded paddy fields largely altered the riverine DOM concentration and composition. In the lower reaches, the C/N ratio of the bulk DOM decreased with the river flow. However, according to principal component analysis, no changes were observed in the optical properties with river flow, suggesting that the C/N ratio of bulk DOM changed owing to in situ biological activity in the river. DOC biodegradation was observed at four sites in the upper and middle reaches but not at the two sites in the lower reaches. However, the DON concentration during the dark incubation experiments at all sites did not differ significantly, which implies that microbial degradation, particularly by small microorganisms, is a factor that decreased the C/N ratio of bulk DOM in the upper and middle reaches. In contrast, large microorganisms possibly degraded C-rich DOM to decrease the C/N ratio of bulk DOM in the lower reaches of the Ishikari River.
Dissolved organic matter (DOM) in rivers is an essential component of biogeochemical cycles (Findlay and Sinsabaugh, 2003), and three major sources of DOM in rivers include 1) soils, 2) riparian zones, and 3) biological production in situ in rivers (Bertilsson and Jones, 2003; Willett et al., 2004; Yamashita et al., 2010; Bianchi, 2011; Yamashita et al., 2011; Drake et al., 2018). The chemical characteristics of DOM may vary depending on its origin. DOM is the source of energy and carbon in riverine ecosystems, particularly for heterotrophic microorganisms (Wetzel, 1992; Battin et al., 2016), and its bioavailability depends on its composition and structure (Amon and Benner, 1996; Amon et al., 2001). Therefore, the environmental dynamics of DOM in rivers must be determined by considering their origin and bioavailability for an improved understanding of riverine ecosystems.
In addition to providing energy, dissolved organic nitrogen (DON) plays an important role as an organic nutrient in riverine ecosystems (Wymore et al., 2015). The ratio of dissolved organic carbon (DOC) to DON (i.e., the C/N ratio of DOM) of bio-labile DOM is generally lower than that of bio-refractory DOM in marine environments (Hopkinson and Valino, 2005; Lønborg and Álvarez-Salgado, 2012), implying that nitrogen-containing DOM is relatively easily consumed by heterotrophic microbes in the ocean. The C/N ratio of riverine DOM is generally higher than that of marine DOM because of the inputs from soil-derived DOM, which has a higher C/N ratio (Lara et al., 1998; Lee et al., 2020). In contrast, DOM produced by algae in rivers and derived from wastewater and agricultural land have lower C/N values than soil-derived DOM (Westerhoff and Mash, 2002; Voss et al., 2021). Furthermore, Wiegner et al. (2006) conducted a degradation experiment in rivers on the east coast of the United States at 25°C under dark conditions for 6 days and observed that DON had a higher degradation rate and higher bioavailability than DOC. Similar results have been observed in different rivers (Hendrickson et al., 2007; Petrone et al., 2009; Wiegner et al., 2009; Islam et al., 2019). The contribution of protein-like fluorophores to total fluorophores has been related to the bioavailability of bulk DOM (Balcarczyk et al., 2009; Fellman et al., 2009; Petrone et al., 2011), implying that at least fractions of the DON, such as peptides and proteins, are actively involved in the metabolism of lower trophic levels in riverine ecosystems.
Previous studies have also suggested that DON bioavailability is controlled by dissolved inorganic nitrogen (DIN) concentration. Heterotrophic microorganisms, such as bacteria, can use DIN for nitrogen assimilation and DON as a carbon and energy source (Lutz et al., 2011; Wymore et al., 2015). DON concentration may increase with increasing DIN concentration under the condition that DON has the role of nutrient source for microorganisms (Wymore et al., 2015). On the other hand, Kaushal and Lewis (2005) and Pisani et al. (2017) proposed a model for the relationship between DIN concentration and DON bioavailability. In the model, DON bioavailability was reduced in DIN-rich environments.
The evaluation of factors controlling DOC concentration in rivers often involves comparison with the DOC quality determined by optical means along with its comparison with land use and land cover (Aitkenhead and McDowell, 2000; Mulholland, 2003; Yamashita et al., 2011; Lu et al., 2014; Mann et al., 2016; Williams et al., 2016; Connolly et al., 2018). However, compared with the factors controlling DOC concentration, those controlling the DON concentration and C/N ratio of bulk DOM in rivers have been evaluated in a relatively small number of studies. For example, Willet et al. (2004) observed that DON concentrations remained stable across a wide range of hydrologically and ecologically diverse catchments with highly varying DIN concentrations. Jacobs et al. (2017) compared DOC and DON concentrations in rivers with different land use patterns, namely forest, field, and plantation agriculture, and observed that both DOC and DON concentrations were not affected by land use land cover in the catchment. DOM in forest areas generally have higher C/N ratios than those in urban/suburban and agricultural areas (Seitzinger et al., 2002; Graeber et al., 2015; Heinz et al., 2015; Yates et al., 2019; Voss et al., 2021). Yates et al. (2019) also reported that the C/N ratio of DOM in the blanket peatland area was higher than that in the coniferous woodland area. In contrast, Bhattacharya and Osburn (2020) reported that the C/N ratio of stream DOM in the Neuse River Basin, the largest watershed in the state of North Carolina, United States, exhibited slightly higher values in wetland and agricultural areas than in urban and forested areas. Thus, the factors controlling DON concentrations and the C/N ratio of bulk DOM in rivers have not been well documented (Heinz et al., 2015).
In this study, we determined the spatial distribution of DOC and DON concentrations and the optical properties of DOM in the Ishikari River Basin in Hokkaido, northern Japan. The biodegradability of DOC and DON was also determined by 40-days dark incubation experiments. The aim of this study was to estimate the factors controlling the DOC and DON concentrations and C/N ratio of bulk DOM in the Ishikari River to improve our understanding of the influence of C/N ratio on the environmental dynamics of riverine DOM.
The study area was located in the catchment of the Ishikari River, Hokkaido, Japan (Figure 1). This stream originates from Mt. Ishikari (1967 m) and is the longest river in Hokkaido, with a basin area of 14,330 km2 and a river length of 268 km. The stream has many tributaries, from large to small, and transports freshwater to the Sea of Japan. The Ishikari River generally has a maximum annual flow in April because of snowmelt in the spring (Duan et al., 2013). In addition, the river flow is relatively high in August and September because of heavy precipitation from typhoons and fronts.
FIGURE 1. Map of the study area. White plots indicate the sampling sites and the number next to the plot denotes the site number. The black dotted lines divide upper, middle and lower reaches. This map was created by processing “Digital National Land Information (Data of Land use in 2016)" (Ministry of Land, Infrastructure, Transport and Tourism) (https://nlftp.mlit.go.jp/ksj/index.html).
The topography of the watershed is 60% mountains and 40% plains. Most of the upper reaches of the watershed are mountainous. The watershed of the upper reaches of the Ishikari River Basin comprises the Taisetsu Mountains, which are a group of volcanoes reaching heights of up to 2,000 m. In contrast, the lower reaches of the watersheds are plain, represented by the Ishikari Plain, and wetlands have also developed.
The land use land cover of watersheds is largely dominated by forests (Kunii and Saito, 2009; Duan et al., 2015). Alpine vegetation was evident in the headwaters of the watersheds. In other mountainous areas, deciduous broad-leaved forests are developed, with partial distribution of planted coniferous forests. Paddy fields are widely developed, in both basins and the plains. In addition, the land is utilized for other agricultural purposes such as cultivation of vegetables and beans. The cropping period for paddy fields and other agricultural land is approximately from April to October, with variations depending on the crop. Moreover, urban areas, such as Sapporo and Asahikawa, exist in the watershed.
In this study, stream water samples were collected from the Ishikari River and its eight major tributaries (Table 1; Figure 1). Thus, 27 sampling sites were selected mostly from bridges near gauging stations operated by the Hokkaido Development Bureau of the Ministry of Land, Infrastructure, Transport and Tourism. Fifteen sites were selected from the Ishikari River (mainstream) (1–15), four sites from the Uryu River (U1 to U4), two sites from Ikushunbetsu River (I1 and I2), and one site each from the Chubetsu, Biei, Osarappe, Sorachi, Yubari, and Chitose rivers (Chu1, B1, O1, S1, Y1, and Chi1, respectively) (Figure 1).
Observations were conducted at Sites 1–5 and U1–U4 on 23 July 2020; Sites 6–12, Chu1, B1, O1, and S1 on 24 July 2020; and Sites 13–15, I1–I2, Y1, and Chi1 on 27 July 2020. The weather on the observation days was sunny and cloudy, with no precipitation. Surface water samples were collected from the bridge using a stainless-steel bucket with a rope and poured into 500 ml acid-washed (soaked in 1 M hydrochloric acid overnight and washed with Milli-Q water) polycarbonate bottles. Both buckets and bottles utilized for water sampling were rinsed thrice with river water. The water temperature and electrical conductivity (EC) of the river water were measured using an electrical conductivity meter (LAQUAact ES-71, Horiba Scientific).
Samples were filtered on site through a pre-combusted (450°C, 3 h) glass fiber filter (Whatman GF/F filter, pore size: approximately 0.7 μm) under reduced pressure. The filtrate was dispensed into two acid-washed 10 ml acrylic tubes and two 40 ml glass vials and subsequently stored in a cooler. All tubes and glass vials were rinsed thrice with filtered sample water. Immediately after returning to the laboratory at the Faculty of Environmental Earth Science, Hokkaido University, the acrylic tubes were kept frozen in the dark for inorganic nutrient and total dissolved nitrogen (TDN) analyses. In addition, glass vials for DOM analyses were kept refrigerated in the dark.
The concentration of DOC (μMC) was measured by the combustion catalytic oxidation method using a total organic carbon analyzer (TOC-L CSH, Shimadzu). The DOC concentration was calculated from the standard curve using a potassium hydrogen phthalate solution and was determined daily.
DIN (NH4+, NO2−, and NO3−) and phosphate (PO4−) concentrations (µMN and µMP, respectively) were measured by colorimetric analysis using a continuous flow analyzer (QuAAtro, BRAN + LUEBBE GmbH). The TDN concentration (µMN) was determined by measuring the NO3− concentration after oxidative decomposition by the wet oxidation method using potassium peroxydisulfate (Yamashita et al., 2021). The detection limit was calculated as the sum of the mean of the blank concentration and thrice the standard deviation. The detection limits were 0.26 μMN for NH4+, 0.06 μMN for NO2−, 0.59 μMN for NO3− + NO2−, 0.02 μMP for PO4−, and 0.48 μMN for TDN. The DON concentration (µMN) was estimated by subtracting the DIN concentration from the TDN concentration. The C/N ratio of DOM was calculated as the molar ratio of the DOC concentration to the DON concentration.
UV-Vis absorption spectra were measured using a spectrophotometer (UV-1900i, Shimadzu) to measure the UV-Vis absorbance of DOM. These absorption spectra were acquired with a 5 cm quartz-windowed cell at wavelengths between 200 and 800 nm at 0.5 nm intervals. The absorbance spectrum of the sample was baseline-corrected by subtracting average values ranging from 683 to 687 nm from the entire spectrum, according to Babin et al. (2003).
The spectral slope ratio (SR) was determined as the ratio of the spectral slope of the shorter wavelength region (275–295 nm) to that of the longer wavelength region (350–400 nm) (Helms et al., 2008). SR is known to be related to the molecular weight of DOM, with a high value representing low molecular weight organic matter and vice versa. The specific UV absorbance at 254 nm (SUVA254), an index representing the relative contribution of aromatic compounds of DOC, was calculated by dividing the decadic absorption coefficient at 254 nm [A254 (m−1)] by the DOC concentration (mg L−1) (Weishaar et al., 2003).
The fluorescence properties of DOM were measured using a spectrofluorometer (Fluoromax-4, Horiba Scientific). Excitation-emission matrix (EEM) spectra were acquired in a 1 cm quartz cell at excitation wavelengths between 250 and 450 nm at 5 nm intervals according to Tanaka et al. (2014), with few modifications. The emission wavelengths were acquired from 290 to 550 nm at 2 nm intervals. The integration time was set to 0.1 s. All fluorescence spectra were determined after inner filter correction with absorbance spectrum (McKnight et al., 2001), instrumental bias correction (Cory et al., 2010), and subtraction of the EEM of Milli-Q water. The fluorescence unit was converted to a Raman unit with a water Raman peak analyzed daily at 350 nm excitation of Milli-Q water (Lawaetz and Stedmon, 2009).
Three typical peaks were determined from the EEM patterns of samples from the Ishikari River and its major tributaries. The peaks were categorized as B peak (Ex/Em = 275/310, protein-like), A peak (Ex/Em = 250/450, humic-like), and C peak (Ex/Em = 320/440, humic-like) according to (Coble 1996; Coble et al., 1998). Ratios of fluorescence intensity of protein-like peak B to that of humic-like peaks A or C (B/C or B/A) were determined as qualitative parameters of fluorescent DOM. The fluorescence index (FI) was determined as the ratio of the fluorescence intensity at emission wavelengths of 470 and 520 nm with that at an excitation wavelength of 370 nm (McKnight et al., 2001; Cory and McKnight, 2005). The FI is an indicator of the origin of humic-like fluorescent DOM; lower and higher values of FI indicate allochthonous sources and autochthonous sources, respectively (McKnight et al., 2001). The humification index (HIX) indicates the degree of humification of DOM and was determined as the ratio of the peak area at 435–480 and 300–345 nm with that at 255 nm excitation (Zsolnay, 2003). The higher the value of HIX, the more highly conjugated the humic-like substances, and vice versa (Zsolnay, 2003).
To evaluate the bioavailability of DOC and DON in stream water, biodegradation experiments were conducted at six sites (Sites 1, 6, 10, 11, 14, and 15) from the mainstream of the Ishikari River according to Hasegawa et al. (2010) and Yamashita et al. (2013), with few modifications.
The sample water was filtered through a Whatman GF/F filter (pore size: approximately 0.7 μm), dispensed into three 40 ml glass vials for each site, and incubated for 40 days in a cool incubator (CN-40A, Mitsubishi Electric) under dark conditions at 20°C, similar to the river water temperature at the time of observation. After 40 days of incubation, the DOC concentration was measured immediately, without further filtration. The sample water was dispensed into acid-washed 10 ml acrylic tubes and kept frozen until DIN concentration measurement. Although this bioassay method is simpler and expected to have lower possibility of contamination than the method of adding inoculum, large microbes might be removed from the incubations by filtration with the GF/F filter (Hasegawa et al., 2010).
The average of the triplicate incubation results was implemented as the concentration after the bioassay. In addition, standard deviation was used to determine the error associated with the bioassay. The amount of decomposed DOC was calculated by subtracting the DOC concentration after the bioassay from that before. The amount of DON decomposition was calculated by subtracting the DIN concentration before the bioassay from that after, considering that DIN is not converted to organic matter or lost during the decomposition experiment; instead, DIN is produced by the decomposition of DON. The rate of change (in %) was calculated by dividing the amount of decomposed DOC (DON) by the DOC (DON) concentration before the bioassay and multiplying it by 100.
Linear regression analysis was performed to examine the relationship between DON and DOC concentrations. The Pearson’s correlation coefficient was determined for each parameter. Principal component analysis (PCA) was performed on the data pertaining to the C/N ratio of DOM, FI, HIX, B/C, B/A, A/C, SUVA254, and SR to comprehensively evaluate the chemical properties of DOM at each site. All statistical analyses were performed using R 4.1.1 (R Core Team, 2021).
The water temperature and EC values are presented in Table 1. The water temperature ranged from 12.9 to 23.7°C with an overall average of 18.9°C. In the upper reaches, the water temperature was lower than that in the lower reaches of the mainstream (Ishikari River), and in the major tributaries, it was similar to or lower than that in the mainstream.
The EC ranged from 0.070 to 0.161 mS cm−1 in the mainstream and from 0.068 to 0.212 mS cm−1 in the major tributaries. Regardless of the mainstream or tributaries, the EC in the upper reaches was lower than that in the lower reaches.
DOC concentrations, DON concentrations, and the C/N ratio of DOM are summarized in Table 2, and their spatial variations are shown in Figure 2. In the mainstream, the DOC concentration ranged from 34 μMC (Site 1) to 197 μMC (Site 10). The DOC concentration in the mainstream increased with the river flow in the upper and middle reaches and remained unchanged in the lower reaches. The DOC concentration in each major tributary was generally lower than that at each confluence with the mainstream, except for the Uryu River. The highest DOC concentration (310 μMC) was observed in the middle reaches of the Uryu River (Site U2).
FIGURE 2. The spatial distribution of the (A) Dissolved organic carbon (DOC) concentration, (B) Dissolved organic nitrogen (DON) concentration, and (C) Carbon/nitrogen (C/N) ratio in the Ishikari River Basin. White plots represent mainstream and black plots represent major tributaries. Plots of major tributaries are inserted at the confluence with the mainstream. The lowermost reaches of each tributary are plotted in the figures. The black dotted lines divide upper, middle and lower reaches.
The DON concentration in the mainstream ranged from 1.8 μMN (Site 1) to 14.0 μMN (Site 15) and increased with the river flow in the upper and middle reaches, remaining unchanged in the lower reaches. As with the DOC concentrations, each major tributary demonstrated lower DON concentrations than the concentration at each confluence with the mainstream, with the exception of the Uryu River. The highest DON concentration (15.4 μMN) was observed in the lower reaches of the Uryu River (Site U4).
A positive linear relationship existed between DOC and DON concentrations in the Ishikari River and the tributaries (Figure 3).
FIGURE 3. Relationship between dissolved organic nitrogen (DON) and dissolved organic carbon (DOC) concentrations. White plots represent the mainstream, and black plots represent the major tributaries. The black dotted line is a linear regression line for all samples.
The C/N ratio exhibited spatial variation in the Ishikari River Basin (Table 2; Figure 2). In the mainstream, the C/N ratio ranged from 12.1 (Site 15) to 22.0 (Site 3) and continuously decreased with the river flow from the upper to the lower reaches. The C/N ratio of the major tributaries (12.3–23.6) was similar to that of the mainstream.
The DIN and PO4− concentrations are listed in Table 2. The DIN concentration ranged from 7.4 to 76.8 μMN in the Ishikari River and the major tributaries. DIN concentrations in the upper reaches were lower than those in the middle and lower reaches. The DIN concentrations in the major tributaries were heterogeneous. Overall, the DIN concentrations were approximately three times higher than the DON concentrations at most sites.
The PO4− concentration was less than 1 μMP at all sites (ranging from 0.08 to 0.99 μMP). The PO4− concentrations in the upper reaches were lower than those in the middle and lower reaches of the mainstream. In the major tributaries, the PO4− concentrations were distributed heterogeneously. The N/P ratio of inorganic nutrients ranged from 25 to 293, with an average ± standard deviation of 95 ± 52.
The optical properties of DOM, such as SR, SUVA254, B/C, B/A, A/C, FI, and HIX, at each site are summarized in Table 2.
SR in the Ishikari River Basin ranged from 0.65 at the Chitose River (Site Chi1) to 1.06 at the upper reaches of the mainstream (Site 1) with average ± standard deviation of 0.84 ± 0.09. SR in the mainstream decreased with the river flow in the upper reaches and remained unchanged in the middle and lower reaches. The SR values of the major tributaries were heterogeneously distributed.
The overall average ± standard deviation of SUVA254 is 4.05 ± 0.68 (2.47 at Site 1 to 5.34 at Site Chi1). In the mainstream, SUVA254 increased with the river flow in the upper and lower reaches, while the SUVA254 values in the middle reaches and in major tributaries were heterogeneously distributed.
In the mainstream, the ratio of protein-like fluorophores to humic-like fluorophores, B/C and B/A, was higher in the middle reaches (in particular Sites 6 to 8 and 11) than in the upper and lower reaches, even though the ratios in the most upstream site (Site 1) had high values. The ratios in the major tributaries (Sites I2, Y1, and Chi1) whose confluence was located at the lower reaches were higher than those in the major tributaries with confluence in the upper and middle reaches.
The ratio of humic-like fluorophores, A/C, in the Ishikari River and the major tributaries did not change considerably (from 1.74 at Site 7 to 2.12 at Site I2, average ± standard deviation = 1.91 ± 0.09).
The FI exhibited a narrow range from 1.61 at the most upper reaches of the mainstream (Site 1) to 1.41 at the upper reaches of the Uryu River (Site U1), with an average ± standard deviation of 1.54 ± 0.04. The value in the mainstream increased with the river flow from Sites 2 to 7 and then decreased toward Site 14. The values of the major tributaries exhibited a heterogeneous distribution.
The HIX ranged from 3.51 at the Ikushunbetsu River (Site I2) to 7.99 at the most upper reaches of the mainstream (Site 1), and its average ± standard deviation was 5.05 ± 0.09. The HIX value in the mainstream was the highest in the uppermost site (Site 1), decreased with river flow up to Site 7, and then increased slightly toward Site 10, even though the values in the lower reaches did not change significantly. The HIX in the major tributaries was distributed heterogeneously.
The PCA results are shown in Figure 4. PC1 accounted for a variance of 33%, with a large positive coefficient of HIX and negative coefficients of B/A and B/C. In addition, PC2 accounted for a variance of 26%, with a large positive coefficient of SUVA254 and a large negative coefficient of SR. In particular, the positive score of PC1 indicated high humification, and the negative score indicated a high abundance of protein-like fluorophores. Furthermore, PC2 demonstrated a high molecular weight and aromaticity with a positive score and a low molecular weight with a negative score. On one hand, in the mainstream, a large positive PC1 score was observed in the upper reaches (Sites 1, 2, and 3) and some of the middle and lower reaches (Sites 10, 12, 13, and 15). On the other hand, a large negative PC1 was evident in the middle reaches (Sites 6, 7, and 8). For PC2, sites in the upper and middle reaches (Sites 1, 2, 3, 6, 7, and 8) exhibited negative scores, while other sites in the middle reaches (Sites 4, 5, and 9–11) and those in the lower reaches exhibited positive scores. The PC scores of the tributaries were generally more dispersed than those of the mainstream.
FIGURE 4. Results of principal component analysis (PCA) (A) coefficients, and (B) scores using qualitative indicators of dissolved organic matter (DOM) at each site. White and black plots in (b) represent the mainstream and major tributaries, respectively. The number next to the plot is the site number.
The changes in DOC and DON concentrations in the 40-days dark incubation experiments are shown in Figure 5. The reproducibility of the triplicate incubations was good, and 17–29% of the initial DOC decreased (in 40 days) at four sites in the upper and middle reaches. In contrast, the DOC concentrations did not change significantly at the two sites in the lower reaches.
FIGURE 5. Changes in the (A) dissolved organic carbon (DOC) concentrations and (B) dissolved organic nitrogen (DON) concentrations associated with the 40-days bioassay. Error bars represent the standard deviation from triplicate incubation.
The DON concentrations at any of the six sites did not significantly differ before and after incubation, because variations in DON concentration by incubation were smaller than the standard deviation of the triplicate incubations.
The DOC and DON concentrations in the mainstream increased with the river flow in the upper and middle reaches and remained unchanged in the lower reaches (Figure 2). A positive linear relationship was observed between DOC and DON concentrations in the Ishikari River and the major tributaries (Figure 3). The spatial distribution of DOC and DON concentrations and their linear relationship indicate that allochthonous DOM inputs mostly dominated DOM in the Ishikari River Basin, as suggested by Bernal et al. (2018). DOC and DON concentrations were negatively and positively correlated with SR and SUVA254, respectively (Figure 6), implying that allochthonous DOM might be characterized by high molecular weight and high aromaticity. Interestingly, the DOC and DON concentrations in each major tributary were generally lower than those at each confluence with the mainstream (Figure 2), suggesting that major tributaries were not the major factors controlling the DOM concentration and composition in the mainstream.
FIGURE 6. Matrix of Pearson’s correlation coefficients among each parameter. The significance is indicated by * (p < 0.05), ** (p < 0.01) and N.S. (non-significant). The sample number for analysis was 27.
A decrease in the C/N ratio of bulk DOM with the river flow from the upper reaches to the lower reaches of the Ishikari River (Figure 2) suggests: 1) changes in the C/N ratio of allochthonous DOM from minor tributaries, including the agricultural channel (which were not observed in this study), from the upper to the lower reaches, 2) substantial contribution of DOM from riverine riparian zone and biological production, or 3) preferential removal of C-rich DOM than N-rich DOM.
The DOC and DON concentrations increased slightly in the upper reaches of the Ishikari River (Sites 1–3) and nearly doubled between the upper (Site 3) and the middle (Site 4) reaches. The C/N ratio of bulk DOM decreased substantially from Sites 3 to 4 (Figure 2). The physical and chemical properties of watershed soils are strongly related to land use land cover (Smith, 2008), which might induce a relationship between land use land cover and DOM concentration (Yates et al., 2019). In this study, the upper reaches (Sites 1–3) were located in a canyon in the mountains, and most of the land use land cover in the watershed was forest. However, Site 4 was located in the basin, with paddy fields and urban areas distributed around the site (Figure 1). Connolly et al. (2018) stated that a small slope in the watershed increases the residence time of water in the soil, which enhances the leaching of DOM from the soil and, thus, increases the DOM concentration in the stream. The contribution of wetlands in the watershed is a well-known and major factor controlling riverine DOC concentrations (Mulholland, 2003; Laudon et al., 2004; Yamashita et al., 2010). Paddy field outflow has also been suggested as a source of DOM in riverine water (Ruark et al., 2010; Krupa et al., 2012; Suzuki et al., 2015). The quality of DOM at Site 1, evaluated using PCA, could be characterized as having a low molecular weight and low aromaticity. The quality changed to high molecular weight and high aromaticity at Site 4 (Figure 4). Reportedly, DOM originating from forests has low molecular weight (Inamdar et al., 2012; Bhattacharya and Osburn, 2020). However, DOM from agriculture and wetlands have high molecular weights and are rich in humic (Royer and David, 2005; Fellman et al., 2010; Bhattacharya and Osburn, 2020). Allochthonous DOM with humic characteristics was reported to load from flooded paddy fields to tributaries during the cropping period, which strongly influenced the DOM quality in tributaries (Abe et al., 2011). These results imply that the increase in DOM concentration accompanying the change in the quality from the upper to the middle reaches is probably due to the contribution of the paddy field outflow to the mainstream.
The DOC and DON concentrations increased with decreasing C/N ratio in the middle reaches of the Ishikari River (Sites 4–11) (Figure 2). Moreover, the changes in these concentrations could not always be explained by the contribution of high levels of DOC and DON from major tributaries (Figure 2). This implies that the contribution of high levels of DOM with low C/N ratios from minor tributaries, riparian zones, and/or biological production is a probable reason for the increase in DOC and DON concentrations. The DOM composition in downstream of major tributaries is affected by various land use land cover in the wide area of the watershed of the major tributaries, while that in minor tributaries (including agricultural channels) may be affected by simple land use land cover because the watershed area of the minor tributaries is relatively small. Therefore, similar to the change in DOM quantity from the upper to the middle reaches, the increase in DOM concentrations might be due to the contribution of flooded paddy field-derived DOM from minor tributaries that were not sampled in this study. Furthermore, the PCA results showed that the DOM was rich in protein-like fluorophores in the middle reaches, particularly at Sites 6–8 and 11. Protein-like fluorophore-rich DOM might have originated from microbial sources and recent biological activity in both stream water and riparian zones (Bernal et al., 2018). Moreover, the autochthonous DOM, such as proteins, is known to increase in the middle reaches owing to the open canopy of riparian forests and shallow water depths that facilitate biological production, such as algal growth. The production of protein-rich materials by algae decreases the C/N ratio of bulk riverine DOM (Westerhoff and Mash, 2002). In addition, a negative correlation was evident between the C/N ratio of the bulk DOM and FI (R = 0.62, p < 0.01; Figure 6). These results imply that the C/N ratio in the middle reaches was affected by the contribution of autochthonous DOM, including protein-like and autochthonous humic-like fluorophores. Furthermore, the influx of groundwater-derived DOM from the riparian zone in the middle reaches might have reduced the C/N ratio. This is because low molecular weight and protein-like DOM is abundant in the groundwater, as high molecular weight and aromatic DOM is adsorbed by the soil (Inamdar et al., 2012).
In the lower reaches of the Ishikari River (Sites 12–15), DOC and DON concentrations were variable, but the C/N ratio tended to decrease with the river flow. The major tributaries did not affect the DOM concentration substantially in the mainstream of the Ishikari River (Figure 2). PCA loadings in the downstream sites were plotted within a narrow region with positive PC1 and PC2 (Figure 4), implying that the composition of allochthonous DOM from minor tributaries, including the agricultural channels (which were not observed in this study), was similar to that of the mainstream at the lower reaches of the Ishikari River. These results imply that the contribution of allochthonous DOM from minor tributaries, including agricultural channels flowing directly into the mainstream, might be an important factor controlling the DOC and DON concentrations in the lower reaches of the Ishikari River. DOM in the lower reaches had higher molecular weights and higher aromaticity than those in the upper reaches (Figure 4). Thus, similar to the changes in the quantity and quality of DOM in the upper to middle reaches, paddy field-derived DOM was the probable source for the minor tributaries, including the agricultural channels. Although the DOM quality, mainly determined by its optical properties, was similar in the lower reaches, the C/N ratio (which was not largely influenced in the PCA) of bulk DOM decreased with the river flow in the lower reaches, suggesting that the C/N ratio of paddy field-derived DOM might be changed in situ in the mainstream.
The preferential removal of C-rich or N-rich DOM may result in negligible changes in the DOM concentrations but is evident as a change in the C/N ratio (Wymore et al., 2015). Photochemical and microbial degradation is the major degradation process for riverine DOM (Wiegner and Seitzinger, 2001; Cory and Kling, 2018). The humic-like substances and lignin phenols, which are components of aromatic allochthonous DOM, are preferentially degraded by sunlight (Opsahl and Benner, 1998; Moran et al., 2000; Benner and Kaiser, 2011) and might contribute to the decrease in the C/N ratio of DOM along with river flow. However, SUVA254, which is an index of the relative contribution of aromatic compounds of DOC, increased with the river flow in the upper and lower reaches. The SR values have been reported to increase with the photodegradation of DOM (Helms et al., 2008), while they do not change with the photodegradation of oceanic DOM (Yamashita et al., 2013). The SR values decreased in the upper reaches and remained unchanged in the middle and lower reaches, implying that variations in SR in the Ishikari River cannot be explained solely by photodegradation. Furthermore, peak A-type fluorescence components have been reported to be photodegradation products or photorefractory components (Chen et al., 2010; Yamashita et al., 2010). The ratio of humic-like fluorophores (A/C) in the Ishikari River did not change significantly with the river flow. These distribution patterns of the optical proxies imply that photodegradation is not a major process controlling the optical properties of DOM and the C/N ratio of DOM in the Ishikari River.
Some studies have reported the preferential removal of amino acids and neutral sugars by riverine and marine microbes (Yamashita and Tanoue, 2003; Davis and Benner, 2007; Davis et al., 2009; Benner and Kaiser, 2011). The C/N ratio of labile DOM is generally lower than that of refractory DOM in marine environments (Hopkinson and Vallino, 2005). The preferential removal of N-rich DOM over C-rich DOM has also been documented for riverine DOM (Wiegner et al., 2006; Hendrickson et al., 2007; Wiegner et al., 2009; Islam et al., 2019). However, in this study, DOC concentration decreased while DON concentration did not change after 40 days of dark incubation in samples obtained from the upper and middle reaches (Figure 5). The results of the degradation experiments suggested that DOC decomposed, while DON remained unchanged, causing a decrease in the C/N ratio with the river flow in the upper and middle reaches of the Ishikari River. The possible factors contributing to the different degradations of DOC and DON are discussed in the following subsection. In contrast, both DOC and DON did not decompose during the 40 days of dark incubation in samples obtained from the lower reaches (Figure 5). These results suggest that the decrease in the C/N ratio of DOM with river flow in the lower reaches might be the result of biodegradation of C-rich DOM by large microorganisms (>0.7 μm) that were not present in the bioassay of the study.
Bioassay experiments were conducted to evaluate the bioavailability of DOM in riverine water. The initial DOC concentration was degraded by 17–29% in 40 days at four sites in the upper and middle reaches, but the DOC concentrations did not change significantly at the two downstream sites. This result suggests that the labile DOC in the river is utilized by microorganisms in the upper and middle reaches, whereas the bio-refractory DOC accounts for a large proportion of DOM in the lower reaches. However, the results of this study might underestimate the actual loss of DOC, because large sized microorganisms (>0.7 μm) were removed from the bioassay experiments through filtration. As discussed in the previous subsection, the decrease in the C/N ratio of DOM with river flow in the lower reaches implies that large microorganisms preferentially remove C-rich DOM over N-rich DOM.
The degradation rate of DOC (%) was not significantly correlated with PO4− or DIN concentrations at the six sites (R = -0.49, p = 0.33, and n = 6 for PO4−; and R = -0.55, p = 0.26, and n = 6 for DIN), suggesting that differences in the concentration of inorganic nutrients did not affect the biodegradation of riverine DOC, particularly by small microorganisms (<0.7 μm), in this study.
Microbial degradation of DOC has been observed in previous studies (Wikner et al., 1999; Royer and David, 2005; Wiegner et al., 2009). The bio-degraded DOM at four sites in the upper and middle reaches was probably derived from forest or paddy fields, while bio-refractory DOM at the two sites in the lower reaches might be derived from paddy fields. Although the direct relationship between microbial reactivity and land use land cover has not been extensively studied, Asmala et al. (2013) reported that DOM originating from a catchment dominated by natural forests and peatlands was less biodegradable than that from agricultural areas.
DOM in the lower reaches (Sites 14 and 15) can be characterized as possessing high molecular weight and high aromaticity (Figure 4), which is generally considered to be typical characteristics of bio-refractory materials (Bhattacharya and Osburn, 2020). The biodegradability of DOM has been related to the contribution of protein-like fluorophores to the total fluorophores (Balcarczyk et al., 2009; Fellman et al., 2009; Petrone et al., 2011). Relationships between biodegradability and B/A and B/C, which correspond to the contribution of protein-like fluorophores in total fluorophores, were observed in this study (R = 0.83, p = 0.04, and n = 6 for B/A; and R = 0.82, p = 0.05, and n = 6 for B/C). These results imply that the abundance of protein-like substances may affect the biodegradability of riverine DOC, particularly by small microorganisms, in the Ishikari River.
Notably, no significant change occurred in the DON concentration during the biodegradation experiments at any of the six sites. DON generally degrades during incubation in the dark (Seitzinger and Sanders, 1997; Stepanauskas et al., 2000; Wiegner et al., 2009), and N-rich DOM is generally considered to be more biodegradable than C-rich DOM (Wiegner et al., 2006; Hendrickson et al., 2007; Petrone et al., 2009; Wiegner et al., 2009; Islam et al., 2019). In contrast, no change or increase in DON concentration was observed in several dark incubation experiments conducted previously (Kaushal and Lewis, 2005; Wiegner et al., 2006; Pisani et al., 2017), which was similar to the results of our dark incubation experiments (Figure 5).
The fact that DON concentration did not change but the DOC concentration decreased can be considered to be a case where riverine microorganisms degrade non-N-containing organic compounds but do not decompose N-containing organic compounds. However, this mechanism did not seem to be the case for riverine DOM in this study, because DOC degradability was related to the relative abundance of protein-like fluorophores (peak B), and DON concentration was positively correlated with the abundance of protein-like fluorophores (R = 0.85; Figure 6). These results imply that protein-like fluorophores, which are N-containing organic compounds, were selectively degraded during the dark incubation period.
Another possible explanation for the different behaviors of DOC and DON during the biodegradation experiments is that the decomposition and production of N-containing organic compounds occur at the same extent. Hence, the microorganisms take up N-containing organic compounds with higher C/N ratios and release N-containing organic compounds with lower C/N ratios. This mechanism is consistent with the findings of Pisani et al. (2017), who reported the production of many new N-containing organic compounds during dark microbial incubation of riverine DOM.
This study investigated the spatial distribution of DOC and DON concentrations and the C/N ratio with DOM optical properties in the Ishikari River Basin, and dark incubation experiments were conducted to determine the bioavailability of DOC and DON as a factor controlling their spatial distribution. A major factor influencing the DOC and DON concentrations as well as the optical properties of DOM in the mainstream might be the input of high levels of DOM with high molecular weight and high aromaticity from flooded paddy fields. In addition to the allochthonous input, the input of autochthonous DOM with a low C/N ratio and selective removal of C-rich DOM were suggested to be important factors in decreasing the C/N ratio of DOM with the river flow in the mainstream.
This study discussed the factors determining riverine DOM characteristics based on changes in the DOM concentration and optical properties. However, using optical properties of DOM alone may not be sufficient to identify the origin and dynamics of riverine DOM. The addition of other parameters to optical properties of DOM may lead to improved identification of the origin and dynamics of riverine DOM. For example, the stable carbon isotope (δ13C) of DOC can be used to determine the origin of DOM in rivers (Bhattacharya and Osburn, 2020).
The results of the dark incubation experiments and changes in the C/N ratio of DOM with river flow in the mainstream suggest different roles of small and large microorganisms in the degradation of riverine DOM. The dark incubations of riverine DOM with different sizes of inoculum (Grunert et al., 2010) may clarify the relationships between the degradability of various DOM components and the size of microorganisms. In addition, the role of biofilms at the riverbed in the degradation of riverine DOM should be explored (Battin et al., 2016). The relationship between degradable DOM components involved in the C/N ratio and the type of microorganisms may improve our understanding of riverine DOM as a source of energy and/or organic nutrients in river ecosystems.
The original contributions presented in the study are included in the article/Supplementary Material, further inquiries can be directed to the corresponding author.
Conceptualization: YT, YY, Data curation: YT, KH, and YY, Formal analysis: YT, KH, Funding acquisition: YY, Investigation: YT, KH, and YY, Methodology: YT, KH, and YY, Project administration: YY, Resources: YY, Supervision: YY, Validation: YY, Visualization: YT, Writingoriginal draft: YT, YY, Writingreview and editing: YT, YY.
This research was partly supported by the Japan Society for the Promotion of Science grant KAKENHI JP19H04249 to Y.Y.
The authors declare that the research was conducted in the absence of any commercial or financial relationships that could be construed as a potential conflict of interest.
All claims expressed in this article are solely those of the authors and do not necessarily represent those of their affiliated organizations, or those of the publisher, the editors, and the reviewers. Any product that may be evaluated in this article, or claim that may be made by its manufacturer, is not guaranteed or endorsed by the publisher.
We wish to acknowledge the help of Yuka Sazuka, Soushi Abe, and Kota Ishizaka for their assistance with observations and DOM analysis. We would also like to acknowledge I. Kudo and his lab members for their help with inorganic nutrient analysis. We would like to thank Editage (www.editage.com) for English language editing.
Abe, Y., Maie, N., and Shima, E. (2011). Influence of Irrigated Paddy fields on the Fluorescence Properties of Fluvial Dissolved Organic Matter. J. Environ. Qual. 40 (4), 1266–1272. doi:10.2134/jeq2010.0374
Aitkenhead, J. A., and McDowell, W. H. (2000). Soil C:N Ratio as a Predictor of Annual Riverine DOC Flux at Local and Global Scales. Glob. Biogeochem. Cycles 14 (1), 127–138. doi:10.1029/1999GB900083
Amon, R. M. W., and Benner, R. (1996). Bacterial Utilization of Different Size Classes of Dissolved Organic Matter. Limnol. Oceanogr. 41 (1), 41–51. doi:10.4319/lo.1996.41.1.0041
Amon, R. M. W., Fitznar, H.-P., and Benner, R. (2001). Linkages Among the Bioreactivity, Chemical Composition, and Diagenetic State of marine Dissolved Organic Matter. Limnol. Oceanogr. 46 (2), 287–297. doi:10.4319/lo.2001.46.2.0287
Asmala, E., Autio, R., Kaartokallio, H., Pitkänen, L., Stedmon, C. A., and Thomas, D. N. (2013). Bioavailability of Riverine Dissolved Organic Matter in Three Baltic Sea Estuaries and the Effect of Catchment Land Use. Biogeosciences 10, 6969–6986. doi:10.5194/bg-10-6969-2013
Babin, M., Stramski, D., Ferrari, G. M., Claustre, H., Bricaud, A., Obolensky, G., et al. (2003). Variations in the Light Absorption Coefficients of Phytoplankton, Nonalgal Particles, and Dissolved Organic Matter in Coastal Waters Around Europe. J. Geophys. Res. 108 (C7), 3211. doi:10.1029/2001JC000882
Balcarczyk, K. L., Jones, J. B., Jaffé, R., and Maie, N. (2009). Stream Dissolved Organic Matter Bioavailability and Composition in Watersheds Underlain with Discontinuous Permafrost. Biogeochemistry 94, 255–270. doi:10.1007/s10533-009-9324-x
Battin, T. J., Besemer, K., Bengtsson, M. M., Romani, A. M., and Packmann, A. I. (2016). The Ecology and Biogeochemistry of Stream Biofilms. Nat. Rev. Microbiol. 14, 251–263. doi:10.1038/nrmicro.2016.15
Benner, R., and Kaiser, K. (2011). Biological and Photochemical Transformations of Amino Acids and Lignin Phenols in Riverine Dissolved Organic Matter. Biogeochemistry 102, 209–222. doi:10.1007/s10533-010-9435-4
Bernal, S., Lupon, A., Catalán, N., Castelar, S., and Martí, E. (2018). Decoupling of Dissolved Organic Matter Patterns between Stream and Riparian Groundwater in a Headwater Forested Catchment. Hydrol. Earth Syst. Sci. 22, 1897–1910. doi:10.5194/hess-22-1897-2018
Bertilsson, S., and Jones, J. B. (2003). “Supply of Dissolved Organic Matter to Aquatic Ecosystems,” in Aquatic Ecosystems: Interactivity of Dissolved Organic Matter (San Diego: Academic Press), 3–24. doi:10.1016/b978-012256371-3/50002-0
Bhattacharya, R., and Osburn, C. L. (2020). Spatial Patterns in Dissolved Organic Matter Composition Controlled by Watershed Characteristics in a Coastal River Network: The Neuse River Basin, USA. Water Res. 169, 115248. doi:10.1016/j.watres.2019.115248
Bianchi, T. S. (2011). The Role of Terrestrially Derived Organic Carbon in the Coastal Ocean: A Changing Paradigm and the Priming Effect. Proc. Natl. Acad. Sci. 108 (49), 19473–19481. doi:10.1073/pnas.1017982108
Chen, M., Price, R. M., Yamashita, Y., and Jaffé, R. (2010). Comparative Study of Dissolved Organic Matter from Groundwater and Surface Water in the Florida Coastal Everglades Using Multi-Dimensional Spectrofluorometry Combined with Multivariate Statistics. Appl. Geochem. 25 (6), 872–880. doi:10.1016/j.apgeochem.2010.03.005
Coble, P. G. (1996). Characterization of marine and Terrestrial DOM in Seawater Using Excitation-Emission Matrix Spectroscopy. Mar. Chem. 51 (4), 325–346. doi:10.1016/0304-4203(95)00062-3
Coble, P. G., Del Castillo, C. E., and Avril, B. (1998). Distribution and Optical Properties of CDOM in the Arabian Sea during the 1995 Southwest Monsoon. Deep-Sea Res. Part II-Top. Stud. Oceanogr 45, 10–11. doi:10.1016/S0967-0645(98)00068-X
Connolly, C. T., Khosh, M. S., Burkart, G. A., Douglas, T. A., Holmes, R. M., Jacobson, A. D., et al. (2018). Watershed Slope as a Predictor of Fluvial Dissolved Organic Matter and Nitrate Concentrations across Geographical Space and Catchment Size in the Arctic. Environ. Res. Lett. 13, 104015. doi:10.1088/1748-9326/aae35d
Cory, R. M., and Kling, G. W. (2018). Interactions between Sunlight and Microorganisms Influence Dissolved Organic Matter Degradation along the Aquatic Continuum. Limnol Oceanogr Lett. 3, 102–116. doi:10.1002/lol2.10060
Cory, R. M., and McKnight, D. M. (2005). Fluorescence Spectroscopy Reveals Ubiquitous Presence of Oxidized and Reduced Quinones in Dissolved Organic Matter. Environ. Sci. Technol. 39 (21), 8142–8149. doi:10.1021/es0506962
Cory, R. M., Miller, M. P., McKnight, D. M., Guerard, J. J., and Miller, P. L. (2010). Effect of Instrument-specific Response on the Analysis of Fulvic Acid Fluorescence Spectra. Limnol. Oceanogr. Methods 8, 67–78. doi:10.4319/lom.2010.8.67
Davis, J., and Benner, R. (2007). Quantitative Estimates of Labile and Semi-labile Dissolved Organic Carbon in the Western Arctic Ocean: A Molecular Approach. Limnol. Oceanogr. 52, 2434–2444. doi:10.4319/lo.2007.52.6.2434
Davis, J., Kaiser, K., and Benner, R. (2009). Amino Acid and Amino Sugar Yields and Compositions as Indicators of Dissolved Organic Matter Diagenesis. Org. Geochem. 40 (3), 343–352. doi:10.1016/j.orggeochem.2008.12.003
Drake, T. W., Raymond, P. A., and Spencer, R. G. M. (2018). Terrestrial Carbon Inputs to Inland Waters: A Current Synthesis of Estimates and Uncertainty. Limnol Oceanogr Lett. 3, 132–142. doi:10.1002/lol2.10055
Duan, W. L., He, B., Takara, K., Luo, P. P., Nover, D., and Hu, M. C. (2015). Modeling Suspended Sediment Sources and Transport in the Ishikari River basin, Japan, Using SPARROW. Hydrol. Earth Syst. Sci. 19, 1293–1306. doi:10.5194/hess-19-1293-2015
Duan, W., Takara, K., He, B., Luo, P., Nover, D., and Yamashiki, Y. (2013). Spatial and Temporal Trends in Estimates of Nutrient and Suspended Sediment Loads in the Ishikari River, Japan, 1985 to 2010. Sci. Total Environ. 461-462, 499–508. doi:10.1016/j.scitotenv.2013.05.022
Fellman, J. B., Hood, E., Edwards, R. T., and Jones, J. B. (2009). Uptake of Allochthonous Dissolved Organic Matter from Soil and Salmon in Coastal Temperate Rainforest Streams. Ecosystems 12, 747–759. doi:10.1007/s10021-009-9254-4
Fellman, J. B., Hood, E., and Spencer, R. G. M. (2010). Fluorescence Spectroscopy Opens New Windows into Dissolved Organic Matter Dynamics in Freshwater Ecosystems: A Review. Limnol. Oceanogr 55, 2452. doi:10.4319/lo.2010.55.6.2452
Findlay, S., and Sinsabaugh, R. (2003). Aquatic Ecosystems: Interactivity of Dissolved Organic Matter. J. North Am. Benthological Soc. 23. doi:10.1899/0887-3593(2004)023<0662:AEIODO>2.0.CO;2
Graeber, D., Boëchat, I. G., Encina-Montoya, F., Esse, C., Gelbrecht, J., Goyenola, G., et al. (2015). Global Effects of Agriculture on Fluvial Dissolved Organic Matter. Sci. Rep. 5, 16328. doi:10.1038/srep16328
Grunert, B. K., Tzortziou, M., Neale, P., Menendez, A., and Hernes, P. (2010). DOM Degradation by Light and Microbes along the Yukon River-Coastal Ocean Continuum. Sci. Rep. 11, 10236. doi:10.1038/s41598-021-89327-9
Hasegawa, T., Kasai, H., Ono, T., Tsuda, A., and Ogawa, H. (2010). Dynamics of Dissolved and Particulate Organic Matter during the spring Bloom in the Oyashio Region of the Western Subarctic Pacific Ocean. Aquat. Microb. Ecol. 60, 127–138. doi:10.3354/ame01418
Heinz, M., Graeber, D., Zak, D., Zwirnmann, E., Gelbrecht, J., and Pusch, M. T. (2015). Comparison of Organic Matter Composition in Agricultural versus Forest Affected Headwaters with Special Emphasis on Organic Nitrogen. Environ. Sci. Technol. 49 (4), 2081–2090. doi:10.1021/es505146h
Helms, J. R., Stubbins, A., Ritchie, J. D., Minor, E. C., Kieber, D. J., and Mopper, K. (2008). Absorption Spectral Slopes and Slope Ratios as Indicators of Molecular Weight, Source, and Photobleaching of Chromophoric Dissolved Organic Matter. Limnol. Oceanogr. 53 (3), 955–969. doi:10.4319/lo.2008.53.3.0955
Hendrickson, J., Trahan, N., Gordon, E., and Ouyang, Y. (2007). Estimating Relevance of Organic Carbon, Nitrogen, and Phosphorus Loads to a Blackwater River Estuary1. J. Am. Water Resour. Assoc. 43 (1), 264–279. doi:10.1111/j.1752-1688.2007.00021.x
Hopkinson, C. S., and Vallino, J. J. (2005). Efficient export of Carbon to the Deep Ocean through Dissolved Organic Matter. Nature 433, 142–145. doi:10.1038/nature03191
Inamdar, S., Finger, N., Singh, S., Mitchell, M., Levia, D., Bais, H., et al. (2012). Dissolved Organic Matter (DOM) Concentration and Quality in a Forested Mid-Atlantic Watershed, USA. Biogeochemistry 108, 55–76. doi:10.1007/s10533-011-9572-4
Islam, M. J., Jang, C., Eum, J., Jung, S.-m., Shin, M.-S., Lee, Y., et al. (2019). C:N:P Stoichiometry of Particulate and Dissolved Organic Matter in River Waters and Changes during Decomposition. J Ecology Environ. 43, 4. doi:10.1186/s41610-018-0101-4
Jacobs, S. R., Breuer, L., Butterbach-Bahl, K., Pelster, D. E., and Rufino, M. C. (2017). Land Use Affects Total Dissolved Nitrogen and Nitrate Concentrations in Tropical Montane Streams in Kenya. Sci. Total Environ. 603-604, 519–532. doi:10.1016/j.scitotenv.2017.06.100
Kaushal, S. S., and Lewis, W. M. (2005). Fate and Transport of Organic Nitrogen in Minimally Disturbed Montane Streams of Colorado, USA. Biogeochemistry 74, 303–321. doi:10.1007/s10533-004-4723-5
Krupa, M., Spencer, R. G. M., Tate, K. W., Six, J., van Kessel, C., and Linquist, B. A. (2012). Controls on Dissolved Organic Carbon Composition and export from rice-dominated Systems. Biogeochemistry 108, 447–466. doi:10.1007/s10533-011-9610-2
Kunii, D., and Saito, G. (2009). Relationships between Land Use and River Nutrient in the River Basins of Kitakami River and Ishikari River Using Remote Sensing and GIS. J. Integrat Field Sci. 6, 59–70.
Lara, R. J., Rachold, V., Kattner, G., Hubberten, H. W., Guggenberger, G., Skoog, A., et al. (1998). Dissolved Organic Matter and Nutrients in the Lena River, Siberian Arctic: Characteristics and Distribution. Mar. Chem. 59, 3–4. doi:10.1016/S0304-4203(97)00076-5
Laudon, H., Kohler, S., and Buffam, I. (2004). Seasonal TOC export from Seven Boreal Catchments in Northern Sweden. Aquat. Sci. - Res. Across Boundaries 66, 223–230. doi:10.1007/s00027-004-0700-2
Lawaetz, A. J., and Stedmon, C. A. (2009). Fluorescence Intensity Calibration Using the Raman Scatter Peak of Water. Appl. Spectrosc. 63 (8), 936–940. doi:10.1366/000370209788964548
Lee, S.-A., Kim, T.-H., and Kim, G. (2020). Tracing Terrestrial versus marine Sources of Dissolved Organic Carbon in a Coastal bay Using Stable Carbon Isotopes. Biogeosciences 17, 135–144. doi:10.5194/bg-17-135-2020
Lønborg, C., and Álvarez‐Salgado, X. A. (2012). Recycling versus export of Bioavailable Dissolved Organic Matter in the Coastal Ocean and Efficiency of the continental Shelf Pump. Glob. Biogeochem. Cycles 26, GB3018. doi:10.1029/2012GB004353
Lu, Y. H., Bauer, J. E., Canuel, E. A., Chambers, R. M., Yamashita, Y., Jaffé, R., et al. (2014). Effects of Land Use on Sources and Ages of Inorganic and Organic Carbon in Temperate Headwater Streams. Biogeochemistry 119, 275–292. doi:10.1007/s10533-014-9965-2
Lutz, B. D., Bernhardt, E. S., Roberts, B. J., and Mulholland, P. J. (2011). Examining the Coupling of Carbon and Nitrogen Cycles in Appalachian Streams: the Role of Dissolved Organic Nitrogen. Ecology 92, 720–732. doi:10.1890/10-0899.1
Mann, P. J., Spencer, R. G. M., Hernes, P. J., Six, J., Aiken, G. R., Tank, S. E., et al. (2016). Pan-Arctic Trends in Terrestrial Dissolved Organic Matter from Optical Measurements. Front. Earth Sci. 4, 25. doi:10.3389/feart.2016.00025
McKnight, D. M., Boyer, E. W., Westerhoff, P. K., Doran, P. T., Kulbe, T., and Andersen, D. T. (2001). Spectrofluorometric Characterization of Dissolved Organic Matter for Indication of Precursor Organic Material and Aromaticity. Limnol. Oceanogr. 46 (1), 38–48. doi:10.4319/lo.2001.46.1.0038
Moran, M. A., Sheldon, W. M., and Zepp, R. G. (2000). Carbon Loss and Optical Property Changes during Long-Term Photochemical and Biological Degradation of Estuarine Dissolved Organic Matter. Limnol. Oceanogr. 45, 1254–1264. doi:10.4319/lo.2000.45.6.1254
Mulholland, P. J. (2003). “Large-scale Patterns in Dissolved Organic Carbon Concentration, Flux, and Sources,” in Aquatic Ecosystems: Interactivity of Dissolved Organic Matter (San Diego: Academic Press), 139–159. doi:10.1016/b978-012256371-3/50007-x
Opsahl, S., and Benner, R. (1998). Photochemical Reactivity of Dissolved Lignin in River and Ocean Waters. Limnol. Oceanogr 43, 1297. doi:10.4319/lo.1998.43.6.1297
Petrone, K. C., Fellman, J. B., Hood, E., Donn, M. J., and Grierson, P. F. (2011). The Origin and Function of Dissolved Organic Matter in Agro-Urban Coastal Streams. J. Geophys. Res. 116. doi:10.1029/2010JG001537
Petrone, K. C., Richards, J. S., and Grierson, P. F. (2009). Bioavailability and Composition of Dissolved Organic Carbon and Nitrogen in a Near Coastal Catchment of South-Western Australia. Biogeochemistry 92, 27–40. doi:10.1007/s10533-008-9238-z
Pisani, O., Boyer, J. N., Podgorski, D. C., Thomas, C. R., Coley, T., and Jaffé, R. (2017). Molecular Composition and Bioavailability of Dissolved Organic Nitrogen in a lake Flow-Influenced River in South Florida, USA. Aquat. Sci. 79, 891–908. doi:10.1007/s00027-017-0540-5
R Core Team (2021). R: A Language and Environment for Statistical Computing R Foundation for Statistical Computing. Vienna, Austria. Available at: : https://www.R-project.org/.
Royer, T. V., and David, M. B. (2005). Export of Dissolved Organic Carbon from Agricultural Streams in Illinois, USA. Aquat. Sci. 67, 465–471. doi:10.1007/s00027-005-0781-6
Ruark, M. D., Linquist, B. A., Six, J., van Kessel, C., Greer, C. A., Mutters, R. G., et al. (2010). Seasonal Losses of Dissolved Organic Carbon and Total Dissolved Solids from Rice Production Systems in Northern California. J. Environ. Qual. 39, 304–313. doi:10.2134/jeq2009.0066
Seitzinger, S. P., Sanders, R. W., and Styles, R. (2002). Bioavailability of DON from Natural and Anthropogenic Sources to Estuarine Plankton. Limnol. Oceanogr. 47 (2), 353–366. doi:10.4319/lo.2002.47.2.0353
Seitzinger, S., and Sanders, R. (1997). Contribution of Dissolved Organic Nitrogen from Rivers to Estuarine Eutrophication. Mar. Ecol. Prog. Ser. 159, 1–12. doi:10.3354/meps159001
Smith, P. (2008). Land Use Change and Soil Organic Carbon Dynamics. Nutr. Cycl Agroecosyst 81, 169–178. doi:10.1007/s10705-007-9138-y
Stepanauskas, R., Laudon, H., and Jørgensen, N. O. G. (2000). High DON Bioavailability in Boreal Streams during a spring Flood. Limnol. Oceanogr. 45, 1298–1307. doi:10.4319/lo.2000.45.6.1298
Suzuki, T., Nagao, S., Horiuchi, M., Maie, N., Yamamoto, M., and Nakamura, K. (2015). Characteristics and Behavior of Dissolved Organic Matter in the Kumaki River, Noto Peninsula, Japan. Limnology 16, 55–68. doi:10.1007/s10201-014-0441-4
Tanaka, K., Kuma, K., Hamasaki, K., and Yamashita, Y. (2014). Accumulation of Humic-like Fluorescent Dissolved Organic Matter in the Japan Sea. Sci. Rep. 4, 5292. doi:10.1038/srep05292
Voss, M., Asmala, E., Bartl, I., Carstensen, J., Conley, D. J., Dippner, J. W., et al. (2021). Origin and Fate of Dissolved Organic Matter in Four Shallow Baltic Sea Estuaries. Biogeochemistry 154, 385–403. doi:10.1007/s10533-020-00703-5
Weishaar, J. L., Aiken, G. R., Bergamaschi, B. A., Fram, M. S., Fujii, R., and Mopper, K. (2003). Evaluation of Specific Ultraviolet Absorbance as an Indicator of the Chemical Composition and Reactivity of Dissolved Organic Carbon. Environ. Sci. Technol. 37 (20), 4702–4708. doi:10.1021/es030360x
Westerhoff, P., and Mash, H. (2002). Dissolved Organic Nitrogen in Drinking Water Supplies: a Review. J. Water Supply Res. Technol.-Aqua 51 (8), 415–448. doi:10.2166/aqua.2002.0038
Wetzel, R. G. (1992). Gradient-dominated Ecosystems: Sources and Regulatory Functions of Dissolved Organic Matter in Freshwater Ecosystems. Hydrobiologia 229, 181–198. doi:10.1007/BF00007000
Wiegner, T. N., Tubal, R. L., and MacKenzie, R. A. (2009). Bioavailability and export of Dissolved Organic Matter from a Tropical River during Base- and Stormflow Conditions. Limnol. Oceanogr. 54 (4), 1233–1242. doi:10.4319/lo.2009.54.4.1233
Wiegner, T., Seitzinger, S., Glibert, P., and Bronk, D. (2006). Bioavailability of Dissolved Organic Nitrogen and Carbon from Nine Rivers in the Eastern United States. Aquat. Microb. Ecol. 43, 277–287. doi:10.3354/ame043277
Wiegner, T., and Seitzinger, S. (2001). Photochemical and Microbial Degradation of External Dissolved Organic Matter Inputs to Rivers. Aquat. Microb. Ecol. 24 (1), 27–40. doi:10.3354/ame024027
Wikner, J., Cuadros, R., and Jansson, M. (1999). Differences in Consumption of Allochthonous DOC under Limnic and Estuarine Conditions in a Watershed. Aquat. Microb. Ecol. 17, 289–299. doi:10.3354/ame017289
Willett, V. B., Reynolds, B. A., Stevens, P. A., Ormerod, S. J., and Jones, D. L. (2004). Dissolved Organic Nitrogen Regulation in Freshwaters. J. Environ. Qual. 33, 201–209. doi:10.2134/jeq2004.2010
Williams, C. J., Frost, P. C., Morales-Williams, A. M., Larson, J. H., Richardson, W. B., Chiandet, A. S., et al. (2016). Human Activities Cause Distinct Dissolved Organic Matter Composition across Freshwater Ecosystems. Glob. Change Biol. 22, 613–626. doi:10.1111/gcb.13094
Wymore, A. S., Rodríguez-Cardona, B., and McDowell, W. H. (2015). Direct Response of Dissolved Organic Nitrogen to Nitrate Availability in Headwater Streams. Biogeochemistry 126, 1–10. doi:10.1007/s10533-015-0153-9
Yamashita, Y., Kloeppel, B. D., Knoepp, J., Zausen, G. L., and Jaffé, R. (2011). Effects of Watershed History on Dissolved Organic Matter Characteristics in Headwater Streams. Ecosystems 14, 1110–1122. doi:10.1007/s10021-011-9469-z
Yamashita, Y., Kojima, D., Yoshida, N., and Shibata, H. (2021). Relationships between Dissolved Black Carbon and Dissolved Organic Matter in Streams. Chemosphere 271, 129824. doi:10.1016/j.chemosphere.2021.129824
Yamashita, Y., Nosaka, Y., Suzuki, K., Ogawa, H., Takahashi, K., and Saito, H. (2013). Photobleaching as a Factor Controlling Spectral Characteristics of Chromophoric Dissolved Organic Matter in Open Ocean. Biogeosciences 10 (11), 7207–7217. doi:10.5194/bg-10-7207-2013
Yamashita, Y., Scinto, L. J., Maie, N., and Jaffé, R. (2010). Dissolved Organic Matter Characteristics across a Subtropical Wetland's Landscape: Application of Optical Properties in the Assessment of Environmental Dynamics. Ecosystems 13, 1006–1019. doi:10.1007/s10021-010-9370-1
Yamashita, Y., and Tanoue, E. (2003). Distribution and Alteration of Amino Acids in Bulk DOM along a Transect from bay to Oceanic Waters. Mar. Chem. 82, 3–4. doi:10.1016/S0304-4203(03)00049-5
Yates, C. A., Johnes, P. J., Owen, A. T., Brailsford, F. L., Glanville, H. C., Evans, C. D., et al. (2019). Variation in Dissolved Organic Matter (DOM) Stoichiometry in U.K. Freshwaters: Assessing the Influence of Land Cover and Soil C:N Ratio on DOM Composition. Limnol. Oceanogr 64, 2328–2340. doi:10.1002/lno.11186
Keywords: riverine dissolved organic matter, carbon/nitrogen (C/N) ratio, optical properties, land use land cover, bioavailability, Ishikari River
Citation: Takaki Y, Hattori K and Yamashita Y (2022) Factors Controlling the Spatial Distribution of Dissolved Organic Matter With Changes in the C/N Ratio From the Upper to Lower Reaches of the Ishikari River, Japan. Front. Earth Sci. 10:826907. doi: 10.3389/feart.2022.826907
Received: 01 December 2021; Accepted: 14 February 2022;
Published: 04 March 2022.
Edited by:
Shafi Mohammad Tareq, Jahangirnagar University, BangladeshReviewed by:
Kaijun Lu, University of Texas at Austin, United StatesCopyright © 2022 Takaki, Hattori and Yamashita. This is an open-access article distributed under the terms of the Creative Commons Attribution License (CC BY). The use, distribution or reproduction in other forums is permitted, provided the original author(s) and the copyright owner(s) are credited and that the original publication in this journal is cited, in accordance with accepted academic practice. No use, distribution or reproduction is permitted which does not comply with these terms.
*Correspondence: Yuji Takaki, eXVqaXRha2FraUBlZXMuaG9rdWRhaS5hYy5qcA==
Disclaimer: All claims expressed in this article are solely those of the authors and do not necessarily represent those of their affiliated organizations, or those of the publisher, the editors and the reviewers. Any product that may be evaluated in this article or claim that may be made by its manufacturer is not guaranteed or endorsed by the publisher.
Research integrity at Frontiers
Learn more about the work of our research integrity team to safeguard the quality of each article we publish.