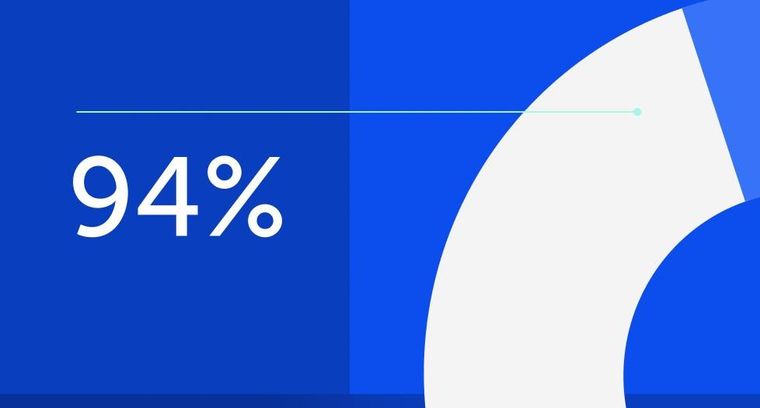
94% of researchers rate our articles as excellent or good
Learn more about the work of our research integrity team to safeguard the quality of each article we publish.
Find out more
ORIGINAL RESEARCH article
Front. Earth Sci., 23 February 2022
Sec. Paleontology
Volume 10 - 2022 | https://doi.org/10.3389/feart.2022.826353
This article is part of the Research TopicPrecambrian PaleontologyView all 13 articles
In the absence of complex, bioturbating organisms, the seafloor during the Precambrian was covered in widespread organic matgrounds. The greatest diversity and complexity of organic mat textures occur in the Ediacaran fossil record as exemplified by the Ediacara Member of the Rawnsley Quartzite, which crops out in and around the Flinders Ranges, South Australia. This succession unambiguously demonstrates that heterogenous mats coexisted with and were central to the ecology and biology of the Ediacara Biota. Excavation of 33 fossiliferous beds with varying types and extents of organosedimentary surface textures provide the opportunity to utilize this record to develop criteria to evaluate the maturity or extent of growth of Ediacaran matgrounds and, using these characteristics, to examine the relationship between mat type, mat maturity and Ediacara Biota community structure. Based on the assumption that mat maturity represents an indicator of the duration of time between burial events, we can test predictions about the relationship between mat maturity and community development. We find that mat maturity, rather than the mat type itself, more strongly influenced the distribution of taxa and the development of Ediacara macroorganism communities. Using a ranked Mat Maturity Index, we find that although density of macroscopic body fossils and genus diversity correlate with mat maturity, evenness does not. We additionally find that the sessile taxa Obamus and Coronacollina are restricted to surfaces with mature mats whereas all other Ediacaran macrobiota show no connection to mat occurrence and maturity. However, we do observe that large Dickinsonia are more likely to occur on surfaces recording mature matgrounds. The exceptional record of mat surfaces preserved in the Flinders Ranges area demonstrates that, in addition to the apparent ecological role played by mat surfaces in Ediacaran communities, they were also likely a significant component of the Ediacara Member biomass and were integral to community function.
The history of life on Earth spans at least 3.5 billion years, the first 2.5 billion years of which were almost exclusively characterized by microscopic, single-celled organisms. Therefore, if we were to visit Earth at a random point in time, we would be unlikely to find the diversity and complexity of macroscopic life that characterizes the biosphere today. In the absence of complex, bioturbating organisms, the Precambrian seafloor was covered by widespread organic matgrounds, consisting of aggregates of primarily microscopic forms. These are readily identified in the geologic record as distinct macroscopic sedimentary structures, features such as “microbially induced sedimentary structures” (MISS; Noffke, 2009)—e.g., microbial laminations and organosedimentary textures preserved on bedding planes—and organic microfossils that together represent definitive evidence of Precambrian life.
The greatest diversity and complexity of organic mat textures in the fossil record occur in successions of Ediacaran age (635–541 Ma; e.g., Bose et al., 2012; Gehling, 1999; Hagadorn and Bottjer, 1999; Porada and Bouougri, 2007; Sarkar et al., 2011). The Ediacaran stratigraphic record is further characterized by textured organic surfaces (TOS; Gehling and Droser, 2009) which consist of organosedimentary textures recording the presence of not only benthic microbial aggregates but also densely packed communities of macroscopic and multicellular eukaryotic organisms, as well as their interaction with hydrodynamic and sedimentary processes. Multicellular matgrounds included “thickets” of macroscopic, eukaryote-grade tubular organisms such as Funisia dorothea (Droser and Gehling, 2008; Surprenant et al., 2020). TOS thus refers to the sedimentological expression of these matgrounds and the processes impacting them.
Heterogenous mats coexisted with and were central to the ecology and biology of the Ediacara Biota, the oldest macroscopic community-forming organisms in the fossil record (e.g., Xiao and Laflamme, 2009; Droser and Gehling, 2015). Thus, the Ediacaran occupies a unique time in Earth history wherein the earliest complex macrofauna and matgrounds are observed occupying the same environments. Such matgrounds played a pivotal role in rendering the seafloor habitable for the Ediacara Biota, by stabilizing seafloor sediments and providing food and potentially oxygen (e.g., Seilacher, 1999; Gingras et al., 2011; Droser et al., 2017; Gehling and Droser, 2018; Evans et al., 2019; Evans et al., 2020). Furthermore, the organically-stabilized substrate also shaped the sedimentologic and stratigraphic expression of Ediacaran successions by binding sediment, which inhibited reworking and erosion and facilitated the capture of a complete sedimentary and stratigraphic record (Noffke, 2009; Tarhan et al., 2017).
The Ediacara Member of the Rawnsley Quartzite, which crops out throughout the Flinders Ranges area (South Australia), contains the most diverse assemblage of the Ediacara Biota (Droser et al., 2006; Darroch et al., 2015; Droser and Gehling, 2015; Droser et al., 2019) and sedimentary structures indicative of widespread, heterogenous mats (Figures 1–3; Gehling and Droser, 2009). At the Nilpena Ediacara National Park (NENP), excavation of 40 bedding planes, 33 of which preserve >10 individual macroscopic body fossils, reveals an unprecedented abundance and diversity of in situ macroorganism communities and associated microbial mats. Many of the bedding-plane features that are central to defining MISS and TOS (e.g., preservational variability, bed-scale diversity, associated macrobiota, grain size, mineralogy, chemical composition, and sedimentary structures) have previously been described (e.g., Bottjer et al., 2007; Bouougri and Porada, 2007; Carbone and Narbonne, 2014; Corenbilt et al., 2019; Elliott et al., 2011; Hill et al., 2016; Kumar and Ahmad, 2014; Laflamme et al., 2012; Liu et al., 2013; Nettle et al., 2014; Nofke, 2015; Noffke et al., 2002; Noffke, 2009; Sarkar et al., 2008, Sarkar et al., 2016; Tarhan et al., 2017; Vago et al., 2017). Here our aim is to use this record to develop criteria to evaluate the maturity or extent of growth of Ediacaran matgrounds and, using these characteristics, to examine the relationship between mat type and maturity and Ediacara Biota community structure.
FIGURE 1. Textured organic surfaces (TOS) on excavated beds from the Ediacara Member and the informal Nilpena Member. (A) TOS on bedding plane TB-ARB. (B) Elephant skin TOS. (C) TOS on bedding plane 1T-F-Annex. (D) TOS on bedding plane WS-Sub. (E) Weave on TC-MM3 bedding plane. (F) Helminthoidichnites preserved in hyporelief on the bedding plane of TB-Sh2. Scale bars = 5 cm.
FIGURE 2. Distinct mat textures preserved in hyporelief on bedding plane LV-Fun. (A) Clotted TOS. (B) Foam TOS. (C) Hair TOS. (D) Hair and clotted TOS in distinct patches with sharp transitional boundaries. Scale bars = 5 cm.
FIGURE 3. Complex TOS and macrofossils associated with TOS on excavated beds. (A) Complex TOS created by Plexus ricei aggregates on bed P-SNG1. (B) Complex TOS produced by a densely packed population of Funisia dorothea on WS-MAB annex surfaces (C) Dickinsonia body fossil draped over the complex TOS “groove” on STC-X bed. (D) Dickinsonia body fossil overlying undefined TOS on bedding plane TB-ARB. (E) Dickinsonia body fossil overlying TOS and associated Dickinsonia footprints on TB-BRW bed. (F) Obamus coronatus fossil (left) and putty mold (right), showing that the organism was embedded within the TOS on LV-Fun bed. Scale bars = 5 cm.
In the Flinders Ranges area of South Australia, the Ediacara Member of the Rawnsley Quartzite consists of 10–300 m of shallow marine deposits that are characterized by dense and well-preserved fossil assemblages of the Ediacara Biota (Gehling, 2000). A new unit, the Nilpena Member, characterized by a basal incision, has been informally described at NENP (Gehling et al., 2019) and includes the upper tens of meters of what was previously included in the Ediacara Member. Within these two units, benthic communities of the Ediacara Biota and organic matgrounds were preserved by episodic obrution events as casts and molds on the bases of sandstone beds (Gehling, 1999). At NENP, exposures of the Ediacara Member and the informal Nilpena Member have uniquely facilitated the excavation and reconstruction of 40 discrete bedding planes preserving evidence of both macroscopic organisms and widespread organic surfaces. These bedding planes represent, in total, approximately 350 square meters of Ediacaran seafloor, permitting the spatially broad and stratigraphically detailed reconstruction of snapshots of Ediacara communities. Importantly, each bed can be correlated with a cross-sectional reference in outcrop, including bed junctions within a stratigraphic package.
Four facies at NENP are fossil rich and preserve variable depositional environments (Gehling and Droser, 2013; Droser et al., 2019), two of which are readily excavated for bedding-plane analysis. The Oscillation-Rippled Sandstone (ORS) Facies of the Ediacara Member is particularly relevant for deciphering in situ relationships between the organic mat and macroscopic organisms of the Ediacara Biota. The ORS Facies consists of mm- to cm-scale beds of symmetrically rippled to interference-rippled, fine- to coarse-grained sandstone representing deposition in relatively shallow water near to above fair-weather wave base (Gehling and Droser, 2013; Tarhan et al., 2017). Over 30 excavated beds at NENP are from this facies and preserve classic members of the Ediacara Biota (e.g., Dickinsonia) and abundant, diverse TOS predominantly as external molds on the bases of beds. Within the informal Nilpena Member, the Planar-Laminated and Rip-Up Sandstone (PLRUS) Facies consists of laterally continuous, planar-laminated, fine-grained sandstone beds on which fossils and TOS similarly occur primarily as hyporelief external molds. This facies represents the upper fill of a sub-wave base incision, deposited under unidirectional flow (Gehling and Droser, 2013; Tarhan et al., 2017). There are six excavated beds from this facies. A particularly unusual characteristic of both the ORS and PLRUS Facies is the lack of mud-sized sediment (Gehling, 1999; Gehling and Droser, 2013; Tarhan et al., 2017; Droser et al., 2019); the fossiliferous ORS and PLURS successions discussed here are completely composed of very fine-to coarse-grained sandstone.
The Phanerozoic record of marine siliciclastic sedimentary rocks is typically characterized by bed junctions that are erosive, graded (e.g., sandstone overlain by mudstone) or bioturbated. Sequential beds of similar grain size are typically amalgamated. During the Ediacaran, in contrast, sediment mixing by bioturbation was largely absent and the organic binding provided by mats inhibited sediment reworking and erosion, as well as providing a diagenetic interface for authigenic mineralization via the precipitation of silica cements, promoting lithologic separation (Noffke, 2009; Tarhan et al., 2016; Tarhan et al., 2017).
There are a number of distinct sedimentary features that occur within the Ediacara Member and the informal Nilpena Member as a result of abundant, heterogeneous organic surfaces, as discussed in detail by Tarhan et al. (2017). Widespread organic surfaces, which acted as stabilizing barriers between successive depositional events, also resulted in: 1) the preservation of extremely thin (sub-mm- to mm-scale) beds, which we refer to as shims; 2) textural uniformity, including lack of disparity in grain size, between adjacent beds; 3) lack of Phanerozoic-style amalgamation; 4) lack of erosional bed junctions; and 5) palimpsest ripples or doubly rippled bedforms defined by rippled bed tops and bases which crisply cast the tops of underlying rippled beds (Tarhan et al., 2017; Droser et al., 2019). In particular, the lack of amalgamation between beds of similar grain sizes and lithologies, including some as thin as a millimeter (Tarhan et al., 2017) as a result of organic mats is a striking feature associated with these widespread organic surfaces, and a sedimentological signature that is rarely found in the Phanerozoic record (Tarhan et al., 2017). Furthermore, in the ORS and PLRUS Facies, because the mats served as organic dividers between events, there is little to no erosional reworking or amalgamation, even between successive beds of similar grain size—resulting in a uniquely high-resolution record of each successive depositional event.
Thus, the unusual paucity of erosional loss or unconformities within these facies facilitates the capture of not only a complete record of depositional events but also of the organic mat and macrobiota ecosystem that developed between these depositional events. This is fundamentally unlike the Phanerozoic sedimentological record, where upper layers of sediment are commonly reworked, if not eroded, and texturally uniform layers are typically amalgamated, and fossil assemblages are commonly time-averaged. The events—both biological and physical—that happen between depositional events are likewise typically not preserved.
The majority of individual beds in the ORS Facies are shims of <5 mm (and commonly 1–2 mm) in thickness (Figure 4; Tarhan et al., 2017) and laterally discontinuous. These shims are typically characterized by textured organic surfaces of varying types and stages of development. While we are able to identify and characterize and even excavate these shims, they do not commonly preserve macroscopic body fossils, likely because the extreme thinness of shims relative to Ediacara epimat organisms resulted in onlapping of shims along the margins of macroorganisms rather than complete casting (Tarhan et al., 2017). In some cases, shims contain holes where macroorganisms were present and the macroorganisms are in turn cast by a thicker bed overlying the shim (Figures 4, 5). An example of this is the relationship between STC-G and STC-G Shim (Figures 4, 5). However, shims commonly contain assemblages of the undermat-mining trace fossil Helminthoidichnites (Tarhan et al., 2017; Gehling and Droser, 2018). Thus, most depositional events were not lethal for Ediacara macroorganisms but they did commonly smother the mat surface.
FIGURE 4. Images of bed STC-G and STC-G Shim. (A) Excavated and flipped sole surface of STC-G (left) and top bedding surface of shim underlying STC-G. (B) Image of the sole surface of STC-G with STC-G Shim still in place, red arrows denote macrofossils from the underlying bed that were not cast by STC-G Shim but were cast by the thicker STC-G. Scale bars = 50 cm. (A) Modified from Tarhan et al. (2017).
FIGURE 5. Schematic of depositional scenarios with thick sediment packages and with thin sediment packages or “shims”. (A) Immediately after deposition, the uppermost sediments are rippled and have not yet developed TOS. (B) Over an ecologically significant period time, TOS develops on the sediment package and Ediacara macrobiota begin to colonize the substrate. (C) A thick sediment package is deposited on top of the TOS and macrobiota community, representing final burial of this surface. (D) The resulting fossil surface is preserved on the base of the thick depositional event and preserves TOS associated with Ediacara macrofossils on one surface. (E) An alternate scenario wherein the TOS macrobiota community is overlain by a thin “shim” of sediment that does not completely bury sessile organisms such as Tribrachidium and allows mobile organisms such as Dickinsonia to escape. (F) Over an ecologically significant period of time this shim develops TOS and partially buried sessile organisms and unburied mobile organisms continue to live. (G) A thick sediment package is deposited on top of the shim so that the underlying community is completely buried. (H) The resulting fossil surface preserves both the shim and the overlying thick sediment package as discrete surfaces. The shim preserves the TOS from the original community and holes in the shim are left where organisms were partially buried. The thick sediment package preserves the macrofossils of organisms that were living on top of the shim and that were partially partially buried or onlapped by the shim.
The development of the mat surface itself is a function of the time between depositional events, and is unrelated to either the thickness of the underlying bed upon which the mat grew or that of the overlying bed which casted the mat surface. Thus, as would be expected, shims can and do record textural evidence of well-developed mats even where they have no or few body fossils. We are mainly concerned with relationships between mats and discrete macrobiota, and so, because shims do not readily preserve body fossils, the focus of this study is on excavated beds on the order of centimeters in thickness.
Under the unique conditions of the Ediacaran matground-dominated seafloor, the amount of time between depositional events would have had a considerable impact on the matground maturity of each preserved bed as well as the community of organisms that inhabited that surface. Studies of modern matgrounds demonstrate that relatively mature mats can develop on the order of weeks (Doemel and Brock, 1977).
Sedimentological characters, including TOS, provide three main criteria for evaluating mat maturity independent of associated body fossils (Table 1). The primary indicator of organically mediated sedimentology at NENP is the extent of adherence between successive beds (Tarhan et al., 2017; Figure 4). We infer that limited matground development, e.g., the presence of only a very thin biofilm, resulted in moderate adherence of adjacent beds whereas development of a thicker organic surface resulted in very low adherence between adjacent beds (Tarhan et al., 2017; Droser et al., 2019). Second, in the ORS Facies, beds characterized by a thin biofilm are indicated by relatively sharply defined ripples, whereas surfaces that were colonized by thicker and more mature mats are characterized by muted ripples (Figure 4), presumably due to both overgrowth of previously formed ripples and inhibition of additional reworking and ripple accretion (Tarhan et al., 2017; Droser et al., 2019). As such, the clarity and profile of ripples across a bed surface (both part and counter-part) and in cross-section are a useful measure of mat development. Examination of TOS on these surfaces also provides an indication of maturity. Third, high surface relief of TOS, and the presence of distinct iterative structures likely reflects relatively high matground maturity and duration of growth (Figures 1–3). Maturity does not appear to be associated with a particular type of matground (i.e., a particular type of TOS), but greater maturity may have facilitated more variability in growth morphologies or taphonomic morphologies resulting from matground-sediment and matground-current interactions, as recorded by TOS variability across a given bedding-plane surface. Using these three criteria, we evaluate mat maturity, denoted as Mat Maturity Index (MMI), on all excavated beds containing macroscopic body fossils, on a relative scale from 1 to 4 (Table 2 and Figures 1–3, Figure 6). Beds assigned a MMI value of 1 are considered the least mature, as evidenced by substantial adhesion to underlying beds and the presence of well-defined ripple crests (Figures 4, 6). These beds also contain no visible TOS. Two fossiliferous beds (Table 2) are classified as Mat Maturity Index 1. A MMI ranking of 2 was applied to those beds with clearly defined ripples but no adhesion. While these may include visible TOS, it is typically in discrete, isolated patches and consists of relatively low-relief structures. Beds with a MMI of 3 are characterized by muted ripple crests, no adhesion and visible TOS. MMI 4 is indicated by no adhesion, notably muted ripples and heterogeneous TOS that entirely covers the surface, with locally high relief (Figures 1–3).
TABLE 2. Bed data for taxa and mats. N is the number of discrete body fossils. Dominant TOS type indicated by M micropucker, E elephant skin, W weave, C clotted, F foam, H hair, I complex irregular, F Funisia, A algae and P Plexus. NA is no visible mat structure. Beds with more than one type of TOS are indicated by the appropriate combination of letters. Mat Maturity Index is based on rankings from Table 1. Within each site, beds are listed in stratigraphic order with the younger at the top. The stratigraphic relationship between sites is not known.
FIGURE 6. Schematic of mat development over time (T1-T5) and its associated sedimentological and ecological impacts. Thickness of the “Ripple muting” and “Adhesion” triangles corresponds to the intensity of the variable, i.e., the thickest part of each triangle represents strong adhesion or ripple muting whereas the thinnest part represents weak adhesion or rippling muting. T1 represents a newly deposited sediment package with newly formed ripples and no mat, this surface would exhibit high adhesion and no ripple muting. T2 represents the same surface after a short period of time during which it has developed an immature mat, adhesion is moderate and ripples are only slightly muted. The same surface in T3 has a higher maturity mat and has begun to be colonized by mobile and sessile macrobiota, adhesion is moderate-to-weak and ripples are moderately muted. T4 illustrates that mat continues to develop and become more complex in texture and the community of Ediacaran macroorganisms becomes larger and more diverse, adhesion is weak and ripple muting is strong. T5 represents a mature surface that has been exposed for a long period of time, illustrating a thick and texturally diverse mat that is populated by a high-density and high-diversity community of macrobiota, at this stage there is no adhesion and ripple muting is strong.
Modern microbial mat ecosystems contain diverse consortia of primarily microscopic organisms that vary in composition and texture forming a variety of surface expressions (e.g., Gomes et al., 2020). The physical characteristics, growth rates and community composition of modern microbial mats has been demonstrated to vary significantly based on environmental factors such as irradiance and nutrient concentrations (Hawes et al., 2016; Stal et al., 1985). Examination of TOS from the Ediacara Member and informal Nilpena Member indicates that mat surfaces were remarkably diverse in preserved physical expression, widespread, and heterogeneously distributed across the Ediacaran seafloor (Figure 1; Droser et al., 2006; Droser et al., 2019; Gehling and Droser, 2009; Tarhan et al., 2017). Variation in TOS within and between beds is a function of the composition of the organic surface, the physical energy of the environment and duration of time between episodes of disturbance and final burial. Thus, specific textures do not necessarily correspond to discrete microbial communities or taxa but are also a product of energy, chemistry and both underlying and overlying lithology (Noffke et al., 2002; Sarkar et al., 2008; Bose et al., 2012; Mariotti et al., 2014; Sarkar et al., 2016; Kovalchuck et al., 2017). TOS are preserved on the bases of beds in positive and negative hyporelief, in the same manner as body fossils (Gehling and Droser, 2009; Tarhan et al., 2016).
TOS can be categorized using three primary characteristics: 1) regular or irregular patterning; 2) the size of patterning and 3) relief—largely positive, negative, or mixed (Table 3). While many TOS surfaces are not readily categorized into distinct morphotypes, there are a number of recurring TOS fabrics in the Ediacara and Nilpena members (Figure 1). A number of textures have been described previously from the Ediacara Member (Gehling and Droser, 2009) and here we describe several more. These are summarized in Table 3. The well-known “elephant skin” is preserved as very fine networks of sharp reticulating grooves with patterns of triple-junctions, sharp grooves, and triangular pits (Figure 1B). Coarse “elephant skin” may appear like micro-load casts (Gehling and Droser, 2009). “Pucker” is another commonly occurring TOS which consists of closely spaced, equal-sized dimples and pits (Gehling and Droser, 2009)—which, when occurring on the order of a mm, are referred to as “micropucker” (Figure 1D). “Weave” consists of subparallel to parallel rectilinear elements branching at acute angles, occurring in patches with minimal relief (Figure 1E) (Tarhan et al., 2022).
We add to this list three more distinct textures. Areas of variably sized but aggregated, sub-rounded to oval-shaped high-relief mounds with thick grooves on bed soles are referred to as “clotted” (Figure 2A). “Foam” consists of highly regular mm-scale uniformly sized circular positive-relief domes (Figure 2B). “Hair” consists of long—up to tens of centimeters—sub-mm- to mm-scale strands commonly occurring in bunches and locally twisted (Figure 2C). Elephant skin, clotted and foam are preserved largely in positive hyporelief, reflecting local depressions in the mat surface, at a scale ranging from millimeters to centimeters. These patterns may reflect the presence of a near uniform reticulate pattern in the case of elephant skin, burst bubbles in the case of foam (Eriksson et al., 2007) or pinnacles or tufts in the case of clotted (Gerdes, 2007).
Many TOS surfaces likely represent multiple generations of “microbial” mats and/or local patchiness in mat community composition. These mats commonly co-occurred with (and may have also hosted) multicellular organisms such as algae. Definition of distinct morphotypes for these TOS is challenging but they appear to typically record the most mature mats, as indicated by the Mat Maturity Index criteria outlined above and in Table 1 and are therefore instead grouped together as “irregular complex TOS” (but likely represent a range of organisms and communities, as well as interactions with hydrodynamic and sedimentary processes). These types of TOS are exhibited on beds 1T-F and TB-ARB (Figures 1B,C). The rheology of the upper sediment surface and mat may also have impacted the expression of resulting TOS. This is exemplified by bed WS-Sub where micropucker (Gehling and Droser, 2009) appears to have been formed on a malleable rather than stiff upper mat surface (Figure 1D). Many beds exhibit variable TOS across the surface, particularly those beds that have a MMI ranking of 3 or 4. LV-Fun exemplifies this with distinct patches of clotted and foam as well as hair TOS (Figure 2), in places demarcated by very sharp boundaries (Figure 2D). Additionally, LV-Fun is also locally characterized by irregular complex TOS.
The organic substrates inferred to have covered vast stretches of the Ediacaran seafloor are commonly referred to as “microbial mats” because modern mats typically consist of microscopic, predominantly single-celled organisms. However, modern organic substrates comprised of macroscopic, multicellular taxa have also been described (e.g., “spicule mats” such as that described by Morganti et al., 2021) and densely aggregated multicellular organisms formed widespread matgrounds in the past (Gehling and Droser, 2009) (Figures 3A,B). While multicellular organisms likely comprised part of the consortia recorded by many varieties of TOS at NENP, fossiliferous surfaces also contain TOS that are dominated by the textural remnants of specific macroscopic multicellular taxa. The most widespread example of these is Funisia—a straight or curved hollow tubular macrofossil up to 30 cm long and 12 mm wide with a circular cross-section and uniform diameter, consisting of sub-rectangular modules ranging from 6 to 8 mm long (Droser and Gehling, 2008). Funisia stood upright on the seafloor in great density, estimated to be as high as 6,000/m2 and is, by an order of magnitude, the most abundant fossil at NENP (Surprenant et al., 2020). Upon death, Funisia fell to the seafloor, commonly in loosely oriented aggregations, potentially as a result of lethal perturbation by high-energy currents. In some cases, these felled tubes were buried without further colonization of the surrounding seafloor, whereas in others the Funisia organic surface was subsequently colonized by another community of Ediacara macroorganisms (Figure 7).
FIGURE 7. Schematic of the two scenarios through which complex Funisia dorothea TOS is hypothesized to have formed. (A) A living community of densely packed individuals of Funisia. (B) Death of the Funisia population in the absence of sediment deposition, creating a thick TOS. (C) The Funisia population is buried soon after death, resulting in the casting of the Funisia TOS on the base of the depositional event. (D) In an alternate scenario, the dead Funisia population remains unburied on the seafloor for an ecologically significant period of time, background sedimentation occurs and mobile organisms begin to colonize the surface. (E) The dead Funisia population remains exposed on the seafloor and Funisia begin to decay while new TOS begins to form on top. More macroorganisms, including sessile taxa, begin to colonize the decaying Funisia surface. (F) The fossil surface resulting from the burial of this time averaged Funisia surface preserves the TOS “Groove”, representing the build up of sediment and TOS between decaying Funisia associated with a community of mobile and sessile macroorganisms.
Funisia completely covers four of the excavated beds (WS-MAB, TC-MM2, STC-AB and STC-X) and is very common on two additional beds (LV-Fun, TB-BRW). On three of these surfaces (WS-MAB, TC-MM2, STC-AB), Funisia is distinctively associated with dense assemblages of Aspidella, the discoidal holdfast of a frondose organism such as Arborea. These Aspidella-Funisia assemblages are so distinctive of the Ediacara and Nilpena members (occurring in both the ORS and PLRUS Facies) that they have been inferred to reflect an ecological association or biofacies (Tarhan et al., 2015). Moreover, the presence of Funisia aggregations appears to have also directly influenced the preservation of associated Aspidella and thus represents a taphofacies—where the latter occur with Funisia aggregations, they are characteristically preserved along bed bases as convex internal molds of the pedal (basal) surface of the holdfast, whereas Aspidella occurring in the absence of Funisia aggregations are characteristically preserved along bed bases as external and composite molds of variable relief of the upper surface of the holdfast and, in cases, the associated stem (Tarhan et al., 2015) or are entirely removed, leaving the biogenic tool mark described as mop (Tarhan et al., 2010; Tarhan et al., 2015). Bed STC-X, in contrast, contains abundant Funisia associated with a more variable macrofossil assemblage. The pervasive Funisia TOS on this bed indicates that a dense assemblage of Funisia once populated this surface. However, following the death and collapse of the Funisia community, this patch of seafloor presumably remained unburied on the surface for a sufficiently long period of time that Dickinsonia, Tribrachidium and other epimat macroorganisms began to colonize the organic substrate provided by dead and decaying Funisia (Figure 3B, Figures 7D–F). The draping and distortion of Dickinsonia overlying palimpsests of toppled Funisia on this surface (Figure 3C) reveal that a dense community of Funisia initially populated this surface and, once toppled, created a unique albeit topographically irregular organic substrate that allowed Dickinsonia and other taxa to colonize the area. STC-X provides an example of time-averaging of two distinct communities preserved on an individual bed. Critically, however, this time-averaging occurred on an ecological (and likely sub-annual) time scale. Even on beds where Funisia does not cover the bed surface and was simply a component of the local community, it still appears to have contributed significantly to matground and organic substrate development, and would have provided an organic interface separating successive sandy deposits and was thus not only ecologically but also sedimentologically and taphonomically important (Tarhan et al., 2015; Droser et al., 2019).
Plexus was a broadly curved, elongate tubular organism that resided in a prone orientation on the Ediacaran seafloor. Plexus individuals range in size from 5 to 80 cm long and 5–20 mm wide, and have two main components: a rigid median structure and a fragile, commonly collapsed outer wall (Joel et al., 2014). Plexus is preserved as an external mold on bed soles, and as a counterpart cast on bed tops in sandstones of the ORS Facies. While Plexus is recognized as isolated individuals elsewhere, on three successive beds at NENP it covers the surface and dead or decaying Plexus served as an organic mat base-layer that was subsequently colonized by other organisms. Similar to Funisia on bed STC-X, these likely represent two ecologically time-averaged communities (Figure 3A; Joel et al., 2014), for example on P-P bed, where abundant Plexus are overlain by Dickinsonia, Aulozoon and Phyllozoon.
Densely aggregated fossil algae produced an organic surface on 1T-BOF. In this case, several genera and species of algae (Xiao et al., 2020) cover the bed. The association of particularly small individuals of Dickinsonia and Spriggina with algal macrofossil aggregations on 1T-BOF suggests that these dense and diverse algae served as a nursery preferentially colonized by juvenile macrobiota (Droser et al., 2019).
All of the beds with a TOS composed of multicellular organisms exhibit a MMI of 4 based on the criteria described above; these surfaces are characterized by bed-covering, commonly high-relief TOS; ripples are present but muted and successively excavated beds are not characterized by adhesion (Figures 3A–C).
The environments created by widespread matgrounds in the Ediacaran played a significant role in the distribution of early animals. Fossil evidence suggests that Ediacaran organisms were specifically adapted to these substrates, living in and relying upon resources provided by matgrounds (Seilacher, 1999; Gehling and Droser, 2009, Gehling and Droser, 2018; Gehling et al., 2005, Gehling et al., 2014; Sperling and Vinther, 2010; Evans et al., 2019; Evans et al., 2020). Oxygen concentrations are thought to have been highly variable throughout the Ediacaran, and estimates suggest that they were typically much lower than in similarly energetic, shallow marine modern environments (e.g., Sperling et al., 2015; Reinhard et al., 2016; also reviewed by; Droser et al., 2017; Evans et al., 2018a; Cole et al., 2020; Reinhard and Planavsky, 2021). The activity of photosynthetic organisms living in mat communities would have produced oxygen and pockets of oxygen trapped along mat interfaces may have thus created more habitable microenvironments (e.g., Gingras et al., 2011). Although less stringently limited by oxygen, similar ecological patterns are observed in the modern in communities where photosynthetic microbial mats are responsible for a large percentage of local primary production (Almela et al., 2019). In short, the ecologies of the oldest fossil animals were intimately linked to the unique substrates of the Ediacaran (Droser et al., 2017).
The majority of Ediacara Biota macroorganisms were sessile taxa living on or embedded in the mat. For example, Obamus and Coronacollina both lived embedded in the upper surface of the mat rendering the outer edge of the organism, in places, indistinguishable from the mat (Clites et al., 2012; Dzaugis et al., 2019). Tribrachidium specimens have relatively distinct edges but lived slightly embedded in the mat as demonstrated by the preservation of their basal surfaces as slight divots where specimens had been removed by current or death (Fedonkin, 1985; Hall et al., 2015). The frond holdfast Aspidella provides the best example of anchoring within the mat as discussed above.
Trace fossil evidence suggests that several Ediacaran organisms fed on abundant organic matter found in these matgrounds (Gehling et al., 2005, 2014; Sperling and Vinther, 2010; Gehling and Droser, 2018; Evans et al., 2019, Evans et al., 2020; Xiao et al., 2019). Four Ediacara taxa—Dickinsonia, Ikaria, Kimberella and Yorgia—left trace fossil evidence of their grazing and mobility (Gehling et al., 2005, Gehling et al., 2014; Ivantsov, 2009, Ivantsov, 2011; Evans et al., 2018b, Evans et al., 2019, Evans et al., 2020). Because Ediacaran matgrounds were widespread and interlaminated with sediment (and may have also been rich in sediment), in order to produce a preservable trace fossil these organisms had to actively disrupt the mat surface (Droser et al., 2017; Evans et al., 2019). In order for these traces to be successfully preserved as trace fossils, this excavated area would then need to be buried and molded by the overlying sand before re-growth of the mat obscured the traces of these organisms’ activities. Indeed, a comparison of Dickinsonia body fossil and “footprint” sizes reveals that footprints are, on average, significantly larger than body fossils, suggesting that, at least in part, small footprints were more readily obscured by mat growth (Evans et al., 2019). The close association between the leveed trace fossil Helminthoidichnites (preserved in both concave and convex relief, even along individual traces, and both bed tops and bases) and particularly thin shims and thinner portions of shims (Gehling and Droser, 2018) suggests that the Helminthoidichnites tracemaker or tracemakers (e.g., Ikaria, cf. Evans et al., 2020) grazed not only on exposed upper surfaces of the mat, but also traced nutrient-rich mats underlying thin sand veneers (Figure 1F). Other taxa, including Andiva and Spriggina, may have also been mobile but are not associated with evidence of disruption of the local organic mat surface, potentially suggesting a different feeding mode (Evans et al., 2019). Assemblages of aligned Parvancorina (themselves aligned with other paleocurrent indicators) have been interpreted to reflect a rheotactic lifestyle for Parvancorina (Coutts et al., 2018; Paterson et al., 2017) but these organisms do not appear to have visibly impacted the underlying mat during such activity.
The large number of beds and distribution of different types of TOS provide an opportunity to examine the potential association of specific TOS types and certain macrofossil taxa. Surprisingly, there is only one example of such an association: Funisia matgrounds and the holdfast form genus Aspidella, as discussed above. Other common taxa such as Dickinsonia, Tribrachidium, Spriggina, Rugoconities and Parvancorina do not preferentially occur with specific TOS types.
Mat maturity, rather than the mat type itself, however, may have more strongly influenced the distribution of taxa and the development of Ediacara macroorganism communities. Based on the assumption that mat maturity represents an indicator of the duration of time between burial events, we can test several predictions about the relationship between MMI and community development: 1) diversity, evenness and macrofossil density should correlate with mat maturity and 2) the range of body sizes within a species on individual beds should increase with mat maturity.
Of common taxa that occur on more than three beds, only two, Obamus and Coronacollina, are restricted to surfaces with a high MMI ranking. Obamus occurs only on LV-Fun, TB-ARB, 1T-F and 1T-NA, all with MMI values of 4. Coronacollina similarly occurs on beds with MMI values of 4 and only a single bed with an MMI of 3, 1T-LS. All other common taxa occur on beds with MMI values of 2, 3 and 4. Because there are only two fossiliferous beds (i.e., beds containing macroscopic body fossils) with an MMI value of 1, it is difficult to evaluate the significance of low mat maturity for the distribution of taxa. However, STC-I contains a number of common taxa, including Dickinsonia, Rugoconites and Aspidella (Figure 8). Thus, with the exception of Obamus and Coronacollina, mat maturity does not appear to have dictated the distribution of taxa.
FIGURE 8. Pie charts illustrating the community composition and relative abundance of bedding planes STC-I, TC-MM3, 1T-F, and 1T-T, all from the ORS Facies.
Fossil density appears to be the metric that is most robustly correlated with mat maturity. Fossil density in non-multicellular TOS surfaces across all taxa, including taxonomically diverse assemblages, ranges from 2.5 to 95 individuals per square meter (Table 2 and Figure 9B). Fossil density does not take into account body size. However, with the notable exception of Dickinsonia, discussed below, the range of body sizes—millimeters to <5 cm in length—observed in most individuals of the most common taxa is small enough to have had minimally impacted density.
FIGURE 9. Box and whisker plots demonstrating how various paleoecological metrics correspond to mat maturity. M = multicellular TOS. (A) Number of genera. (B) Density (number of organisms/meter2). (C) Shannon Diversity. (D) Simpson Evenness. All beds with multicellular TOS have a MMI of 4. However, they are unique in that they do not necessarily represent a similar amount time between depositional events as MMI 4 beds dominated by non-multicellular organisms. Thus, these beds are not included with the other MMI 4 beds in this figure but are illustrated separately.
The number of described genera on individual beds excavated at Nilpena range from 2 to 15 (Table 2 and Figure 9A). Overall, the highest number of genera occur on beds with an MMI of 3 or 4 (excluding those TOS formed by aggregated macroscopic multicellular organisms) (Figure 9A). We also calculated Shannon diversity indices (H) which incorporate both the number of species and also the evenness of their abundance. The distribution of Shannon diversity indices does not appear to correlate with MMI, regardless of whether only “microbial”-grade TOS or all TOS types, including aggregations of multicellular organisms such as Funisia, are considered (Figure 9C).
For typical surfaces of the ORS Facies (i.e., excluding those with multicellular-grade TOS), STC-I and TC-MM3 provide contrasting examples (Figure 8). Dickinsonia is the most common macrofossil on each of these two beds. The base of TC-MM3 is characterized by muted but still readily distinguishable ripples, no adherence to the underlying bed and visible TOS (MMI of 3) with a fossil density of 18.4 individuals/m2. We interpret TC-MM3 to record a relatively mature community. TC-MM3 is also characterized by relatively high taxonomic diversity with 15 described genera (Figure 9 and Table 2). In contrast, bed STC-I is characterized by sharp-crested ripples, no visible TOS and was underlain by a shim that strongly adhered to STC-I, indicating that the mat colonizing that shim (and preserved on the base of STC-I) was immature. This bed has 7 macrofossil genera, a fossil density of 5.8 individuals/m2 and a Shannon diversity index of 1.55, just slightly higher than TC-MM3 (Table 2).
1T-F, also from the ORS Facies with common Dickinsonia, exemplifies a mature mat (MMI 4) (Figure 8). 1T-F does not adhere to the underlying bed and ripples are strongly muted. Furthermore, 1T-F is characterized by spatially heterogenous TOS with local areas of relatively high (mm-scale) relief (Figure 1C). 1T-F records 16 genera and the highest Shannon diversity index recorded at NENP (H = 2.12). Although Dickinsonia is common, 1T-F exhibits higher evenness than either TC-MM3 or STC-I (Table 1).
In contrast, Bed 1T-T has a MMI of 4 and, although it is characterized by a high macrofossil density (40.6 individuals/m2) and a relatively high number of genera, it is dominated by Tribrachidium (Figure 8), resulting in a low Shannon diversity index (H = 0.82).
Bedding planes characterized by multicellular TOS are among the most mature beds (Table 1). These beds reflect densely packed communities of Funisia, Plexus or macroalgae that would have required a relatively longer duration between depositional events to develop, grow and experience macroorganism recruitment and colonization. They have a generic diversity ranging from 3 to 7 taxa and Shannon indices ranging from 0.05 to 1.56, depending on whether death of the mat-forming community was closely followed by burial or instead remained unburied at the sediment-water interface for a sufficiently long duration for a new community to develop, thus recording an ecologically time-averaged surface. Those surfaces that were not buried immediately (i.e., time-averaged surfaces) generally have higher generic diversity and Shannon indices because they represent multiple communities. STC-X exhibits such a situation with a community that lived on top of unburied Funisia, as reflected by its eight genera and Shannon diversity index of 1.56 (Figure 3C).
Body size is positively correlated with changes in nutrient availability in modern ecosystems (e.g., Woodward et al., 2005), and variable oxygen concentrations produce size limitations on aerobic organisms (e.g., Payne et al., 2011). Greater time of exposure, as represented by more mature mats, would have increased the probability of growth of macroorganisms to more extreme sizes (Droser et al., 2017). Thus, we might expect mature mats to exhibit a greater range of body sizes, including both juvenile and adult forms. However, advanced maturity would not limit the ability of settlement by juvenile forms and these nutrient-rich organic substrates may even have been preferred by some taxa. Indeed, Obamus is limited to beds characterized by mature mats (e.g., TB-ARB and LV-Fun), suggesting that larvae preferentially colonized these settings (Dzaugis et al., 2019). Body size does appear to be related to TOS recording densely packed fossil algae communities on 1T-BOF, where particularly small specimens of Dickinsonia and Spriggina occur, suggesting that these dense and diverse algae served as a nursery preferentially colonized by juvenile macrobiota (Xiao et al., 2013; Droser et al., 2019).
Dickinsonia was mobile and fed directly on the organic mat (Ivantsov and Malakhovskaya, 2002; Gehling et al., 2005; Sperling and Vinther, 2010; Evans et al., 2019). It occurs on nearly every bed and is the third most common genus, behind Funisia and the holdfast Aspidella, at NENP (Droser et al., 2019). Dickinsonia is the most abundant fossil on seven beds, dominating (>50% of all fossils) four of them. These beds vary in mat maturity, and Dickinsonia is therefore an ideal candidate to investigate potential trends between body size and mat maturity. This fossil is less common on beds characterized by multicellular TOS, potentially due to the difficulty of navigating these high-relief surfaces (Droser et al., 2019) and so we exclude those beds from this evaluation.
Dickinsonia dominates bed TC-MM3 and is the most abundant fossil on 1T-F, both characterized by mature mats (Table 2). However, it also dominates STC-I, one of the least mature mats from the ORS Facies. Trace fossil evidence suggests that a large Yorgia was also present for some period on bed STC-I, although lack of body fossil preservation suggests it moved on prior to burial. Thus, very low mat maturities did not limit the distribution of large, mobile taxa that fed directly on the organic mat, such as Dickinsonia and Yorgia, but it is unclear for what duration they would have been able to support larger forms, especially of those organisms that activity fed upon matgrounds. The ability of these taxa to move into and out of a community (Evans et al., 2019) may complicate the observed impacts of overall low benthic nutrient availability.
To test for potential relationships, we compare the MMI of beds at NENP with the maximum body size of associated Dickinsonia and preserved footprints. Despite the mobility and resultant transient nature of occupation within an environment, we find that mature mats are more likely to contain large Dickinsonia (Figure 10). This supports the hypothesis that greater time of exposure and/or increased nutrient availability on these surfaces supported a greater range of body sizes, at least among mobile and mat-feeding macroorganisms. Although this includes different species of Dickinsonia that may grow at variable rates, all fed in the same manner.
FIGURE 10. Box and whisker plot demonstrating the relationship between Dickinsonia body size and mat maturity.
Body size data for sessile organisms is more limited, largely because they are less common and typically occupy a smaller range of body sizes than Dickinsonia at NENP. Although Aspidella is more abundant, this form genus could represent the holdfast of multiple different taxa, potentially belonging to distinct morphogroups (Rangeomorpha and Arboreomorpha) (Tarhan et al., 2015). However, the preservation of Aspidella assemblages characterized by right-skewed size distributions on individual fossiliferous bedding planes on which it is the most abundant macrofossil is more parsimoniously interpreted as populations of a single taxon (Tarhan et al., 2015). Nonetheless, preservation of Aspidella is intimately linked to mat development (Tarhan et al., 2015; Surprenant et al., 2020). Similarly, as noted here, Funisia most commonly occurs in dense aggregates, often to the exclusion of other taxa and representing a distinct TOS in and of itself (Droser and Gehling, 2008; Gehling and Droser, 2009). Thus, despite their abundance, Aspidella and Funisia are less ideally suited than Dickinsonia as indicators of macroorganism responses to mat maturity.
Tribrachidium is potentially the best sessile taxon for investigating relationships with mat maturity because it occurs on numerous beds (and, like Aspidella and Funisia, spans multiple facies), dominating two (Hall et al., 2015; Droser et al., 2019). The body size distribution of Tribrachidium, however, does not match trends observed for Dickinsonia. Tribrachidium occurs in clusters of similar-sized specimens, with the smallest and largest cohorts preserved on beds TC-MM3 and WS-TBEW (Hall et al., 2015), respectively, both with an MMI of 3. A third cohort of intermediate size occurs occurs on bed 1T-T, likewise characterized by high MMI. Millimeter-scale Tribrachidium also occur on the newly excavated LV-Fun, one of the surfaces with the highest MMI at NENP. Thus, the size of Tribrachidium cohorts does not appear to be correlated with mat maturity and is more likely a random result of the time of colonization relative to burial.
Though there is little evidence of interactions between Ediacara taxa, there are abundant indications that several Ediacara taxa interacted with the organically bound seafloor. Widespread organic surfaces served as a source of food for a number of organisms, particularly mobile taxa, and others lived in close proximity to the mat, embedded or “stuck” within it. However, most of the Ediacara taxa recorded on fossiliferous bedding planes at NENP do not appear to be preferentially associated with particular types of TOS. The most remarkable aspect of the textured organic surfaces at NENP is the variability of morphotypes and the heterogeneity of these surfaces within and between beds. While many of the TOS occurring on these beds are regular in morphology and readily distinguishable, certain textures—particularly on beds with an MMI of 4—consist of irregular complex TOS. This level of heterogeneity is consistent with that characterizing some modern well-developed mats (Stal et al., 1985; Hawes et al., 2016; Gomes et al., 2020).
Based on biomarker data, cyanobacteria may have been the primary constituents of many Proterozoic photosynthetic mats (Gomes et al., 2020). However, other taxa clearly contributed to these communities (Gomes et al., 2020). This is obvious in the case of multicellular TOS but it also likely true of even “microbial-grade” TOS. In particular, variability within an individual bed, such as LV-Fun (Figure 2) on the scale of centimeters and decimeters likely reflects compositional differences in that environmental differences on such a small scale can be largely discounted. This is consistent with modern mats that are heterogeneous on micro and macro scales, reflecting a variety of environmental and compositional differences.
Time between depositional events was irregular as indicated by the range of mat maturities. As predicted, fossil density and number of genera appear to be positive correlated with MMI. Regions of the seafloor characterized by longer “hiatal” intervals between depositional events experienced greater matground growth and maturity and fostered the colonization of more macroorganisms representing different genera. However, with the exception of Obamus and Coronacollina, the distribution of individual taxa does not appear to have been dictated by mat maturity. The distribution of these two taxa is consistent with larval settlement preferences.
Individual beds of the Ediacara Member exhibit a wide range of evenness values (Table 2; Droser and Gehling, 2015). There are beds with evenness values on par with those of the Phanerozoic, such as 1T-F (Droser and Gehling, 2015; Droser et al., 2019; Finnegan et al., 2019). However, those data currently available suggest that, following Powell and Kowalewski (2002), on the whole, evenness may have increased through time (Droser and Gehling, 2015). The prevalence of lower evenness values in general in the Ediacaran as compared with the Phanerozoic likely impacts the potential correlation of MMI with fossil assemblage evenness. In other words, a high-dominance community is not necessarily “immature”. For example, 1T-T has an MMI of 4 with a relatively high number of genera and high fossil density—but is dominated by a cohort of Tribrachidium (Hall et al., 2015). It would be difficult to interpret this bed as recording anything other than a relatively mature macroorganism community. For most beds at NENP, the same taxa are present across beds but not in equivalent abundances; it is the varying relative abundances of each bed that distinctively characterize these fossil assemblages. High dominance by an individual taxon is not uncommon (Droser et al., 2019). For example, Dickinsonia and the frond holdfast Aspidella each occur on nearly all of the beds at NENP. Dickinsonia dominates some beds and Aspidella-associated frondose organisms presumably dominated others. Thus, although these metrics are directly related, the lack of correlation of Shannon diversity indices and evenness in fossilized Ediacara communities should perhaps not be surprising.
Mats have long been recognized to be significant components of Ediacaran ecosystems. They form an integral part of the actual communities as sources of food, providing a suitable and stabilized substrate and potentially a source of oxygen in a low-oxygen world. While it is not possible to evaluate biomass from these fossil communities, given the widespread nature of these mats and the maturity and complexity of mats with sufficient time to develop, it is very likely that mats constituted a significant component of Ediacaran biomass as they do in certain environments today (e.g., Sutherland, 2009). Interestingly, the type of mat does not appear to be a controlling factor in the distribution of taxa. However, the maturity of mats, reflecting the duration of time between events is not surprisingly correlated with both density and generic diversity.
The raw data supporting the conclusion of this article will be made available by the authors, without undue reservation.
MD and SE wrote the first draft of the paper. SE and IH did the data analysis. Concepts and subsequent drafts were developed by all of the authors.
This work was supported by a NASA Exobiology grant (80NSSC19K0472 to MD, LT, and SE) and NASA Earth and Space Science Fellowships (grant PLANET17F-0124 to SE and 20-PLANET20-0015 to RS).
The authors declare that the research was conducted in the absence of any commercial or financial relationships that could be construed as a potential conflict of interest.
All claims expressed in this article are solely those of the authors and do not necessarily represent those of their affiliated organizations, or those of the publisher, the editors and the reviewers. Any product that may be evaluated in this article, or claim that may be made by its manufacturer, is not guaranteed or endorsed by the publisher.
We are grateful to Ross and Jane Fargher for access to the National Heritage Nilpena Ediacara fossil site on their property, acknowledging that this land lies within the Adnyamathanha Traditional Lands. We are also grateful to the South Australia Department of the Environment and Water for facilitating field work. We thank P. Dzaugis, M. Dzaugis and J. Irving for field assistance. H. McCandless aided in the preparation of this manuscript.
Almela, P., Velázquez, D., Rico, E., Justel, A., and Quesada, A. (2019). Carbon Pathways through the Food Web of a Microbial Mat from Byers Peninsula, Antarctica. Front. Microbiol. 10, 628. doi:10.3389/fmicb.2019.00628
Bose, P. K., Eriksson, P. G., Sarkar, S., Wright, D. T., Samanta, P., Mukhopadhyay, S., et al. (2012). Sedimentation Patterns during the Precambrian: a Unique Record? Mar. Pet. Geology. 33, 34–68. doi:10.1016/j.marpetgeo.2010.11.002
Bottjer, D. J., Hagadorn, J. W., Schieber, J., Bose, P. K., Eriksson, P. G., Banjeree, S., et al. (2007). “Mat-growth Features,” in Atlas of Microbial Mat Features Preserved within the Clastic Rock Record (Amsterdam, New York: Elsevier), 53–71.
Bouougri, E. H., and Porada, H. (2007). Siliciclastic Biolaminites Indicative of Widespread Microbial Mats in the Neoproterozoic Nama Group of Namibia. J. Afr. Earth Sci. 48, 38–48. doi:10.1016/j.jafrearsci.2007.03.004
Carbone, C., and Narbonne, G. M. (2014). When Life Got Smart: The Evolution of Behavioral Complexity through the Ediacaran and Early Cambrian of NW Canada. J. Paleontol. 88, 309–330. doi:10.1666/13-066
Clites, E. C., Droser, M. L., and Gehling, J. G. (2012). The Advent of Hard-Part Structural Support Among the Ediacara Biota: Ediacaran harbinger of a Cambrian Mode of Body Construction. Geology 40, 307–310. doi:10.1130/g32828.1
Cole, D. B., Mills, D. B., Erwin, D. H., Sperling, E. A., Porter, S. M., Reinhard, C. T., et al. (2020). On the Co-evolution of Surface Oxygen Levels and Animals. Geobiology 18, 260–281. doi:10.1111/gbi.12382
Corenbilt, D., Darrozes, J., Julien, F., Otto, T., Roussel, E., Steiger, J., et al. (2019). The Search for a Signature of Life on Mars: A Biogeomorphological Approach. Astrobiol. 19, 1279–1291. doi:10.1089/ast.2018.1969
Coutts, F. J., Bradshaw, C. J. A., García-Bellido, D. C., and Gehling, J. G. (2018). Evidence of Sensory-Driven Behavior in the Ediacaran Organism Parvancorina: Implications and Autecological Interpretations. Gondwana Res. 55, 21–29. doi:10.1016/j.gr.2017.10.009
Darroch, S. A. F., Sperling, E. A., Boag, T. H., Racicot, R. A., Mason, S. J., Morgan, A. S., et al. (2015). Biotic Replacement and Mass Extinction of the Ediacara Biota. Proc. R. Soc. B. 282, 20151003. doi:10.1098/rspb.2015.1003
Doemel, W. N., and Brock, T. D. (1977). Structure, Growth, and Decomposition of Laminated Algal-Bacterial Mats in Alkaline hot springs. Appl. Environ. Microbiol. 34 (4), 433–452. doi:10.1128/aem.34.4.433-452.1977
Droser, M. L., Gehling, J. G., and Jensen, S. R. (2006). Assemblage Palaeoecology of the Ediacara Biota: The Unabridged Edition? Palaeogeogr. Palaeoclimatol. Palaeoecol. 232, 131–147. doi:10.1016/j.palaeo.2005.12.015
Droser, M. L., and Gehling, J. G. (2008). Synchronous Aggregate Growth in an Abundant New Ediacaran Tubular Organism. Science 319, 1660–1662. doi:10.1126/science.1152595
Droser, M. L., Gehling, J. G., Tarhan, L. G., Evans, S. D., Hall, C. M. S., Hughes, I. V., et al. (2019). Piecing Together the Puzzle of the Ediacara Biota: Excavation and Reconstruction at the Ediacara National Heritage Site Nilpena (South Australia). Palaeogeogr. Palaeoclimatol. Palaeoecol. 513, 132–145. doi:10.1016/j.palaeo.2017.09.007
Droser, M. L., and Gehling, J. G. (2015). The Advent of Animals: The View from the Ediacaran. Proc. Natl. Acad. Sci. USA 112, 4865–4870. doi:10.1073/pnas.1403669112
Droser, M. L., Tarhan, L. G., and Gehling, J. G. (2017). The Rise of Animals in a Changing Environment: Global Ecological Innovation in the Late Ediacaran. Annu. Rev. Earth Planet. Sci. 45, 593–617. doi:10.1146/annurev-earth-063016-015645
Dzaugis, P. W., Evans, S. D., Droser, M. L., Gehling, J. G., and Hughes, I. V. (2019). Stuck in the Mat: Obamus Coronatus, a New Benthic Organism from the Ediacara Member, Rawnsley Quartzite, South Australia. Aust. J. Earth Sci. 67, 1440–0952. doi:10.1080/08120099.2018.1479306
Elliott, D. A., Vickers-Rich, P., Trusler, P., and Hall, M. (2011). New Evidence on the Taphonomic Context of the EdiacaranPteridinium. Acta Palaeontologica Pol. 56, 641–650. doi:10.4202/app.2010.0060
Eriksson, P. G., Schieber, J., Bouougri, E., Gerdes, G., Porada, H., Banerjee, S., et al. (2007). CHAPTER THREE CLASSIFICATION OF STRUCTURES LEFT BY MICROBIAL MATS IN THEIR HOST SEDIMENTS.
Evans, S. D., Diamond, C. W., Droser, M. L., and Lyons, T. W. (2018a). Dynamic Oxygen and Coupled Biological and Ecological Innovation during the Second Wave of the Ediacara Biota. Emerging Top. Life Sci. 2, 223–233. doi:10.1042/etls20170148
Evans, S. D., Dzaugis, P. W., Droser, M. L., and Gehling, J. G. (2018b). You Can Get Anything You Want from Alice’s Restaurant Bed: Exceptional Preservation and an Unusual Fossil Assemblage from a Newly Excavated Bed (Ediacara Member, Nilpena, South Australia). Aust. J. Earth Sci. 67, 1440–0952. doi:10.1080/08120099.2018.1470110
Evans, S. D., Gehling, J. G., and Droser, M. L. (2019). Slime Travelers: Early Evidence of Animal Mobility and Feeding in an Organic Mat World. Geobiology 17, 490–509. doi:10.1111/gbi.12351
Evans, S. D., Hughes, I. V., Gehling, J. G., and Droser, M. L. (2020). Discovery of the Oldest Bilaterian from the Ediacaran of South Australia. Proc. Natl. Acad. Sci. USA 117, 7845–7850. doi:10.1073/pnas.2001045117
Fedonkin, M. A. (1985). “Systematic Description of Vencian Metazoans,” in The Vendian System. Paleontology. 1. Editors B. S. Sokolov, and A. B. Iwanowski (Berlin: Springer-Verlag), 71–120.
Finnegan, S., Gehling, J. G., and Droser, M. L. (2019). Unusually Variable Paleocommunity Composition in the Oldest Metazoan Fossil Assemblages. Paleobiology. 45 (2), 235–245. doi:10.1017/pab.2019.1
Gehling, J. G., and Droser, M. L. (2009). Textured Organic Surfaces Associated with the Ediacara Biota in South Australia. Earth Sci. Revs. 96, 196–206. doi:10.1016/j.earscirev.2009.03.002
Gehling, J. G., and Droser, M. L. (2013). How Well Do Fossil Assemblages of the Ediacara Biota Tell Time. Geology 41, 447–450. doi:10.1130/g33881.1
Gehling, J. G., and Droser, M. L. (2018). Ediacaran Scavenging as a Prelude to Predation. Emerg. Top. Life Sci. 2, 213–222. doi:10.1042/etls20170166
Gehling, J. G. (1999). Microbial Mats in Terminal Proterozoic Siliciclastics: Ediacaran Death Masks. Palaios 14, 40–57. doi:10.2307/3515360
Gehling, J. G. (2000). Environmental Interpretation and a Sequence Stratigraphic Framework for the Terminal Proterozoic Ediacara Member within the Rawnsley Quartzite, South Australia. Precambrian Res. 100, 65–95. doi:10.1016/s0301-9268(99)00069-8
Gehling, J. G., Droser, M. L., Jensen, S., and Runnegar, B. N. (2005). “Ediacara Organisms: Relating Form to Function,” in Evolving Form and Function: Fossils and Development. Editor D. E. G. Briggs (New Haven, CT.: Yale Univ. Press), 43–66.
Gehling, J. G., Runnegar, B. N., and Droser, M. L. (2014). Scratch Traces of Large Ediacara Bilaterian Animals. J. Paleontol. 88, 284–298. doi:10.1666/13-054
Gehling, J. G., García-Bellido, D. C., Droser, M. L., Tarhan, L. G., and Runnegar, B. (2019). The Ediacaran-Cambrian Transition: Sedimentary Facies versus Extinction. Estud. Geol. 75, e099. doi:10.3989/egeol.43601.554
Gingras, M., Hagadorn, J. W., Seilacher, A., Lalonde, S. V., Pecoits, E., Petrash, D., et al. (2011). Possible Evolution of mobile Animals in Association with Microbial Mats. Nat. Geosci 4, 372–375. doi:10.1038/ngeo1142
Gomes, M. L., Riedman, L. A., O’Reilly, S., Lingappa, U., Metcalfe, K., Fike, D. A., et al. (2020). Taphonomy of Biosignatures in Microbial Mats on Little Ambergris Cay, Turks and Caicos Islands. Front. Earth Sci. 8, 576712. doi:10.3389/feart.2020.576712
Hagadorn, J. W., and Bottjer, D. J. (1999). Restriction of a Late Neoproterozoic Biotope: Suspect-Microbial Structures and Trace Fossils at the Vendian-Cambrian Transition. Palaios 14, 73–85. doi:10.2307/3515362
Hall, C. M. S., Droser, M. L., Gehling, J. G., and Dzaugis, M. E. (2015). Paleoecology of the Enigmatic Tribrachidium: New Data from the Ediacaran of South Australia. Precambrian Res. 269, 183–194. doi:10.1016/j.precamres.2015.08.009
Hawes, I., Jungblut, A. D., Obryk, M. K., and Doran, P. T. (2016). Growth Dynamics of a Laminated Microbial Mat in Response to Variable Irradiance in an Antarctic lake. Freshw. Biol. 61 (4), 396–410. doi:10.1111/fwb.12715
Hill, C., Corcoran, P. L., Aranha, R., and Longstaffe, F. J. (2016). Microbially Induced Sedimentary Structures in the Paleoproterozoic, Upper Huronian Supergroup, Canada. Precambrian Res. 281, 155–165. doi:10.1016/j.precamres.2016.05.010
Ivantsov, A. Y. (2009). New Reconstruction of Kimberella, Problematic Vendian Metazoan. Paleontolog. J. 43, 3–12. doi:10.1134/s003103010906001x
Ivantsov, A. Y. (2011). Feeding Traces of Proarticulata-The Vendian Metazoa. Paleontol. J. 45, 237–248. doi:10.1134/s0031030111030063
Ivantsov, A. Y., and Malakhovskaya, Y. E. (2002). Giant Traces of Vendian Animals. In Doklady Earth Sciences C/C of Doklady-Akademiia Nauk. INTERPERIODICA PUBLISHING. 385, 618–622.
Joel, L. V., Droser, M. L., and Gehling, J. G. (2014). A New Enigmatic, Tubular Organism from the Ediacara Member, Rawnsley Quartzite, South Australia. J. Paleontol. 88 (2), 253–262. doi:10.1666/13-058
Kovalchuck, O., Owttrim, G. W., Konhauser, K. O., and Gingras, M. K. (2017). Desiccation Cracks in Siliciclastic Deposits: Microbial Mat-Related Compared to Abiotic Sedimentary Origin. Sediment. Geol. 347, 67–68. doi:10.1016/j.sedgeo.2016.11.002
Kumar, S., and Ahmad, S. (2014). Microbially Induced Sedimentary Structures (MISS) from the Ediacaran Jodhpur Sandstone, Marwar Supergroup, Western Rajasthan. J. Asian Earth Sci. 91, 352–361. doi:10.1016/j.jseaes.2014.01.009
Laflamme, M., Schiffbauer, J. D., and Narbonne, G. M. (2012). “Deep-water Microbially Induced Sedimentary Structures (MISS) in Deep Time: The Ediacaran Fossil Ivesheadia,”. Microbial Mats in Siliciclastic Depositional Systems through Time. Editors N. Noffke, and H. Chafetz (Tulsa: SEPM Special Publication), 101, 111–123.
Liu, A. G., Brasier, M. D., Bogolepova, O. K., Raevskaya, E. G., and Gubanov, A. P. (2013). First Report of a Newly Discovered Ediacaran Biota from the Irkineeva Uplift, East Siberia. Newsletters Stratigr. 46, 95–110. doi:10.1127/0078-0421/2013/0031
Mariotti, G., Pruss, S. B., Perron, J. T., and Bosak, T. (2014). Microbial Shaping of Sedimentary Wrinkle Structures. Nat. Geosci 7, 736–740. doi:10.1038/ngeo2229
Morganti, T. M., Purser, A., Rapp, H. T., German, C. R., Jakuba, M. V., Hehemann, L., et al. (2021). In Situ observation of Sponge Trails Suggests Common Sponge Locomotion in the Deep central Arctic. Curr. Biol. 31 (8), R368–R370. doi:10.1016/j.cub.2021.03.014
Nettle, D., Halverson, G. P., Cox, G. M., Collins, A. S., Schmitz, M., Gehling, J., et al. (2014). A Middle-Late Ediacaran Volcano-Sedimentary Record from the Eastern Arabian-Nubian Shield. Terra Nova 26, 120–129. doi:10.1111/ter.12077
Noffke, N. (2009). The Criteria for the Biogeneicity of Microbially Induced Sedimentary Structures (MISS) in Archean and Younger, sandy Deposits. Earth-Science Rev. 96, 173–180. doi:10.1016/j.earscirev.2008.08.002
Noffke, N. (2015). Ancient Sedimentary Structures in the <3.7 Ga Gillespie Lake Member, Mars, that Resemble Macroscopic Morphology, Spatial Associations, and Temporal Succession in Terrestrial Microbialites. Astrobiology 15, 169–192. doi:10.1089/ast.2014.1218
Noffke, N., Knoll, A., and Grotzinger, J. (2002). Sedimentary Controls on the Formation and Preservation of Microbial Mats in Siliciclastic Deposits: A Case Study from the Upper Neoproterozoic Nama Group, Namibia. Palaios. 17, 533–544. doi:10.1669/0883-1351(2002)017<0533:scotfa>2.0.co;2
Paterson, J. R., Gehling, J. G., Droser, M. L., and Bicknell, R. D. C. (2017). Rheotaxis in the Ediacaran Epibenthic Organism Parvancorina from South Australia. Sci. Rep. 7, 45539. doi:10.1038/srep45539
Payne, J. L., McClain, C. R., Boyer, A. G., Brown, J. H., Finnegan, S., Kowalewski, M., et al. (2011). The Evolutionary Consequences of Oxygenic Photosynthesis: A Body Size Perspective. Photosynth. Res. 107, 37–57. doi:10.1007/s11120-010-9593-1
Porada, H., and Bouougri, E. H. (2007). Wrinkle Structures-A Critical Review. Earth-Science Rev. 81, 199–215. doi:10.1016/j.earscirev.2006.12.001
Powell, M. G., and Kowalewski, M. (2002). Increase in Evenness and Sampled Alpha Diversity through the Phanerozoic: Comparison of Early Paleozoic and Cenozoic marine Fossil Assemblages. Geol 30 (4), 331–334. doi:10.1130/0091-7613(2002)030<0331:iieasa>2.0.co;2
Reinhard, C. T., and Planavsky, N. J. (2021). The History of Ocean Oxygenation. Annu. Rev. Mar. Sci. 14, 331–353. doi:10.1146/annurev-marine-031721-104005
Reinhard, C. T., Planavsky, N. J., Olson, S. L., Lyons, T. W., and Erwin, D. H. (2016). Earth's Oxygen Cycle and the Evolution of Animal Life. Proc. Natl. Acad. Sci. USA 113, 8933–8938. doi:10.1073/pnas.1521544113
Sarkar, S., Bose, P., Samanta, P., Sengupta, P., and Eriksson, P. (2008). Microbial Mat Mediated Structures in the Ediacaran Sonia Sandstone, Rajasthan, India, and Their Implications for Proterozoic Sedimentation. Precambrian Res. 162, 248–263. doi:10.1016/j.precamres.2007.07.019
Sarkar, S., Samanta, P., and Altermann, W. (2011). Setulfs, Modern and Ancient: Formative Mechanism, Preservation Bias and Palaeoenvironmental Implications. Sediment. Geology. 238, 71–78. doi:10.1016/j.sedgeo.2011.04.003
Sarkar, S., Choudhuri, A., Mandal, S., and Eriksson, P. G. (2016). Microbial Mat-Related Structures Shared by Both Siliciclastic and Carbonate Formations. J. Palaeogeogr. 5, 278–291. doi:10.1016/j.jop.2016.05.001
Seilacher, A. (1999). Biomat-Related Lifestyles in the Precambrian. PALAIOS 14 (1), 86–93. doi:10.2307/3515363
Sperling, E. A., and Vinther, J. (2010). A Placozoan Affinity for Dickinsonia and the Evolution of Late Proterozoic Metazoan Feeding Modes. Evol. Dev. 12, 201–209. doi:10.1111/j.1525-142x.2010.00404.x
Sperling, E. A., Wolock, C. J., Morgan, A. S., Gill, B. C., Kunzmann, M., Halverson, G. P., et al. (2015). Statistical Analysis of Iron Geochemical Data Suggests Limited Late Proterozoic Oxygenation. Nature 523, 451–454. doi:10.1038/nature14589
Stal, L. J., Gemerden, H., and Krumbein, W. E. (1985). Structure and Development of a Benthic marine Microbial Mat. FEMS Microbiol. Ecol. 31 (2), 111–125. doi:10.1111/j.1574-6968.1985.tb01138.x
Surprenant, R. L., Gehling, J. G., and Droser, M. L. (2020). Biological and Ecological Insights from the Preservational Variability of Funisia Dorothea, Ediacara Member, South Australia. PALAIOS 35, 359–376. doi:10.2110/palo.2020.014
Sutherland, D. L. (2009). Microbial Mat Communities in Response to Recent Changes in the Physiochemical Environment of the Meltwater Ponds on the McMurdo Ice Shelf, Antarctica. Polar Biol. 32, 1023–1032. doi:10.1007/s00300-009-0601-x
Tarhan, L. G., Droser, M. L., and Gehling, J. G. (2010). Taphonomic Controls on Ediacaran Diversity: Uncovering the Holdfast Origin of Morphologically Variable Enigmatic Structures. Palaios. 25, 823–830. doi:10.2110/palo.2010.p10-074r
Tarhan, L. G., Droser, M. L., Gehling, J. G., and Dzaugis, M. P. (2015). Taphonomy and Morphology of the Ediacara Form Genus Aspidella. Precambrian Res. 257, 124–136. doi:10.1016/j.precamres.2014.11.026
Tarhan, L. G., Hood, A. V. S., Droser, M. L., Gehling, J. G., and Briggs, D. E. G. (2016). Exceptional Preservation of Soft-Bodied Ediacara Biota Promoted by Silica-Rich Oceans. Geology 44, 951–954. doi:10.1130/g38542.1
Tarhan, L. G., Droser, M. L., Gehling, J. G., and Dzaugis, M. P. (2017). Microbial Mat Sandwiches and Other Anactualistic Sedimentary Features of the Ediacara Member (Rawnsley Quartzite, South Australia): Implications for Interpretation of the Ediacaran Sedimentary Record. Palaios 32, 181–194. doi:10.2110/palo.2016.060
Tarhan, L. G., Droser, M. L., and Gehling, J. G. (2022). Picking Out the Warp and Weft of the Ediacaran Seafloor: Paleoenvironment and Paleoecology of an Ediacara Textured Organic Surface. Precambrian Res. 369, 106539. doi:10.1016/j.precamres.2021.106539
Vago, J. L., and Westall, F.Pasteur Instrument Teams, Landing Site Selection Working Group, andOther Contributors (2017). Habitability on Early Mars and the Search for Biosignatures with the ExoMars Rover. Astrobiol. 17, 471509. doi:10.1089/ast.2016.1533
Woodward, G., Ebenman, B., Emmerson, M., Montoya, J., Olesen, J., Valido, A., et al. (2005). Body Size in Ecological Networks. Trends Ecol. Evol. 20, 402–409. doi:10.1016/j.tree.2005.04.005
Xiao, S., Droser, M., Gehling, J. G., Hughes, I. V., Wan, B., Chen, Z., et al. (2013). Affirming Life Aquatic for the Ediacara Biota in China and Australia. Geology 41 (10), 1095–1098. doi:10.1130/G34691.1
Xiao, S., Chen, Z., Zhou, C., and Yuan, X. (2019). Surfing in and on Microbial Mats: Oxygen-Related Behavior of a Terminal Ediacaran Bilaterian Animal. Geology 47 (11), 1054–1058. doi:10.1130/g46474.1
Xiao, S., Chen, Z., Pang, K., Zhou, C., and Yuan, X. (2020). The Shibantan Lagerstätte: Insights into the Proterozoic–Phanerozoic Transition. Jol. Geol. Soc. 178, jgs2020 135. doi:10.1144/jgs2020-135
Keywords: Ediacara, organic mat, paleoecology, Ediacaran, Ediacara Biota
Citation: Droser ML, Evans SD, Tarhan LG, Surprenant RL, Hughes IV, Hughes EB and Gehling JG (2022) What Happens Between Depositional Events, Stays Between Depositional Events: The Significance of Organic Mat Surfaces in the Capture of Ediacara Communities and the Sedimentary Rocks That Preserve Them. Front. Earth Sci. 10:826353. doi: 10.3389/feart.2022.826353
Received: 30 November 2021; Accepted: 31 January 2022;
Published: 23 February 2022.
Edited by:
Dermeval Aparecido Do Carmo, University of Brasilia, BrazilReviewed by:
Mao Luo, Nanjing Institute of Geology and Paleontology (CAS), ChinaCopyright © 2022 Droser, Evans, Tarhan, Surprenant, Hughes, Hughes and Gehling. This is an open-access article distributed under the terms of the Creative Commons Attribution License (CC BY). The use, distribution or reproduction in other forums is permitted, provided the original author(s) and the copyright owner(s) are credited and that the original publication in this journal is cited, in accordance with accepted academic practice. No use, distribution or reproduction is permitted which does not comply with these terms.
*Correspondence: Mary L. Droser, TWFyeS5kcm9zZXJAdWNyLmVkdQ==
Disclaimer: All claims expressed in this article are solely those of the authors and do not necessarily represent those of their affiliated organizations, or those of the publisher, the editors and the reviewers. Any product that may be evaluated in this article or claim that may be made by its manufacturer is not guaranteed or endorsed by the publisher.
Research integrity at Frontiers
Learn more about the work of our research integrity team to safeguard the quality of each article we publish.