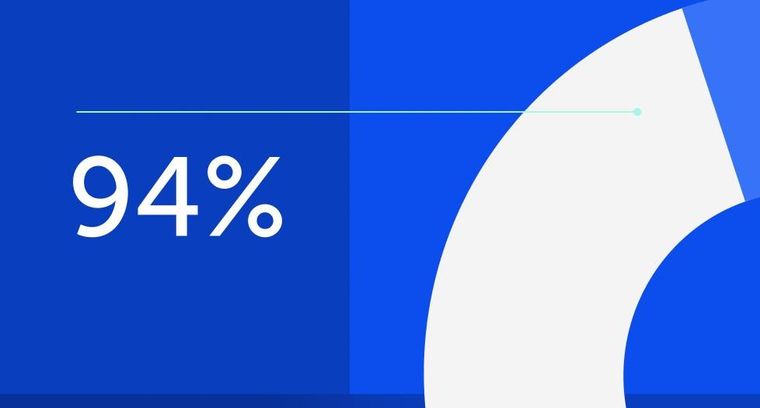
94% of researchers rate our articles as excellent or good
Learn more about the work of our research integrity team to safeguard the quality of each article we publish.
Find out more
ORIGINAL RESEARCH article
Front. Earth Sci., 26 July 2022
Sec. Geochemistry
Volume 10 - 2022 | https://doi.org/10.3389/feart.2022.810597
This article is part of the Research TopicFluid-Mobile Element Tracers of Subduction Processes – The Record in Volcanic Arc Magmas and Exposed Subduction ComplexesView all 7 articles
Continental arcs have an episodic magmatic activity over long-time periods, which is believed to modulate long-term climate. Island arcs have also the potential to release large amount of CO2 into the atmosphere, but whether they display an episodic magmatic history throughout their lifespan that contributes to the long-term (>10 Ma) climate changes remains an open question. To set additional constraints on the magmatic history of island arcs, here we examine fresh basalts and mineral-hosted melt inclusions from the Izu intra-oceanic arc, shortly after the eruption of boninites (∼45 Ma ago). Using chemical markers, we show that the long-term magmatic activity of the mature Izu arc has been relatively continuous over its lifespan, except during opening of the Shikoku back-arc Basin (∼23–20 Ma). Because slab dehydration and slab melting trigger decarbonation and carbonate dissolution of the subducted plate, we use slab-fluid markers (Ba/Th, Cs/Th, Cs/Ba, Rb/Th, Th/Nb) to examine the variations of slab-derived CO2 captured by the arc magmas. The long-term steadiness in the arc magmatic activity and in the slab-fluid contribution suggests that the CO2 outgassed during mature arc volcanism may have remained relatively homogeneous for the past 40 Ma in Izu. If worldwide mature island arcs also maintain a relatively steady-state magmatic activity over their lifespan, the long-term CO2 outgassed by these arc volcanoes may be rapidly balanced by chemical weathering and tectonic erosion, which rapidly draw down the atmospheric CO2 (within 200–300 kyr). This rapid negative feedback to long-term volcanic degassing permits to sustain a viable atmospheric CO2 for millions of years. The lack of co-variations between the markers of climate changes (δ13O, δ18C) and the long-term averages of the markers of slab fluids further implies that long-term volcanic degassing of CO2 from mature island arcs might play a minor role in the slide into icehouse climatic conditions. This long-term degassing stability may be, instead, a contributor to maintaining a broadly stable climate over long timescales.
The exchanges in volatile species (H2O, CO2, S, Cl) between the Earth’s surface and its interior have played a central role in sustaining planet habitability for hundreds of millions of years. Subduction zones have efficiently cycled volatiles between the ocean–atmosphere and the mantle over geological times (Kerrick, 2001; Berner, 2005) by replenishing the volatile supply into the interior of the Earth. The release of subducted seawater back into the asthenospheric mantle triggers arc volcanism (Grove et al., 2006), which has contributed to maintain liveable conditions on Earth. However, it is still unclear whether the emission of volcanic CO2 by island arcs has the potential to affect long-term climate changes (>10 Ma) (Sleep and Zahnle, 2001; Dasgupta and Hirschmann, 2010; Eguchi et al., 2020). Island arcs have been proposed to play a minor role on the long-term climate fluctuations, as continental arcs intersecting carbonate platforms may dominate the volcanic CO2 released into the atmosphere during flare-ups (i.e., periods of high magma addition) (Berner, 1999; Lee et al., 2013; McKenzie et al., 2016; Cao et al., 2017; Mason et al., 2017). Despite their potential to release large amounts of volcanic CO2 in the atmosphere (Marty and Tolstikhin, 1998; Hilton et al., 2002; Dasgupta and Hirschmann, 2010; Johnston et al., 2011; Kelemen and Manning, 2015), lack of magmatic records throughout their lifespan hinders our current understanding of the potential role of island arcs on past climate variations. Such knowledge gaps have raised questions such as: to what extent volcanic degassing in island arcs might have caused past climate fluctuations? Does it control the long-term (>10 Ma, such as greenhouse-icehouse climates or climate optimum) or the short-term (≤ 1 Ma) climate variations (e.g., Milankovitch cycles)?
The Izu intra-oceanic arc is a long-lived subduction zone, which initiated about 52 Ma ago (Stern and Bloomer, 1992; Ishizuka et al., 2006; Reagan et al., 2010; Ishizuka et al., 2011a; Shervais et al., 2019). Drilling expeditions (e.g., IODP Expeditions 350 and 352, and ODP Sites 782 and 786) have provided unique, high-resolution records of the magmatic sequences that erupted throughout the lifespan of the Izu arc (Straub and Layne, 2003; Straub et al., 2004; Busby et al., 2017; Reagan et al., 2017; Reagan et al., 2019; Shervais et al., 2021). The Izu intra-oceanic arc thus represents an ideal location to examine the temporal evolution of island arc magmatism and slab-derived fluids since arc inception (Ishizuka et al., 2011b; Reagan et al., 2019; Shervais et al., 2021), and their influence on past climate change. To set additional constraints onto the processes that control arc magmatic activity, we examine the composition of the Izu arc magmas that erupted shortly after the termination of boninitic magmatism (∼45 Ma ago) (Ishizuka et al., 2011a; Ishizuka et al., 2011b; Reagan et al., 2017; Reagan et al., 2019; Ishizuka et al., 2020). Building on previous work (Gill et al., 1994; Bryant et al., 2003; Straub, 2003; Straub et al., 2010; Straub et al., 2015; Aiuppa et al., 2017), we use chemical markers to examine the temporal variations in i) the extent of mantle melting, ii) the length of the melting column (and hence, in the arc crustal thickness), iii) mantle flow and mantle source compositions, and iv) in water-rich and solute-rich slab fluids released from the subducted Pacific plate spanning over the life of the Izu arc. Correlations between the slab-fluid markers and the CO2/ST ratios outgassed by worldwide island arcs (Aiuppa et al., 2017) demonstrate that slab dehydration and slab melting drive slab decarbonation (Ague and Nicolescu, 2014; Vitale Brovarone et al., 2018). Therefore, the temporal variations in markers of water-rich slab fluids (Ba/Th, Cs/Th, Cs/Ba, Rb/Th) and sediment-derived melts (Th/Nb) are further utilized to track the variations in slab-derived CO2 released during volcanism over the lifetime of the Izu arc. Finally, we discuss the implications of our results to explore whether volcanic degassing by mature island arcs has played a central role on long-term climate changes for the past 45 Ma.
The Izu convergent margin represents the northern part of the Izu-Bonin-Mariana (IBM) intra-oceanic arc system (Figure 1) (Ishizuka et al., 2006; Reagan et al., 2010; Ishizuka et al., 2011a; Reagan et al., 2019). The Pacific plate began to subduct underneath the Philippine Sea plate about 52 Ma ago. To the north, the Izu arc is colliding with the Japan arc, forming the Izu peninsula (Saito and Tani, 2017). Lack of sedimentary cover in the IBM fore-arc exposes the oceanic crust that formed during the early stages of the subduction zone to the surface (Stern et al., 2003).
FIGURE 1. Locality maps. (A) Location of the Izu-Bonin-Mariana intra-oceanic arc system in the Western Pacific. The red box shows the area of (B). (B) Location map of the northern Izu convergent margin. A 100-km wide extensional zone that extends westward from the active rifts, as represented by the blue dashed lines. The location of the IODP and ODP drilling sites used in this study are also plotted. Figure is modified after http://publications.iodp.org/scientific_prospectus/350/ and Straub (2003).
The IBM early arc (also named infant arc hereafter) emplaced shortly after the termination of boninitic volcanism ∼45 Ma ago (Ishizuka et al., 2011a). It was composed of small and scattered submarine volcanoes within 90–100 km from the trench (Ishizuka et al., 2011a; Ishizuka et al., 2020; Kanayama et al., 2012, 2014). Infant arc volcanoes progressively evolved into a mature, thicker-crust volcanic arc that is currently located at ∼ 150–200 km from the trench. The mature arc volcanoes now overlie a deeper portion of the subducted Pacific slab (∼100 km depth). The release of slab fluids from the deeper part of the subducted plate has progressively conferred an arc fingerprint to the arc magmas (Hochstaedter et al., 2001; Straub, 2003; Straub and Layne, 2003; Tollstrup et al., 2010; Ribeiro et al., 2019). The arc magmatic activity has become more focused and has rapidly stabilized in its current location around 41 Ma (Ishizuka et al., 2011a; Kanayama et al., 2012, 2014; Ribeiro et al., 2019). The mature volcanic arc front has thus started forming within 10 Ma of subduction inception (Ishizuka et al., 2006; Straub et al., 2010; Ishizuka et al., 2011b; Straub et al., 2015; Ribeiro et al., 2019; Ishizuka et al., 2020).
In Izu, opening of the Shikoku back-arc Basin at ∼ 29–26 Ma disrupted the mature volcanic arc front, forming the Palau-Kyushu Ridge (Okino et al., 1994; Ishizuka et al., 2011b). Mature arc volcanism vanished from ∼23 to 20 Ma to accommodate opening of the Shikoku Basin (Taylor, 1992; Ishizuka et al., 2011b). The mature volcanic arc is now emplaced onto a ∼20-km thick crust (Van der Hilst and Seno, 1993; Stern et al., 2003; Takahashi et al., 2009). Recent back-arc extension (that initiated ∼2.8 Ma ago) has produced an active back-arc rift and back-arc knolls with a bimodal composition behind the Izu arc (Ishizuka et al., 2003; Tollstrup et al., 2010) (Figure 1). Opening of the Shikoku Basin and subduction-collision of the Izu-Bonin Trench with the Ogasawara Plateau triggered a rolling back of the subducted Pacific slab in Quaternary time (Van der Hilst and Seno, 1993; Wallace et al., 2005; Miller et al., 2006). The slab dip of the subducted Pacific plate thus increases southward underneath the Izu volcanic arc (Van der Hilst and Seno, 1993).
We compiled a dataset from the literature (n = 1,448) which integrates the fresh volcanic glass shards (Figure 2E) and associated melt inclusions hosted in plagioclase and clinopyroxene recently drilled in the Izu intra-oceanic arc during the IODP Expeditions 350 (Schindlbeck et al., 2018a; Schindlbeck et al., 2018b) and 352 (Kutterolf et al., 2018), as well as from the ODP Leg 126, Site 782, and ODP Leg 125, Sites 786 and 784 (Bryant et al., 2003; Straub, 2003; Straub and Layne, 2003; Straub et al., 2004; Barnes and Straub, 2010; Straub et al., 2010) (Figure 1B). Samples classified as “Izu volcanic arc front” from the IODP expeditions 350 and 352 were carefully selected. We also used the bulk rock composition of the infant arc basalts from the Bonin Islands (Ishizuka et al., 2020), as well as the bulk composition of volcaniclastics from the ODP Leg 126, Sites 792, 787, 788, 790, 791, 793 (Hiscott and Gill, 1992; Gill et al., 1994). The recent compilation of bulk analyses from the Izu arc (DeBari et al., 2020) is also included.
FIGURE 2. Classification diagrams for sample provenance. (A) K2O, (B) La/Yb and (C) La/Sm vs. SiO2 diagrams used to discriminate the volcaniclastics from the arc front, the back-arc basin and the Izu rear arc (Heywood et al., 2020). (D) Ba/Th vs. Nb/Yb diagram of Pearce et al. (2005) used to track the mantle and the subduction components. (E) Photomicrograph of a basaltic glass shard polished into epoxy for in situ analyses. The microphotograph was taken under a polarized microscope. WR: whole rock. Large white circles with a red outline depict the dataset of Gill et al. (1994) and Hiscott and Gill (1992); while the smaller white circles with a red outline represent the dataset of Debari et al. (2020).
Samples were screened for in situ analyses of fresh glass shards and mineral-hosted melt inclusions where available, as elements such as Rb, Cs and Ba are sensitive to alteration. Glass shards and mineral-hosted melt inclusions were carefully scrutinized for freshness by plotting their water content and trace element ratios against indices of fractionation (see Supplementary Material). Bulk rocks with a total sum of oxides equal to 100 ± 2 wt% and LOI <2 wt% were also filtered to ensure freshness. Because fractionation of some mineral phases occurring in felsic magmas (e.g., biotite, ilmenite, apatite, amphibole) can obscure their primary composition, our sample set was further filtered for basaltic composition (SiO2 ≤ 56 wt%). Our careful screening thus ensures that incompatible element ratios can reliably track mantle and subduction processes (Pearce et al., 2005).
Our filtered dataset includes 1,217 fresh basaltic glass shards and mineral-hosted melt inclusions from tephra layers with an age ranging from 0.04 to 45.12 Ma, 89 bulk rocks from the infant Izu arc with ages ranging from 40.2 to 45.3 Ma, and 339 bulk rocks and bulk sediments from the mature arc with ages ranging from 0 to 30.9 Ma. Our new comprehensive dataset captured the full spectrum of basaltic magmas throughout the lifespan of the Izu arc. The complete dataset can be found in Supplementary Table S1. The compiled dataset of DeBari et al. (2020) is reported at: https://doi.org/10.1594/IEDA/111493.
In this study, we use chemical proxies to track the temporal variations in mantle melting (TiO2, Na6), mantle depletion (TiO2/Y, Nb/Yb) and slab fluids (Ba/Th, Cs/Th, Cs/Ba, Rb/Th, Th/Nb) over the lifetime of the Izu arc to examine the role of island arc magmatism on past climate changes (details are provided below). There are, however, some limitations to our approach. Despite our careful screening, alteration processes may affect magma composition. For instance, the unusually high Ba/Th and H2O/K2O, Rb/Th and Cs/Ba ratios observed in some magmas may reveal alteration, as there is no clear systematics between the different slab-fluid markers (Figures 6–9). Additionally, our filtering towards basaltic composition may also introduce some sample bias, as some short-term variations may only be recorded by the felsic magmas (Gill et al., 1994; Bryant et al., 2003; Straub, 2003; Straub et al., 2015). However, our careful screening aims at minimizing secondary processes (e.g., alteration, crystal fractionation, and fractional melting) to ensure that the markers reliably track primary processes (mantle source and slab fluids). For these reasons, we mainly focus on the long-term temporal variations, as some of the short-term variabilities might have been filtered out. Therefore, this approach allows us to solely examine the long-term variations in the magmatic activity of the arc (≥5 Ma) and of the climate (≥10 Ma). We cannot investigate the role of volcanic degassing on shorter timescales and its influence on short-term climate variations (<1 Ma), as well as the small magmatic cycles (≤5 Ma) that could exist in the Izu arc (Schindlbeck et al., 2018a).
Volcaniclastics can travel from tens to hundreds of kilometers away from their sources. Therefore, their provenance must be carefully examined to ensure that the tephras come from the Izu volcanic arc front. Because lava flows and pillow lavas cannot travel far from their source, provenance of bulk samples (e.g., mature arc magmas depicted as white circles with a red outline, and infant arc magmas depicted as yellow circles in Figures 2–9) is not examined further.
FIGURE 3. Fractionation trends. (A) CaO (wt%), and (B) Al2O3 (wt%) vs. MgO (wt%) diagrams. The kink in MgO ∼6–7 wt% in the basaltic lavas marks the onset of plagioclase (plag) and clinopyroxene (cpx) fractionation, implying that basalts with MgO ≥6–7 wt% are likely to have only crystallized olivine along their liquid line of descent, so they may have retained their primary composition. WR, whole rock
FIGURE 4. Volatile contents over time. (A) H2O (wt%), (B) Cl (ppm), (C) F (ppm) contents of the Izu basaltic glass shards vs. time. Thick green lines from 0 to ∼5 Ma and from 15 to 29 Ma depict the opening of the back-arc rift and Shikoku Basin, respectively. A gap in is observed during opening of the Shikoku back-arc Basin, suggesting that the basaltic magmatic activity was interrupted during that interval of time. BAB: back-arc basin.
FIGURE 5. Chondrite-normalized rare Earth element patterns. (A) Izu basaltic glass shards (red compositional field); and (B) infant arc basalts (whole rock in yellow compositional field). Representative REE patterns for the Izu mature arc and for the Izu infant arc are also depicted as thick red lines (A) and a thick black line (B), respectively. Diagrams are normalized using the chondrite contents of Sun and McDonough (1989). The orange compositional field in panel A represents the mature arc magmas (bulk analyses) from the compiled dataset of DeBari et al. (2020). Detailed REE patterns can be found in the Supplementary Material.
FIGURE 6. Classification diagrams for sample provenance. (A) La/Yb and (B) La/Sm vs. time. The diagrams are used to examine the provenance of the volcaniclastics examined in this study. Some of the Izu volcaniclastics possess higher La/Sm and La/Yb ratios than most of the volcaniclastics from the volcanic arc front, as depicted by the pink compositional field. High La/Yb and La/Sm are characteristics of the rear-arc magmas, suggesting that the outliers may come from the rear-arc.
FIGURE 7. Temporal evolution of the degree of mantle melting and mantle depletion. (A) TiO2 (wt%), (B) Nb/Yb, (C) TiO2/Y, (D) Na6 (wt%) diagrams used to examine the compositional evolution of the mantle source (Nb/Yb and TiO2/Y) and extent of melting (TiO2 and Na6) over time. Some of the outliers may belong to the rear-arc, as suggested in Figure 6. The blue line represents an averaged composition of the proxies with 1σ error bar.
FIGURE 8. Temporal evolution of the slab fluids. The slab fluid compositions are inferred from markers of slab dehydration, Ba/Th (A) and Rb/Th (B), and sediment melts, Th/Nb (C), as in (Pearce et al., 2005). (D) Temporal variations in H2O/K2O is also used to track the compositional fluctuations of the water-rich slab fluids over time. The slab-fluid proxies display little variations over time. SI: subduction inception. The blue line represents an averaged composition with 1σ error bar.
FIGURE 9. Comparison between the markers of climate variations and slab dehydration for the past 45 Ma. (A) Markers of past climate variations δ18O and δ13C (Zachos et al., 2001). The general decrease in δ18O since the middle Eocene reveals a decrease in the Earth’s surface temperatures for the past 45 Ma (Zachos et al., 2001). (B) Cs/Ba and (C) Rb/Th ratios used to track the aqueous (volatile-rich) slab fluids released underneath the Izu arc for the past 45 Ma. The blue line represents an averaged composition of the markers of slab dehydration with 1σ error bar. There is a slight increase in the markers of slab dehydration at ∼ 40Ma (i.e., from the infant to the mature arc); while the long-term averages in the slab-fluid proxies have remained relatively constant in the mature Izu arc from 40 Ma.
The volcanic arc front, the rear arc, the back-arc rifts and back-arc knolls in Izu display distinct geochemical features, which can be used to distinguish the geodynamic settings of the volcaniclastics (Gill, 1981). For instance, Izu arc lavas are characterized by low K2O content <1.5 wt%, La/Sm < 1.3 and La/Yb < 1.2, as compared to the back-arc and the rear arc magmas (Gill, 1981; Heywood et al., 2020). By contrast, rear-arc magmas possess La/Yb > 2, La/Sm > 1.5, along with higher K2O content (Figure 2). As such, magma composition can be used to distinguish the provenance of tephras (Gill, 1981; Heywood et al., 2020). Because our goal is to examine the temporal variations of arc magma composition, we must ensure that our filtering will not reduce any compositional variations that could be associated to arc evolution. Therefore, our glass dataset is not filtered any further to minimize any bias that could be associated to filtering. The recent classification of Heywood et al. (2020) is only utilized to assess the provenance of the tephras, and to examine the validity of our approach. The volcaniclastics examined here are classified as volcanic arc, rear arc and back-arc solely based on their classification in the literature (Bryant et al., 2003; Straub, 2003; Straub and Layne, 2003; Straub et al., 2004; Barnes and Straub, 2010; Straub et al., 2010; Schindlbeck et al., 2018a; Kutterolf et al., 2018).
Most of the volcaniclastics plot within the compositional field of the Izu volcanic arc front. 97.4% (35 tephras have La/Yb > 1.2, as highlighted in blue in Supplementary Table S1) of the filtered volcaniclastics and mineral-hosted melt inclusions plot within the arc compositional fields of Heywood et al. (2020) (Figure 2), demonstrating that our selected volcaniclastics (red circles) mostly belongs to the Izu volcanic arc front. This approach is mostly valid for the mature volcanic arc front (red circles), as infant arc magmas (yellow circles) display distinct geochemical features that is further examined below. We also find that some of the screened volcaniclastics could belong to the rear arc based on their chemistry, as discussed below.
Some of the bulk volcaniclastics from Gill et al. (1994) and Hiscott and Gill (1992) (large white circles with a red outline) display a wide compositional range, along with higher La/Sm and La/Yb than observed for the Izu volcanic arc front (Figures 2, 3). This compositional variability could reflect the fact that the bulk analyses integrate a mixture of lithics and free crystals that could derive from several sources. Alteration could also play a role in the composition of some of the incompatible elements of the bulk volcaniclastics, including Na, K, Rb, Cs, La. Therefore, bulk volcaniclastics are examined with caution.
In the K2O-SiO2 classification diagram (Peccerillo and Taylor, 1976), the samples are low-K basalts to basaltic andesites (K2O < 1 wt%) that mostly plot within the arc compositional field (Figure 2). The infant arc magmas (yellow circles in Figure 2), that formed around 45–40 Ma (i.e., within ∼5–10 Ma of subduction inception), generally display higher K2O content than the more mature arc magmas (red circles in Figure 2) that developed from 40 Ma. The infant and mature arc magmas possess MgO content ranging from 2 to 9 wt%, CaO content ranging from 7 to 14 wt% and Al2O3 content ranging from 10 to 21 wt% (Figure 3). The bulk rocks from the mature arc magmas (white circles with a red outline) display higher CaO and Al2O3 contents than the Izu arc glasses at the same MgO content, which likely reveals the effect of crystal fractionation (Figure 3). Indeed, in situ analyses of fresh glasses tend to minimize the effects of crystal fractionation, which are often revealed by lower contents in some of the major elements in the glasses.
The sharp decrease in CaO content with decreasing MgO content, that is associated with a change in Al2O3 content, reveals a kink in arc magma composition at MgO ∼6 – 7 wt% (as depicted by a thick orange line in Figure 3). This kink marks the onset of plagioclase and clinopyroxene crystallization in the arc magmas. Hence, arc magmas with MgO content ≥6 – 7 wt% mainly crystallized olivine on their liquid line of descent, so they may be considered in near equilibrium with their mantle source. The infant arc magmas and the more mature arc magmas (bulk samples) plot along similar liquid lines of descent (Figure 3).
The basaltic glass shards and the mineral-hosted melt inclusions display variable volatile content in water (0.5–5.4 wt%), Cl (50–2,850 ppm), F (72–260 ppm) (Figure 4). Their water content is consistent with the water content recorded in arc magmas (e.g., 0.19–6.14 wt% H2O in the Marianas) (Ruscitto et al., 2012; Plank et al., 2013; Ribeiro et al., 2015). The fractionation trends in H2O content plotted against indices of fractionation (e.g., SiO2 and K2O; Supplementary Figure S1) demonstrate that the basaltic glasses have preserved their original composition (see Supplementary Material). Volcanic degassing, alteration and diffusion between the crystal host and the melt inclusions may thus play a minor role in the glass composition. These fractionation trends further demonstrate that the glass shards and the mineral-hosted melt inclusions may have mostly preserved a reliable water content.
Most of the basaltic glasses from the mature Izu arc display convex chondrite-normalized rare Earth element (REE) patterns with depletion in light REE (LREE) (Figure 5 and Supplementary Material for details). The basaltic glasses from the mature arc also possess flat heavy REE (HREE) patterns. Some of the basaltic glasses from the mature Izu arc are, instead, enriched in LREE as compared to the HREE, as reflected by their higher La/Yb and La/Sm ratios (>1 in Figure 5). They also display a negative Eu anomaly that is characteristics of plagioclase fractionation (Figure 5A). Their enrichment in LREE might reveal their rear-arc provenance, as suggested by Heywood et al. (2020) (Figure 2). The infant arc magmas display, instead, REE patterns that are both enriched and depleted in LREE as compared to the HREE (Figure 5B). They also show a negative Eu anomaly and flat HREE patterns.
Fresh basaltic glass shards and mineral-hosted melt inclusions from the Izu arc (both depicted as red circles in Figure 2) generally have La/Yb < 1.2 and La/Sm < 1.3. Only a few outliers possess La/Yb > 1.2 and La/Sm > 1.3 and plot within the compositional fields of the rear-arc and back-arc magmas (Heywood et al., 2020). Their higher ratios in La/Yb and La/Sm may reveal their rear-arc provenance (Figure 6). Infant arc magmas from the Bonin Islands display, instead, higher La/Sm and La/Yb ratios which overlap the compositional fields of the rear-arc, the back-arc and the arc magmas (Figure 2). Their enrichment in these ratios may reveal their formation during distinct petrogenetic processes (Ishizuka et al., 2006; Ribeiro et al., 2019; Ishizuka et al., 2020).
Understanding the past variations in CO2 fluxes released during arc volcanism (i.e., CO2 outfluxes) is essential to comprehend the processes that modulate climate changes. The CO2 outfluxes in volcanic arcs Farc (g/yr/km of trench) are modulated by the arc magma addition rate M (km3/Ma/km of trench), the arc length L (km) and the pre-eruptive CO2 content of the arc magma CCO2 (ppm), such as:
ρ is the density of the crust (g/cm3). Arc magma addition rate M is defined as the volume of arc magmas that is added to the crust since arc formation, so M largely depends upon the variations in arc crustal thickness (km) over the lifetime of an arc. Arc magma addition rates are usually estimated from seismic crustal profiles (Holbrook et al., 1999; Dimalanta et al., 2002; Calvert et al., 2008), and their temporal variations remain poorly known. As such, most estimates of past CO2 outfluxes in island arcs consider that the arc magma rate M has remained relatively homogeneous over the lifetime of an arc (Johnston et al., 2011; Wong et al., 2019). Yet, whether M remains constant over time has remained largely untested. Additionally, such a magma rate does not account for the volume of arc magmas that is removed by back-arc rifting, crustal erosion and crustal delamination, so M is largely underestimated (Jicha and Jagoutz, 2015). Additional uncertainties come from the CO2 content of the magmas. Direct examinations of the temporal variations in the CO2 content of arc magmas have proven challenging due to a lack of high-resolution temporal records in volcanic glasses (or mineral-hosted melt inclusions) that have preserved a reliable CO2 content (Barnes and Straub, 2010; Straub and Layne, 2002, 2003). About 90% of the magmatic CO2 may also degas upon magmatic ascent (Cartigny et al., 2008; Tucker et al., 2019), so that in situ analyses may not reliably track the pre-eruptive CO2 content. As such, assessing the temporal variations in the volatile outfluxes (CO2, S, H2O) requires a better understanding of the magmatic history of island arcs.
To fill this scientific gap, here we examine the magmatic activity of the Izu arc shortly after its inception. Infiltration of slab fluids into the sub-arc mantle triggers mantle melting, which results in arc magmas (Grove et al., 2006). Therefore, temporal variations in the extent of mantle melting, length of the melting column, arc crustal thickness, mantle source composition, and slab-fluid composition play a first-order control on arc magma composition, arc magmatic activity and the extent of volatiles released by arc volcanism. Chemical proxies are powerful tools to track the compositional changes in mantle and subduction processes in arc basalts through time (Pearce et al., 2005). Therefore, here we use such chemical proxies i) to examine the magmatic history of the Izu arc over its lifetime; and ii) to explore the past evolution of slab-derived CO2 outgassed during island arc volcanism. Finally, we discuss whether island arc volcanism has played an important role on long-term climate variations (≥10 Ma) for the past 45 Ma.
The CO2 outgassed by arc volcanism is modulated by the magma supply rate M Eq. 1, that is the volume of arc magmas added every million years to the arc crust. Therefore, M co-varies with the arc crustal thickness throughout the arc lifespan. Yet, assessing the temporal variations in arc crustal thickness from direct observations (e.g., geophysical survey, seismicity) remains challenging, so we must rely on chemical markers. Chemical elements, such as Na6, La/Sm, La/Yb in arc basalts, are positively correlated with arc crustal thickness in worldwide arc volcanoes (Turner and Langmuir, 2015a, b). This coherent systematics implies that the length of the melting column plays a first-order control on arc magmatic activity. The thickness of the arc lithosphere controls, in return, the extent of mantle melting and the magma addition rate M. For instance, arc volcanoes with longer melting columns (i.e., thinner arc crust) produce magmas at higher degrees of mantle melting (Turner and Langmuir, 2015a; Turner et al., 2016). By contrast, arc volcanoes with a thicker crust will have a shorter melting column, and arc magmas are produced at lower extents of mantle melting (Turner and Langmuir, 2015b). Therefore, the temporal variations in the length of the melting column modulate the arc magmatic activity and the arc crustal thickness over the lifetime of an arc. Below, we further use the temporal variations in these chemical markers to place constraints onto the past variations in arc magma addition rates M in the Izu intra-oceanic arc.
TiO2 is a compatible element that migrates with the melt during mantle melting, while it remains relatively immobile with the slab fluids. TiO2 in fresh glasses is negatively correlated to the degree of mantle melting, so it can also be used to track the extent of mantle melting in arc magmas (Kelley et al., 2006). Na6 is also utilized to track the extent of mantle melting in arc magmas (Klein and Langmuir, 1987; Kelley et al., 2006), as well as to examine the variations in the length of the melting column (Turner and Langmuir, 2015a, b). Na6 content was calculated by bracketing the Na2O content of arc basalts with MgO contents varying between 6 and 7 wt%, following the method of Turner and Langmuir (2015a, b). This method has the main advantage of using the measured values of Na2O at MgO ∼6 – 7 wt%, as opposed to a value corrected from a linear regression (Klein and Langmuir, 1987; Kelley et al., 2006). La/Sm and La/Yb are used to track the length of the melting column, and infer temporal variations in arc crustal thickness (Turner and Langmuir, 2015a, b).
The increase in TiO2 content from the infant to the mature arc suggests that the extent of mantle melting began to decrease as the arc became mature (within ∼5–10 Ma of arc inception). Alternatively, the increase in TiO2 content can also be explained by the influx of a more fertile asthenospheric mantle during arc maturation, as supported by the Nb/Yb variations (Figure 7B). Once the arc has reached maturity (∼40 Ma ago), the chemical markers (TiO2, Na6) suggest that the extent of melting has remained relatively homogeneous (Figures 7A,D) for millions of years. A gap in the volcanic record from 25–15 Ma (Figure 7) suggests that arc magmatism was interrupted or reduced for ∼10 Ma in Izu. This short period of magmatic quiescence (23–20 Ma) coincides with the opening of the Shikoku back-arc Basin (Taylor, 1992) that initiated ∼29–26 Ma ago (Okino et al., 1994; Ishizuka et al., 2011b), as depicted by the thick green line in Figure 7.
La/Sm, La/Yb and Na6 from the mature Izu arc basalts (>40 Ma) plot within a homogeneous compositional field (pink field in Figures 6, 7D), implying a relatively steady arc crustal thickness over the lifetime of the mature Izu arc. Such observations imply that crustal thickening is rapidly achieved during arc infancy (from ∼10 to ∼20 km thick within ∼5–10 Ma of subduction inception) in Izu (Kodaira et al., 2010; Ishizuka et al., 2011a). The chemical proxies used to track the length of the melting column (Na6, La/Sm, La/Yb) remain relatively constant in average in the mature arc, suggesting that once the arc has reached maturity, it has likely preserved its crustal thickness (∼20 km thick) (Kodaira et al., 2010) for millions of years (Figure 7D). Similar crustal thicknesses have been observed in the modern Mariana arc using seismicity (Takahashi et al., 2007).
Despite short-term cyclicities (Gill et al., 1994), the relatively homogeneous long-term averages in the markers of mantle melting and length of the melting column demonstrate that the magmatic activity and the crustal thickness of the Izu arc have remained nearly homogeneous over its lifetime. Such results further suggest that arc magma addition rate M in the Izu arc may have remained relatively steady for the past 40 Ma. Such a long-term steadiness implies that any new supply of arc magmas added by mantle melting to the arc crust is rapidly removed by crustal erosion (von Huene and Scholl, 1991) and back-arc stretching (Jicha and Jagoutz, 2015).
The asthenospheric mantle plays a central role in fingerprinting the composition of arc magmas. Because the asthenospheric mantle also contains some CO2 (Cartigny et al., 2008; Dasgupta and Hirschmann, 2010), variations in the mantle composition may result in variations in the CO2 content of arc magmas. Hence, examining temporal variations in mantle source composition is essential to examine how CO2 outgassed by island arcs may have varied over the arc lifetime.
Nb and Yb are incompatible elements that both remain relatively immobile with the slab fluids, but they are easily mobilized during mantle melting. Nb and Yb have similar partition coefficients during crystal fractionation, but Nb migrates slightly faster than Yb with the mantle melts. Hence, the Nb/Yb ratio has the potential to track mantle depletion and mantle enrichment, as well as the extent of mantle melting (Pearce et al., 2005). Similarly, TiO2/Y ratio can also be used to track mantle enrichment and depletion (Kelley et al., 2006). However, TiO2 is less mobile than Nb during mantle melting; therefore, it is a slightly less sensitive marker to the variations in mantle source composition than Nb. TiO2/Y and Nb/Yb ratios can thus be used to track compositional variations in the mantle source provided that garnet is not a residual phase, as garnet strongly partitions the HREE. The flat HREE patterns of the infant and mature arc magmas (Figure 5 and Supplementary Material for details) demonstrate that garnet is not a residual phase in the Izu arc basalts. Variations in Na6 and TiO2 contents of the Izu arc basalts suggest that the extent of mantle melting has remained relatively homogenous for the past 40 Ma (Figures 7A,D), so TiO2/Y and Nb/Yb proxies can be used to reliably track the temporal variations in mantle depletion-enrichment.
The high Nb/Yb ratios (∼1) of the infant arc magmas (Figure 7B) suggest the influx of relatively fresh and undepleted asthenospheric mantle during arc infancy around 45 Ma (i.e., ∼5 Ma of subduction inception). The decrease in Nb/Yb from the infant to the mature arc magmas (from ∼1 to less than 1) implies that the sub-arc mantle is progressively depleted due to continuous melt extraction (Figures 2D, 7B) during arc maturation. As the arc reached maturity around 40 Ma, the Nb/Yb ratios (0.1–0.3) remained approximately constant throughout the lifespan of the Izu arc, with the exception of a few basaltic glasses with ages <3 Ma (Nb/Yb ∼ 1) (Figure 7B). A small decrease in the Nb/Yb ratios of the basaltic glasses (from ∼0.4 to 0.2) might also exist around 25 Ma. This decrease in Nb/Yb ratios could reveal a mantle depletion that might be associated to opening of the Shikoku Basin between 29 and 26 Ma (Gill et al., 1994; Bryant et al., 2003) (Figure 7). The TiO2/Y ratio (∼100–600) seems to have remained relatively homogenous throughout the lifespan of the Izu arc, with the exception of a few recent basaltic glasses (age <3 Ma) that show higher TiO2/Y (∼1,400). The increase in Nb/Yb (∼1) and in TiO2/Y (∼1,400) in the mature arc magmas for the past 3 Ma could reveal the recent inflow of asthenospheric mantle underneath the Izu arc (Figures 7B,C). Alternatively, those basaltic volcaniclastics could come from the rear-arc, whose magmas are characterized by a depletion in the HREE as compared to the arc magmas (Heywood et al., 2020).
Once the Izu arc has become mature (i.e., from ∼40 Ma), the relatively homogeneous Nb/Yb (0.01–0.03) and TiO2/Y (∼100–600) ratios suggest that the sub-arc asthenospheric mantle is continuously replenished by fresh and relatively fertile inflow of asthenospheric mantle over time (Hochstaedter et al., 2000). Continuous extraction of arc magmas from the sub-arc mantle would thus be rapidly balanced by mantle convection that would bring continuously fresh inflows of asthenospheric mantle underneath the mature arc (Magni, 2019). Mantle convection thus enables the sub-arc mantle to remain relatively fresh and undepleted over time, so it may continue melting without suffering of extensive mantle depletion (Hochstaedter et al., 2000; Straub et al., 2010; Magni, 2019). However, shorter-term variations in mantle flows and mantle source composition may still exist in the mature arc. For instance, opening of the Shikoku back-arc Basin likely depleted the sub-arc asthenospheric mantle, as suggested by a small decrease in the Nb/Yb at ∼ 25 Ma (Bryant et al., 2003) (Figure 7B). The mantle flowing underneath the arc would thus be progressively depleted, due to prior melt extraction underneath the back-arc basin. Once the back-arc basin stops spreading, slab-driven mantle flow is restored, bringing a new and fresh inflow of asthenospheric mantle underneath the Izu arc (Hochstaedter et al., 2000; Magni, 2019). The relative constancy in the long-term averages in markers of mantle source composition further demonstrates that these short-term variations in mantle flows and mantle composition are rapidly counterbalanced (i.e., within 1–5 Ma). Hence, the mantle flowing underneath the mature Izu arc may maintain a relative homogeneous composition over long periods of time (Hochstaedter et al., 2000; Nikolaeva et al., 2008; Straub et al., 2010; Straub et al., 2015).
Slab fluids are major carriers of volatiles (H2O, CO2, S, Cl) at subduction zones. As the slab fluids infiltrate the sub-arc asthenospheric mantle during dehydration and melting of the subducted plate, they trigger mantle melting which results in arc magmas (Grove et al., 2006). Arc magmas thus capture the slab-derived volatiles released upon mineral breakdown (Peacock, 1990; Schmidt and Poli, 1998; Hilton et al., 2002). Mantle melting and melt extraction beneath the arc occur as long as the slab fluids are being released into the sub-arc asthenosphere (Kelley et al., 2010), so arc magmatic activity and arc crustal growth are associated with slab dehydration and slab melting.
Dehydration and melting of the subducted plate can be tracked using chemical markers. For instance, elemental ratios of incompatible elements that are easily mobilized with the water-rich slab fluids (Cs, Ba, Rb) and with the slab melts (Th) over elements that remain rather immobile with the slab fluids (Nb, Ta, Yb) have the potential to track the slab fluids that infiltrated the sub-arc mantle (Elliott, 2003; Pearce et al., 2005). Hence, Ba/Nb, Rb/Nb and Ba/Yb ratios are commonly used to track the total contribution in slab fluids (both aqueous slab fluids and sediment slab melts); while Th/Nb ratios solely track the slab melt contribution (Kogiso et al., 1997; Johnson and Plank, 1999; Pearce et al., 2005). Using incompatible element ratios that have similar partition coefficients during mantle melting and crystal fractionation has the advantage of minimizing these processes, which can obscure the subduction signal in the magma composition. Slab dehydration is tracked by using ratios of incompatible elements that are mobilized with the water-rich slab fluids (Cs, Ba, Rb) over Th, as Th is mainly mobilized with the slab melt (Pearce et al., 2005). Therefore, the Ba/Th, Rb/Th, Cs/Th ratios minimize the contribution from the sediment slab melts, so they mainly track the water-rich slab fluids. Finally, because Cs is more easily mobilized with the aqueous slab fluids released during mantle deserpentinization, as compared to Ba, Cs/Ba ratio can be used to track the water-rich fluids released from the subducted, serpentinized mantle.
Using these systematics, we find that the infant arc magmas display slightly lower Ba/Th, Rb/Th, Cs/Th and Th/Nb than the mature Izu arc glasses (Figures 2D, 8). The increase in the slab-fluid markers from the infant to the mature arc reveals an increased contribution in the water-rich slab fluids and sediment slab melts during arc maturation (i.e., from 45 to 40 Ma). There is also a sharp increase in Ba/Th between 5 and 15 Ma (Figure 8C) that is not observed in the other markers of water-rich slab fluids (Rb/Th, Ba/Nb and Cs/Ba), suggesting that these high Ba/Th ratios might reveal other processes (e.g., alteration). Rb/Th, Ba/Nb, Cs/Ba and Th/Nb (except for two samples with high Th/Nb) plot, instead, within a homogeneous compositional field for the past 40 Ma (pink compositional field in Figures 8, 9). The relatively homogeneous composition of the slab-fluid markers over long time period suggests rapid feedbacks to these short-term variations.
To summarize, we have examined the composition of the Izu arc basaltic glasses to track its long-term magmatic history using chemical markers. We have shown that:
The temporal variations in the chemical proxies in the length of the melting column (Na6, La/Sm, La/Yb), and hence, in the arc crustal thickness, in the extent of mantle melting (Na6, TiO2), in the mantle composition (Nb/Yb, TiO2/Y) and in the slab-fluid composition (Ba/Th, Rb/Th, Ba/Nb, Cs/Ba and Th/Nb) have varied within restricted ranges over long periods of time (>5–10 Ma).
The long-term averages in these chemical makers have relatively little varied in the Izu arc for the past 40 Ma, implying that shorter-term variations (<5 Ma) in mantle and subduction processes are rapidly adjusted, so the mature arc maintain a balance (i.e., steady-state magmatic activity) over long timescales (>5–10 Ma).
Such a long-term steadiness in averaged arc magma composition is consistent with the fact that, despite shorter-term cyclicities, the mature Izu arc has maintained a long-term steady-state magmatic activity for ∼40 Ma (Ishizuka et al., 2006; Straub et al., 2010; Straub et al., 2015). This long-term steady state in arc magmatic activity does not mean that the mantle and the subduction processes have remained constant over the lifetime of the mature Izu arc. Instead, it means that the mantle and the subduction processes have varied within a restricted range over long time periods; and any short-term perturbations are rapidly counterbalanced (within 1–5 Ma). Hence, such a long-term steady state has enabled the mature Izu arc to stabilize and to consolidate in its current location, so continuous arc magma supply contributes to thickening and differentiation of the arc crust (Ribeiro et al., 2019).
The Izu-Bonin-Mariana (IBM) convergent margin is an example of a self-sustained subduction zone, where the volcanic arc front has reached maturity within 10 Ma of subduction inception (Ishizuka et al., 2006; Ishizuka et al., 2011b). The mature IBM arc has maintained its current location at ∼ 150–200 km from the trench for the past 40 Ma (Stern et al., 2003). The fact that the mature Izu arc has preserved its location and a crustal thickness of ∼20 km (Figure 7) for tens of millions of years implies that, despite back-arc opening and short-term variations in arc magmatic activity, the Izu arc has maintained a steady-state magmatic activity over long periods of time. Mature arc volcanoes from worldwide subduction zones have also preserved their location at a slab depth of roughly 112 ± 19 km (Tatsumi, 1986) or 124 ± 38 km (Gill, 1981) for millions of years. Such observations concur with the notion that long-lived intra-oceanic subduction zones with a mature arc may also maintain a long-term steady-state magmatic activity over long period of times. Hence, short-term variations in mantle flows, slab fluids, extent of mantle melting, length of melting column and arc crustal thickness are rapidly adjusted, so mature island arcs may preserve a relatively steady location over long periods of time to stabilize, consolidate and develop. However, some subduction zones do not become self–sustained, and they do not reach a steady state in arc magmatic activity (Gerya et al., 2008; Lallemand and Arcay, 2021). Instead, they abort shortly after their inception, such as the Troodos supra-subduction zone ophiolite in Cyprus (Pearce and Robinson, 2010). A recent compilation showed that about 24 modern intra-oceanic subduction zones became self-sustained within ∼10 Ma of their inception (Lallemand and Arcay, 2021), implying that their associated arcs rapidly reached maturity and stabilized in their current location to consolidate. Such evolution is consistent with the evolution of the IBM arc (Ishizuka et al., 2006; Ribeiro et al., 2019; Ishizuka et al., 2020), implying that the Izu arc may be representative of mature island arcs. Because arc stabilization is essential to achieve crustal thickening and arc maturation (Ribeiro et al., 2019), most worldwide mature island arcs from long-lived subduction zones may also have developed a long-term steady state in arc magmatic activity to maintain a balance in the mantle and subduction processes over long time periods. A long-term steady-state in arc magmatic activity could thus be essential in the development and maturation of infant arcs, so they could preserve a relatively steady location over long periods of time.
Mature island arcs may maintain a long-term steady-state magmatic activity unless the subduction zone is affected by i) plate tectonic reorganization; ii) back-arc basin opening; iii) collision or seamount/ridge subduction (Wu and Wu, 2019) and iv) slab rollback. However, back-arc basin opening and ridge subduction will interrupt the magmatic activity for only a short period of time (≤10 Ma) (Figure 4) (Taylor, 1992; Wu and Wu, 2019). Once mantle convection is restored, arc magmatic activity will initiate again, producing arc magmas with a similar composition to those that developed before the onset of back-arc spreading. So over long-time periods, averaged arc magma composition has only little varied. Such a scenario has been observed in the Izu arc (Figure 6). A change in the slab composition and mantle inflow will also lead to a change in the composition of the arc magmas. But arc magma composition rapidly adjusts to these new conditions (within 1–5 Ma) until a new balance is reached. For instance, the collision-subduction of seamount chains at the Mariana Trench ∼10 Ma ago resulted in an enrichment in Pb isotopic ratios and K2O content in the Mariana arc magmas (Straub et al., 2015). This enrichment has been preserved as long as the seamount chains have been subducting at the Mariana Trench. Hence, the long-term composition of the Mariana arc magmas has remained relatively homogeneous for the past 10 Ma, supporting the notion of a long-term steady-state magmatic activity in mature island arcs. Unless the subduction zone aborts or collides onto a continent or with another arc, arc magmatic activity may persist over long periods of time.
Correlations between the CO2/ST ratios of volcanic gas from worldwide island arcs with markers of slab fluids (e.g., Ba/La, U/Th, Sr/Nd; Figure 10) (Aiuppa et al., 2017; Aiuppa et al., 2019) demonstrate that i) slab dehydration and slab melting play first-order controls on the CO2 outgassed by island arc volcanism (Kerrick and Connolly, 2001; Ague and Nicolescu, 2014; Skora et al., 2015; Vitale Brovarone et al., 2018), where crustal contamination is minimal (Lee et al., 2013; Aiuppa et al., 2017; Mason et al., 2017); and ii) outgassed CO2 in island arcs may mainly come from the slab fluids released from dehydrating and melting the subducted sediments and the altered oceanic crust (Sano and Marty, 1995; Aiuppa et al., 2017). Hence, slab dehydration and slab melting drive decarbonation and carbonate dissolution of the subducted slab (Ague and Nicolescu, 2014; Skora et al., 2015; Vitale Brovarone et al., 2018), and some of the subducted carbon is released back to the surface via island arc magmatism (Kelemen and Manning, 2015; Aiuppa et al., 2019). The relationship inferred between the markers of the slab fluids and the CO2 outgassed by island arc volcanism (Sano and Marty, 1995; Aiuppa et al., 2014; Aiuppa et al., 2017; Aiuppa et al., 2019) suggests that the markers of slab dehydration and slab melting (Figure 10) may be used to track the long-term temporal variations in slab-derived CO2 released by island arc volcanism, where crustal assimilation is minimal.
FIGURE 10. Relationship between the CO2/ST outgassed in worldwide arc volcanoes and the slab-fluid markers Ba/La of Aiuppa et al. (2017). Arc volcanoes were grouped according to their CO2/ST ratios, so that arc volcanoes outgassing large fluxes of CO2 have the highest CO2/ST (Aiuppa et al., 2017). Such arc volcanoes are mostly continental arcs and they plot within the crustal fluid domain (light red compositional field), implying that the largest CO2 fluxes outgassed by volcanic arcs mostly come from crustal contamination, as emphasized by Lee et al. (2013). By contrast, island arc volcanoes that have experienced little crustal contamination, such as the Mariana arc, possess lower CO2/ST ratios that plot within the slab fluid domain (light blue field). The positive correlation between the CO2/ST ratios outgassed in these island arcs (depicted as green circles and yellow triangles) and the slab-fluid markers (Ba/La) demonstrates that the CO2 released in volcanic arcs mainly derive from the subducted slab.
The long-term steadiness in arc magmatic activity in Izu, as suggested by chemical markers (Na6, La/Sm, Nb/Yb, …), supports the notion that the variations in the outgassed CO2 (and hence, CO2/ST) are mainly modulated by the variations in the slab fluid composition over long timescales. The homogenous long-term markers in water-rich slab fluids (Ba/Th, Rb/Th, Cs/Ba) and sediment slab melts (Th/Nb) further imply that the slab-derived CO2 outgassed by the Izu mature arc may have remained relatively homogeneous over long time periods. Such results suggest that the composition of the subducted Pacific slab in Izu might have remained relatively homogeneous for the past 40 Ma, as any variations in the slab composition would be captured by the arc magmas. Continuous eruptions of arc magmas in Izu thus provide a continuous supply of fresh, weatherable rocks on the surface over time, which draws down CO2 within ∼300 kyr after the onset of volcanism (Pogge von Strandmann et al., 2013). Hence, degassing of volcanic CO2 is rapidly balanced by silicate weathering (within ∼200–300 kyr), precipitation of marine carbonates, and burial of organic carbon (Berner and Caldeira, 1997; Allègre et al., 2010). Tectonic erosion of the arc and fore-arc crust may also contribute to rapidly draw down CO2 (Gaillardet et al., 1999; Pogge von Strandmann et al., 2010) via carbon burial, providing an additional return pathway for carbon into the mantle (Straub et al., 2020; von Huene and Scholl, 1991).
Although we have only focused on the Izu arc, this arc belongs to the northern part of the larger Izu-Bonin-Mariana system. In the Eocene, the IBM intra-oceanic arc further extended to the south and it was likely associated to the Tonga-Fiji-Kermadec intra-oceanic arc, representing more than 4,000 km of arc length ∼45 Ma ago (Gill, 1987; Todd et al., 2012; Wu et al., 2016). Since the Cenozoic, 24 intra-oceanic subduction zones have reached maturity and became self-sustained, covering ∼39,360 km trench length total (Figure 11) (Lallemand and Arcay, 2021). Therefore, more than half of the modern intra-oceanic subduction zones have developed a mature arc during the Cenozoic. Although a high-resolution record of the magmatic sequences does not yet exist for most active island arcs, the simple fact that these mature island arcs have maintained their current location for tens of millions of years (Gill, 1981; Tatsumi, 1986) implies that, far from collision zones or ridge/seamount subduction, the arc magmatic activity may have reached a steady state over long timescales. This long-term steady state implies that the sub-arc mantle has been continuously replenished by the inflow of fertile asthenospheric mantle (Magni, 2019); and the arc magmas have captured the slab fluids (and slab-derived volatiles) released from the same portion of the slab (Straub et al., 2010; Straub et al., 2015).
FIGURE 11. Global map showing the self-sustained intra-oceanic subduction zones during the Cenozoic epoch. 24 oceanic subduction zones (in red) have been proposed to have reached the self-sustained state since the Cenozoic, which corresponds to ∼39,360 km cumulative trench length (Lallemand and Arcay, 2021). The world map was made with GeoMapApp (http://www.geomapapp.org).
If worldwide mature island arcs also maintain a long-term steady-state magmatic activity (i.e., constant M in Eq. 1) like in Izu, their long-term CO2 outfluxes may be mainly modulated by the island arc length L in Eq. 1 and the flux of CO2 entering the trenches Fin (g/yr/km of trench), that is defined such as:
Assuming a steady state in the global C cycle over long timescales (Kelemen and Manning, 2015), Fin is balanced by the fluxes of CO2 emitted at the arc Farc and at the back-arc Fba in Eq. 2. Fx is the flux of remaining CO2 in the residual slab that bypasses the generation depth of subduction zone magmas and is recycled into the lower mantle. Fin includes the carbonates in the subducted sediments, altered oceanic crust and lithospheric mantle Eq. 3. H is the thickness of the lithosphere (km), CCO2 is the concentration of CO2 (ppm), ρ is density (g/cm3) and uo is the convergence rate (mm/yr). The length of worldwide oceanic subduction zones L has been proposed to have increased for the past 60 Ma, as several subduction zones initiated in the Western Pacific during the early Cenozoic (Matthews et al., 2016; Müller et al., 2016). Additionally, sedimentary carbonate entering the trenches may have also increased since the Late Cretaceous, resulting in an increase in the CO2 influxes Fin for the past 100 Ma (Dutkiewicz et al., 2018; Wong et al., 2019). Such an increase in the subducted CO2 Fin and in the worldwide arc length L suggests that the flux of CO2 outgassed by worldwide island arc volcanoes Farc could have increased for the past 45 Ma. Alternatively, an increase in the subducted carbonate flux Fin could also imply that the remaining subducted CO2 flux that is returned to the lower mantle Fx might have increased during the Cenozoic Eq. 2. Both scenarios are not mutually exclusive and require further investigations.
A long-term steadiness or a long-term increase in the CO2 fluxes emitted by worldwide island arc volcanoes Farc cannot be easily reconciled with the long-term cooling temperatures and the onset of high latitude glaciation (icehouse climate) that prevail during the Cenozoic (Berner, 1989; Gaillardet et al., 1999; Pogge von Strandmann et al., 2010; Lee et al., 2016). Additionally, the lack of co-variations between the markers of past climate changes (δ18O, δ13C) (Zachos et al., 2001) and the markers of slab dehydration and slab melting (Ba/Th, Rb/Th, Cs/Ba, Th/Nb), that are believed to track the CO2 outfluxes emitted by island arcs (Aiuppa et al., 2017; Aiuppa et al., 2019), implies that the long-term release of slab-derived CO2 by mature island arcs may not be responsible for the long-term climate fluctuations during the Cenozoic (Figures 7–9). Our observations are at odds with evidences of declining atmospheric pCO2 and increasing silicate weathering for at least the past 40 Ma (Raymo and Ruddiman, 1992; Caves Rugenstein et al., 2019). Furthermore, most island arcs are not intersecting carbonate platforms, so they mostly outgas relatively low to moderate CO2 flux (CO2/ST ≤ 4) in the atmosphere (Lee et al., 2013; Aiuppa et al., 2017; Mason et al., 2017; Aiuppa et al., 2019). Such low to moderate CO2 outfluxes suggest that island arc volcanoes may not be the main contributors to the volcanic CO2 outgassed in the atmosphere. Alternatively, eruption of volcaniclastics during explosive volcanism has been proposed to result in a negative climate feedback, which would enhance climate cooling (Soreghan et al., 2019). However, eruption of volcanic aerosols seems to be too short lived (<5 Ma) to cause long-term climate variations (Black et al., 2015; Soreghan et al., 2019). Finally, an increase in the residual subducted CO2 flux Fx that is recycled into the lower mantle could also draw down the atmospheric pCO2 during the Cenozoic, but this possibility has to be further explored.
Although the origin of the Cenozoic global cooling remains widely debated (Raymo and Ruddiman, 1992; Berner, 1994; Müller et al., 2008; Li and Elderfield, 2013; Lee et al., 2015; Cao et al., 2017), our study suggests that, once island arcs have reached maturity, arc volcanic degassing is likely to be rapidly balanced by tectonic erosion and silicate weathering, which may draw down the outgassed volcanic CO2 within less than 1 Ma (Figure 12B). Therefore, mature island arcs might play a minor role in long-term climate changes; and they may instead contribute to maintain background stability. However, additional studies are required to test the assumption that worldwide island arcs from self-sustained oceanic subduction zones have maintained a steady-state magmatic activity over long timescales. Additionally, we cannot exclude the possibility that island arc degassing may have contributed to shorter-term climate variations (<5–10 Ma), as our study only allows us to examine the long-term variations in arc magmatic activity. Finally, our study highlights the importance of examining the arc magmatic history prior assessing volatile outfluxes, as island arc magmatic activity may not have always been at a steady state (Ishizuka et al., 2011b; Ribeiro et al., 2019; Ishizuka et al., 2020). As such, modern magma rates may not be suitable to infer CO2 outfluxes released during subduction infancy. Island arc infancy is indeed a transient stage that is rapidly evolving (Figure 12A). During that stage, the infant arc does not maintain its location, as it is rapidly displaced away from the trench (Ishizuka et al., 2006; Ishizuka et al., 2011a; Ribeiro et al., 2019). The subducted slab is progressively cooling, while the serpentinized fore-arc mantle is developing (Agard et al., 2016; Ribeiro et al., 2019). Such rapid changes are likely to influence the release of slab-derived volatiles into the sub-arc asthenospheric mantle over time.
FIGURE 12. Sketch of carbon cycle over the lifetime of the Izu intra-oceanic arc. (A) From ∼45–40 Ma, the infant Izu arc rapidly developed. The volcanic arc front is progressively displaced away from the trench until reaching its current location. During that transient stage, the steady state is not maintained. (B) The Izu arc has reached maturity ∼40 Ma ago and maintains its current location at ∼ 150 km from the Izu Trench. The serpentinized fore-arc mantle developed and cools the shallow part of the slab top. The subduction zone is in a quasi steady state and maintains self-sustained conditions over long time periods. During that stage, volcanic degassing of CO2 is readily balanced by silicate weathering and tectonic erosion within 100–300 kyr.
The Izu intra-oceanic arc has rapidly reached maturity and has developed a long-term steady-state magmatic activity within 10 Ma of subduction initiation. Despite short-term cyclicities, the long-term averages in the markers of the mantle source composition (Nb/Yb, TiO2/Y), slab fluids (Ba/Th, Rb/Th, Th/Nb, Cs/Ba), arc crustal thickness (La/Sm, La/Yb) and extent of mantle melting (Na6, TiO2) have remained relatively homogeneous for the past 40 Ma in Izu. Such a long-term steadiness in arc magmatic activity implies that the composition of mantle source and slab fluids released at sub-arc depth have varied within restricted ranges over long timescales in the mature Izu arc. Mature island arcs may be so tuned, so any shorter-term variations in mantle and subduction processes would be rapidly balanced (within ∼1–5 Ma) to maintain a steady-state magmatic activity over long-time periods. This long-term steady state could be essential to arc stabilization, crustal thickening and subsequent arc maturation. The continuous supply of arc magmas into the arc crust, and the subsequent arc crustal growth, might thus be rapidly balanced by tectonic erosion and silicate weathering, so the mature Izu arc maintains a relatively steady arc crustal thickness (∼20 km thick) over long time periods.
The fact that worldwide island arcs from self-sustained subduction zones have maintained their current location for millions of years suggests that they may also have developed a long-term steady state magmatic activity. Such a steadiness in the long-term magmatic history of mature island arcs is at odds with the declining atmospheric CO2 since the early Cenozoic, suggesting that long-term degassing by mature island arcs may have played a minor role into long-term climate variations. The long-term volcanic CO2 outgassed by mature island arcs may be instead rapidly balanced by silicate weathering of freshly erupted magmas and tectonic erosion, which draw down the atmospheric CO2 within a few hundreds of thousand years. Volcanic degassing of CO2 by long-lived island arcs would thus contribute to background atmospheric CO2 over long timescales. This subduction-degassing-weathering cycle may have regulated the exchanges in CO2 between the Earth’s surface and its deeper interior to sustain life and planet habitability over geological times.
The original contributions presented in the study are included in the article/Supplementary Material, further inquiries can be directed to the corresponding author.
JR designed the study, compiled the database, and wrote the manuscript. PP and JR discussed implications on climate changes and weathering. All authors contributed to the manuscript, read, and approved the submitted version.
JR acknowledges a PIFI fellowship from the CAS no 2020VCB000. PP is supported by ERC grant 682760 CONTROLPASTCO2.
The authors declare that the research was conducted in the absence of any commercial or financial relationships that could be construed as a potential conflict of interest.
All claims expressed in this article are solely those of the authors and do not necessarily represent those of their affiliated organizations, or those of the publisher, the editors and the reviewers. Any product that may be evaluated in this article, or claim that may be made by its manufacturer, is not guaranteed or endorsed by the publisher.
JR is grateful to J. Gill (USCS), C.T. Lee (Rice University), J. Ryan (South Florida University), and J. Pearce (Cardiff University) for the thoughtful discussions over the past few years that have inspired this manuscript. J. Wu (Houston University) is also thanked for the discussions on IBM past tectonic evolution. W. Leeman (Rice University) is thanked for inviting us to this special session in Frontiers in Earth Sciences. Jaime Barnes, the editor, and the reviewers are thanked for their insightful comments that have greatly improved this manuscript.
The Supplementary Material for this article can be found online at: https://www.frontiersin.org/articles/10.3389/feart.2022.810597/full#supplementary-material
Agard, P., Yamato, P., Soret, M., Prigent, C., Guillot, S., Plunder, A., et al. (2016). Plate Interface Rheological Switches during Subduction Infancy: Control on Slab Penetration and Metamorphic Sole Formation. Earth Planet. Sci. Lett. 451, 208–220. doi:10.1016/j.epsl.2016.06.054
Ague, J. J., and Nicolescu, S. (2014). Carbon Dioxide Released from Subduction Zones by Fluid-Mediated Reactions. Nat. Geosci. 7, 355–360. doi:10.1038/ngeo2143
Aiuppa, A., Fischer, T. P., Plank, T., and Bani, P. (2019). CO2 Flux Emissions from the Earth's Most Actively Degassing Volcanoes, 2005-2015. Sci. Rep. 9, 5442. doi:10.1038/s41598-019-41901-y
Aiuppa, A., Fischer, T. P., Plank, T., Robidoux, P., and Di Napoli, R. (2017). Along-arc, Inter-arc and Arc-To-Arc Variations in Volcanic Gas CO 2/S T Ratios Reveal Dual Source of Carbon in Arc Volcanism. Earth-Science Rev. 168, 24–47. doi:10.1016/j.earscirev.2017.03.005
Aiuppa, A., Robidoux, P., Tamburello, G., Conde, V., Galle, B., Avard, G., et al. (2014). Gas Measurements from the Costa Rica-Nicaragua Volcanic Segment Suggest Possible Along-Arc Variations in Volcanic Gas Chemistry. Earth Planet. Sci. Lett. 407, 134–147. doi:10.1016/j.epsl.2014.09.041
Allègre, C. J., Louvat, P., Gaillardet, J., Meynadier, L., Rad, S., and Capmas, F. (2010). The Fundamental Role of Island Arc Weathering in the Oceanic Sr Isotope Budget. Earth Planet. Sci. Lett. 292, 51–56.
Barnes, J. D., and Straub, S. M. (2010). Chorine Stable Isotope Variations in Izu Bonin Tephra: Implications for Serpentinite Subduction. Chem. Geol. 272, 62–74. doi:10.1016/j.chemgeo.2010.02.005
Berner, R. A. (1989). Biogeochemical Cycles of Carbon and Sulfur and Their Effect on Atmospheric Oxygen over Phanerozoic Time. Glob. Planet. Change 1, 97–122. doi:10.1016/0921-8181(89)90018-0
Berner, R. A., and Caldeira, K. (1997). The Need for Mass Balance and Feedback in the Geochemical Carbon Cycle. Geol 25, 955–956. doi:10.1130/0091-7613(1997)025<0955:tnfmba>2.3.co;2
Berner, R. A. (1994). GEOCARB II; a Revised Model of Atmospheric CO 2 over Phanerozoic Time. Am. J. Sci. 294, 56–91. doi:10.2475/ajs.294.1.56
Berner, R. A. (2005). The Carbon and Sulfur Cycles and Atmospheric Oxygen from Middle Permian to Middle Triassic. Geochimica Cosmochimica Acta 69, 3211–3217. doi:10.1016/j.gca.2005.03.021
Black, B. A., Neely, R. R., and Manga, M. (2015). Campanian Ignimbrite Volcanism, Climate, and the Final Decline of the Neanderthals. Geology 43, 411–414. doi:10.1130/g36514.1
Bryant, C. J., Arculus, R. J., and Eggins, S. M. (2003). The Geochemical Evolution of the Izu-Bonin Arc System: A Perspective from Tephras Recovered by Deep-Sea Drilling. Geochem. Geophys. Geosystems 4. doi:10.1029/2002gc000427
Busby, C. J., Tamura, Y., Blum, P., Guèrin, G., Andrews, G. D. M., Barker, A. K., et al. (2017). The Missing Half of the Subduction Factory: Shipboard Results from the Izu Rear Arc, IODP Expedition 350. Int. Geol. Rev. 59, 1677–1708. doi:10.1080/00206814.2017.1292469
Calvert, A. J., Klemperer, S. L., Takahashi, N., and Kerr, B. C. (2008). Three-dimensional Crustal Structure of the Mariana Island Arc from Seismic Tomography. J. Geophys. Res. 113, B01406. doi:10.1029/2007jb004939
Cao, W., Lee, C.-T. A., and Lackey, J. (2017). Episodic Nature of Continental Arc Activity since 750 Ma: A Global Compilation. Earth Planet. Sci. Lett. 461, 85–95. doi:10.1016/j.epsl.2016.12.044
Cartigny, P., Pineau, F., Aubaud, C., and Javoy, M. (2008). Towards a Consistent Mantle Carbon Flux Estimate: Insights from Volatile Systematics (H2O/Ce, δD, CO2/Nb) in the North Atlantic Mantle (14° N and 34° N). Earth Planet. Sci. Lett. 265, 672–685. doi:10.1016/j.epsl.2007.11.011
Caves Rugenstein, J. K., Ibarra, D. E., and von Blanckenburg, F. (2019). Neogene Cooling Driven by Land Surface Reactivity rather Than Increased Weathering Fluxes. Nature 571, 99–102. doi:10.1038/s41586-019-1332-y
Dasgupta, R., and Hirschmann, M. M. (2010). The Deep Carbon Cycle and Melting in Earth's Interior. Earth Planet. Sci. Lett. 298, 1–13. doi:10.1016/j.epsl.2010.06.039
DeBari, S. M., Heywood, L. J., and Gill, J. B., 2020. Izu Bonin across Arc Geochemical Dataset between Latitudes 32°40' to 30°20', IVersion 1.0., in: (I. E. D. A. IEDA) (Ed.), doi:10.1594/IEDA/111493
Dimalanta, C., Taira, A., Yumul, G. P., Tokuyama, H., and Mochizuki, K. (2002). New Rates of Western Pacific Island Arc Magmatism from Seismic and Gravity Data. Earth Planet. Sci. Lett. 202, 105–115. doi:10.1016/s0012-821x(02)00761-6
Dutkiewicz, A., Müller, R. D., Cannon, J., Vaughan, S., and Zahirovic, S. (2018). Sequestration and Subduction of Deep-Sea Carbonate in the Global Ocean since the Early Cretaceous. Geology 47, 91–94. doi:10.1130/g45424.1
Eguchi, J., Seales, J., and Dasgupta, R. (2020). Great Oxidation and Lomagundi Events Linked by Deep Cycling and Enhanced Degassing of Carbon. Nat. Geosci. 13, 71–76. doi:10.1038/s41561-019-0492-6
Elliott, T. R. (2003). “Tracers of the Slab,” in Inside the Subduction Factory. Editor J. Eiler (Washington, DC: Geophysical Monograph Series), 23–45. doi:10.1029/138gm03
Gaillardet, J., Dupré, B., Louvat, P., and Allègre, C. J. (1999). Global Silicate Weathering and CO2 Consumption Rates Deduced from the Chemistry of Large Rivers. Chem. Geol. 159, 3–30. doi:10.1016/s0009-2541(99)00031-5
Gerya, T. V., Connolly, J. A. D., and Yuen, D. A. (2008). Why Is Terrestrial Subduction One-Sided? Geol 36, 43–46. doi:10.1130/g24060a.1
Gill, J. B. (1987). Early Geochemical Evolution of an Oceanic Island Arc and Backarc: Fiji and the South Fiji Basin. J. Geol. 95, 589–615. doi:10.1086/629158
Gill, J. B., Hiscott, R. N., and Vidal, P. (1994). Turbidite Geochemistry and Evolution of the Izu-Bonin Arc and Continents. Lithos 33, 135–168. doi:10.1016/0024-4937(94)90058-2
Grove, T. L., Chatterjee, N., Parman, S. W., and Medard, E. (2006). The Influence of H2O on Mantle Wedge Melting. Earth Planet. Sci. Lett. 249, 74–89. doi:10.1016/j.epsl.2006.06.043
Heywood, L. J., DeBari, S. M., Gill, J. B., Straub, S. M., Schindlbeck-Belo, J. C., Escobar-Burciaga, R. D., et al. (2020). Across-Arc Diversity in Rhyolites from an Intra-oceanic Arc: Evidence from IODP Site U1437, Izu-Bonin Rear Arc, and Surrounding Area. Geochem. Geophys. Geosystems 21, e2019GC008353. doi:10.1029/2019gc008353
Hilton, D. R., Fischer, T. P., and Marty, B. (2002). Noble Gases and Volatile Recycling at Subduction Zones. Rev. Mineralogy Geochem. 47, 319–370. doi:10.2138/rmg.2002.47.9
Hiscott, R. N., and Gill, J. B. (1992). “Major and Trace Element Geochemistry of Oligocene to Quaternary Volcaniclastic Sands and Sandstones from the Izu–Bonin Arc,” in Proceedings of the Ocean Drilling Program, Scientific Results. Editors B. Taylor, and K. Fujioka (College Station, Texas: Government Printing Office), 126, 467–486.
Hochstaedter, A. G., Gill, J. B., Taylor, B., Ishizuka, O., Yuasa, M., and Monta, S. (2000). Across-arc Geochemical Trends in the Izu-Bonin Arc: Constraints on Source Composition and Mantle Melting. J. Geophys. Res. 105, 495–512. doi:10.1029/1999jb900125
Hochstaedter, A. G., Gill, J. B., Peters, R., Broughton, P., Holden, P., and Taylor, B. (2001). Across-arc Geochemical Trends in the Izu-Bonin Arc: Contributions from the Subducting Slab. Geochem. Geophys. Geosystems 2, 1019. 10.1029/2000GC000105.
Holbrook, W. S., Lizarralde, D., McGeary, S., Bangs, N., and Diebold, J. (1999). Structure and Composition of the Aleutian Island Arc and Implications for Continental Crustal Growth. Geology 27, 31–34.
Ishizuka, O., Kimura, J.-I., Li, Y. B., Stern, R. J., Reagan, M. K., Taylor, R. N., et al. (2006). Early Stages in the Evolution of Izu-Bonin Arc Volcanism: New Age, Chemical, and Isotopic Constraints. Earth Planet. Sci. Lett. 250, 385–401. doi:10.1016/j.epsl.2006.08.007
Ishizuka, O., Tani, K., Reagan, M. K., Kanayama, K., Umino, S., Harigane, Y., et al. (2011a). The Timescales of Subduction Initiation and Subsequent Evolution of an Oceanic Island Arc. Earth Planet. Sci. Lett. 306, 229–240. doi:10.1016/j.epsl.2011.04.006
Ishizuka, O., Taylor, R. N., Umino, S., and Kanayama, K. (2020). Geochemical Evolution of Arc and Slab Following Subduction Initiation: a Record from the Bonin Islands, Japan. J. Petrology 61. doi:10.1093/petrology/egaa050
Ishizuka, O., Taylor, R. N., Yuasa, M., and Ohara, Y. (2011b). Making and Breaking an Island Arc: A New Perspective from the Oligocene Kyushu‐Palau Arc, Philippine Sea. Geochem. Geophys. Geosystems 12. doi:10.1029/2010gc003440
Ishizuka, O., Uto, K., Yuasa, M., and Hochstaedter, A. G. (2003). Volcanism in the Earliest Stage of Back-Arc Rifting in the Izu-Bonin Arc Revealed by Laser-Heating 40Ar/39Ar Dating. J. Volcanol. Geotherm. Res. 120, 71–85. doi:10.1016/s0377-0273(02)00365-7
Jicha, B. R., and Jagoutz, O. (2015). Magma Production Rates for Intraoceanic Arcs. Elements 11, 105–111. doi:10.2113/gselements.11.2.105
Johnson, M. C., and Plank, T. (1999). Dehydration and Melting Experiments Constrain the Fate of Subducted Sediments. Geochem. Geophys. Geosystems 1, 1007. 10.1029/1999GC000014.
Johnston, F. K. B., Turchyn, A. V., and Edmonds, M. (2011). Decarbonation Efficiency in Subduction Zones: Implications for Warm Cretaceous Climates. Earth Planet. Sci. Lett. 303, 143–152.
Kanayama, K., Umino, S., and Ishizuka, O. (2012). Eocene Volcanism during the Incipient Stage of Izu-Ogasawara Arc: Geology and Petrology of the Mukojima Island Group, the Ogasawara Islands. Isl. Arc 21, 288–316. doi:10.1111/iar.12000
Kanayama, K., Umino, S., and Ishizuka, O. (2014). Shallow Submarine Volcano Group in the Early Stage of Island Arc Development: Geology and Petrology of Small Islands South off Hahajima Main Island, the Ogasawara Islands. J. Asian Earth Sci. 85, 1–25. doi:10.1016/j.jseaes.2014.01.012
Kelemen, P. B., and Manning, C. E. (2015). Reevaluating Carbon Fluxes in Subduction Zones, what Goes Down, Mostly Comes up. Proc. Natl. Acad. Sci. U. S. A. 112, E3997–E4006. doi:10.1073/pnas.1507889112
Kelley, K. A., Plank, T., Grove, T. L., Stolper, E. M., Newman, S., and Hauri, E. H. (2006). Mantle Melting as a Function of Water Content beneath Back-Arc Basins. J. Geophys. Res. 111, B09208. doi:10.1029/2005jb003732
Kelley, K. A., Plank, T., Newman, S., Stolper, E. M., Grove, T. L., Parman, S. W., et al. (2010). Mantle Melting as a Function of Water Content beneath the Mariana Arc. J. petrology 51, 1711–1738. doi:10.1093/petrology/egq036
Kerrick, D. M., and Connolly, J. A. D. (2001). Metamorphic Devolatilization of Subducted Marine Sediments and the Transport of Volatiles into the Earth's Mantle. Nature 411, 293–296. doi:10.1038/35077056
Kerrick, D. M. (2001). Present and Past Nonanthropogenic CO2degassing from the Solid Earth. Rev. Geophys. 39, 565–585. doi:10.1029/2001rg000105
Klein, E. M., and Langmuir, C. H. (1987). Global Correlations of Ocean Ridge Basalt Chemistry with Axial Depth and Crustal Thickness. J. Geophys. Res. 92, 8089–8115. doi:10.1029/jb092ib08p08089
Kodaira, S., Noguchi, N., Takahashi, N., Ishizuka, O., and Kaneda, Y. (2010). Evolution from Fore-Arc Oceanic Crust to Island Arc Crust: A Seismic Study along the Izu-Bonin Fore Arc. J. Geophys. Res. Solid Earth 115. doi:10.1029/2009jb006968
Kogiso, T., Tatsumi, Y., and Nakano, S. (1997). Trace Element Transport during Dehydration Processes in the Subducted Oceanic Crust: 1. Experiments and Implications for the Origin of Ocean Island Basalts. Earth Planet. Sci. Lett. 148, 193–205. doi:10.1016/s0012-821x(97)00018-6
Kutterolf, S., Schindlbeck, J. C., Robertson, A. H. F., Avery, A., Baxter, A. T., Petronotis, K., et al. (2018). Tephrostratigraphy and Provenance from IODP Expedition 352, Izu-Bonin Arc: Tracing Tephra Sources and Volumes from the Oligocene to Recent. Geochem. Geophys. Geosyst. 19, 150–174. doi:10.1002/2017gc007100
Lallemand, S., and Arcay, D. (2021). Subduction Initiation from the Earliest Stages to Self-Sustained Subduction: Insights from the Analysis of 70 Cenozoic Sites. Earth-Science Rev. 221, 103779. doi:10.1016/j.earscirev.2021.103779
Lee, C.-T. A., Shen, B., Slotnick, B. S., Liao, K., Dickens, G. R., Yokoyama, Y., et al. (2013). Continental Arc-Island Arc Fluctuations, Growth of Crustal Carbonates, and Long-Term Climate Change. Geosphere 9, 21–36. doi:10.1130/ges00822.1
Lee, C.-T. A., Thurner, S., Paterson, S., and Cao, W. (2015). The Rise and Fall of Continental Arcs: Interplays between Magmatism, Uplift, Weathering, and Climate. Earth Planet. Sci. Lett. 425, 105–119. doi:10.1016/j.epsl.2015.05.045
Lee, C.-T. A., Yeung, L. Y., McKenzie, N. R., Yokoyama, Y., Ozaki, K., and Lenardic, A. (2016). Two-step Rise of Atmospheric Oxygen Linked to the Growth of Continents. Nat. Geosci. 9, 417–424. doi:10.1038/ngeo2707
Li, G., and Elderfield, H. (2013). Evolution of Carbon Cycle over the Past 100 Million Years. Geochimica Cosmochimica Acta 103, 11–25. doi:10.1016/j.gca.2012.10.014
Magni, V. (2019). The Effects of Back-Arc Spreading on Arc Magmatism. Earth Planet. Sci. Lett. 519, 141–151. doi:10.1016/j.epsl.2019.05.009
Marty, B., and Tolstikhin, I. N. (1998). CO2 Fluxes from Mid-ocean Ridges, Arcs and Plumes. Chem. Geol. 145, 233–248. doi:10.1016/s0009-2541(97)00145-9
Mason, E., Edmonds, M., and Turchyn, A. V. (2017). Remobilization of Crustal Carbon May Dominate Volcanic Arc Emissions. Science 357, 290–294. doi:10.1126/science.aan5049
Matthews, K. J., Maloney, K. T., Zahirovic, S., Williams, S. E., Seton, M., and Müller, R. D. (2016). Global Plate Boundary Evolution and Kinematics since the Late Paleozoic. Glob. Planet. Change 146, 226–250. doi:10.1016/j.gloplacha.2016.10.002
McKenzie, N. R., Horton, B. K., Loomis, S. E., Stockli, D. F., Planavsky, N. J., and Lee, C.-T. A. (2016). Continental Arc Volcanism as the Principal Driver of Icehouse-Greenhouse Variability. Science 352, 444–447. doi:10.1126/science.aad5787
Miller, M. S., Gorbatov, A., and Kennett, B. L. N. (2006). Three-dimensional Visualization of a Near-Vertical Slab Tear beneath the Southern Mariana Arc. Geochem. Geophys. Geosyst. 7, Q06012. doi:10.1029/2005gc001110
Müller, D., Seton, M., Zahirovic, S., Williams, S., Matthews, S., Wright, N., et al. (2016). Ocean Basin Evolution and Global-Scale Plate Reorganization Events since Pangea Breakup. Annu. Rev. Earth Planet. Sci. 44, 107–138.
Müller, R. D., Sdrolias, M., Gaina, C., and Roest, W. R. (2008). Age, Spreading Rates, and Spreading Asymmetry of the World's Ocean Crust. Geochem. Geophys. Geosystems 9.
Nikolaeva, K. M., Gerya, T. V., and Connolly, J. A. D. (2008). Numerical Modelling of Crustal Growth in Intraoceanic Volcanic Arcs. Phys. Earth Planet. Interiors 171, 336–356. doi:10.1016/j.pepi.2008.06.026
Okino, K., Shimakawa, Y., and Nagaoka, S. (1994). Evolution of the Shikoku Basin. J. Geomagn. geoelec 46, 463–479. doi:10.5636/jgg.46.463
Peccerillo, A., and Taylor, S. R. (1976). Geochemistry of Eocene Calc-Alkaline Volcanic Rocks from the Kastamonu Area, Northern Turkey. Contr. Mineral. Petrol. 58, 63–81. doi:10.1007/bf00384745
Peacock, S. A. (1990). Fluid Processes in Subduction Zones. Science 248, 329–337. doi:10.1126/science.248.4953.329
Pearce, J. A., and Robinson, P. T. (2010). The Troodos Ophiolitic Complex Probably Formed in a Subduction Initiation, Slab Edge Setting. Gondwana Res. 18, 60–81. doi:10.1016/j.gr.2009.12.003
Pearce, J. A., Stern, R. J., Bloomer, S. H., and Fryer, P. (2005). Geochemical Mapping of the Mariana Arc-Basin System : Implications for the Nature and Distribution of Subduction Components. Geochem. Geophys. Geosystems 6, Q07006. doi:10.1029/2004gc000895
Plank, T., Kelley, K. A., Zimmer, M. M., Hauri, E. H., and Wallace, P. J. (2013). Why Do Mafic Arc Magmas Contain ∼4wt% Water on Average? Earth Planet. Sci. Lett. 364, 168–179.
Pogge von Strandmann, P. A. E., Jenkyns, H. C., and Woodfine, R. G. (2013). Lithium Isotope Evidence for Enhanced Weathering during Oceanic Anoxic Event 2. Nat. Geosci. 6, 668–672. doi:10.1038/ngeo1875
Raymo, M. E., and Ruddiman, W. F. (1992). Tectonic Forcing of Late Cenozoic Climate. Nature 359, 117–122. doi:10.1038/359117a0
Reagan, M. K., Heaton, D. E., Schmitz, M. D., Pearce, J. A., Shervais, J. W., and Koppers, A. A. P. (2019). Forearc Ages Reveal Extensive Short-Lived and Rapid Seafloor Spreading Following Subduction Initiation. Earth Planet. Sci. Lett. 506, 520–529. doi:10.1016/j.epsl.2018.11.020
Reagan, M. K., Ishizuka, O., Stern, R. J., Kelley, K. A., Ohara, Y., Blichert-Toft, J., et al. (2010). Fore-arc Basalts and Subduction Initiation in the Izu-Bonin-Mariana System. Geochem. Geophys. Geosyst. 11, a–n. doi:10.1029/2009GC002871
Reagan, M. K., Pearce, J. A., Petronotis, K., Almeev, R. R., Avery, A. J., Carvallo, C., et al. (2017). Subduction Initiation and Ophiolite Crust: New Insights from IODP Drilling. Int. Geol. Rev. 59, 1439–1450. doi:10.1080/00206814.2016.1276482
Ribeiro, J. M., Ishizuka, O., Lee, C. T. A., and Girard, G. (2020). Evolution and Maturation of the Nascent Mariana Arc. Earth Planet. Sci. Lett. 530, 115912. doi:10.1016/j.epsl.2019.115912
Ribeiro, J. M., Stern, R. J., Kelley, K., Shaw, A., Martinez, F., and Ohara, Y. (2015). Composition of the Slab-Derived Fluids Released beneath the Mariana Forearc: Evidence for Shallow Dehydration of the Subducting Plate. Earth Planet. Sci. Lett. 418, 136–148. doi:10.1016/j.epsl.2015.02.018
Ruscitto, D. M., Wallace, P. J., Cooper, L. B., and Plank, T. (2012). Global Variations in H2O/Ce: 2. Relationships to Arc Magma Geochemistry and Volatile Fluxes. Geochem. Geophys. Geosystems 13, Q03025. doi:10.1029/2011gc003887
Saito, S., and Tani, K. (2017). Transformation of Juvenile Izu-Bonin-Mariana Oceanic Arc into Mature Continental Crust: An Example from the Neogene Izu Collision Zone Granitoid Plutons, Central Japan. Lithos 277, 228–240. doi:10.1016/j.lithos.2016.07.035
Sano, Y., and Marty, B. (1995). Origin of Carbon in Fumarolic Gas from Island Arcs. Chem. Geol. 119, 265–274. doi:10.1016/0009-2541(94)00097-r
Schindlbeck, J. C., Jegen, M., Freundt, A., Kutterolf, S., Straub, S. M., Mleneck-Vautravers, M. J., et al. (2018a). 100- Kyr Cyclicity in Volcanic Ash Emplacement: Evidence from a 1.1 Myr Tephra Record from the NW Pacific. Sci. Rep. 8, 4440. doi:10.1038/s41598-018-22595-0
Schindlbeck, J. C., Kutterolf, S., Straub, S. M., Andrews, G. D. M., Wang, K.-L., and Mleneck-Vautravers, M. J. (2018b). One Million Years Tephra Record at IODP Sites U1436 and U1437: Insights into Explosive Volcanism from the Japan and Izu Arcs. Isl. Arc 27, e12244. doi:10.1111/iar.12244
Schmidt, M., and Poli, S. (1998). Experimentally Based Water Budgets for Dehydrating Slabs and Consequences for Arc Magma Generation. Earth Planet. Sci. Lett. 163, 361–379. doi:10.1016/s0012-821x(98)00142-3
Shervais, J. W., Reagan, M., Haugen, E., Almeev, R. R., Pearce, J. A., Prytulak, J., et al. (2019). Magmatic Response to Subduction Initiation: Part 1. Fore‐arc Basalts of the Izu‐Bonin Arc from IODP Expedition 352. Geochem. Geophys. Geosyst. 20, 314–338. doi:10.1029/2018gc007731
Shervais, J. W., Reagan, M. K., Godard, M., Prytulak, J., Ryan, J. G., Pearce, J. A., et al. (2021). Magmatic Response to Subduction Initiation, Part II: Boninites and Related Rocks of the Izu-Bonin Arc from IOPD Expedition 352. Geochem. Geophys. Geosystems 22, e2020GC009093. doi:10.1029/2020gc009093
Skora, S., Blundy, J. D., Brooker, R. A., Green, E. C. R., de Hoog, J. C. M., and Connolly, J. A. D. (2015). Hydrous Phase Relations and Trace Element Partitioning Behaviour in Calcareous Sediments at Subduction-Zone Conditions. J. Petrology 56, 953–980. doi:10.1093/petrology/egv024
Sleep, N. H., and Zahnle, K. (2001). Carbon Dioxide Cycling and Implications for Climate on Ancient Earth. J. Geophys. Res. 106, 1373–1399. doi:10.1029/2000je001247
Soreghan, G. S., Soreghan, M. J., and Heavens, N. G. (2019). Explosive Volcanism as a Key Driver of the Late Paleozoic Ice Age. Geology 47, 600–604. doi:10.1130/g46349.1
Stern, R. J., and Bloomer, S. H. (1992). Subduction Zone Infancy: Examples from the Eocene Izu-Bonin-Mariana and Jurassic California Arcs. Geol. Soc. Am. Bull. 104, 1621–1636. doi:10.1130/0016-7606(1992)104<1621:szieft>2.3.co;2
Stern, R. J., Fouch, M., and Klemperer, S. L. (2003). “An Overview of the Izu-Bonin-Mariana Subduction Factory,” in Inside the Subduction Factory. Editors J. Eiler, and M. Hirschmann (Washington, D.C.: Geophysical Monograph Series), 175–222. doi:10.1029/138gm10
Straub, S. M., Goldstein, S. L., Class, C., Schmidt, A., and Gomez-Tuena, A. (2010). Slab and Mantle Controls on the Sr-Nd-Pb-Hf Isotope Evolution of the Post 42 Ma Izu-Bonin Volcanic Arc. J. Petrology 51, 993–1026. doi:10.1093/petrology/egq009
Straub, S. M., Gómez-Tuena, A., and Vannucchi, P. (2020). Subduction Erosion and Arc Volcanism. Nat. Rev. Earth Environ. 1, 574–589. doi:10.1038/s43017-020-0095-1
Straub, S. M., and Layne, G. D. (2003). Decoupling of Fluids and Fluid-Mobile Elements during Shallow Subduction: Evidence from Halogen-Rich Andesite Melt Inclusions from the Izu Arc Volcanic Front. Geochem. Geophys. Geosystems 4, 9004. doi:10.1029/2002gc000349
Straub, S. M., Layne, G. D., Schmidt, A., and Langmuir, C. H. (2004). Volcanic Glasses at the Izu Arc Volcanic Front: New Perspectives on Fluid and Sediment Melt Recycling in Subduction Zones. Geochem. Geophys. Geosystems 5, Q01007. doi:10.1029/2002gc000408
Straub, S. M., and Layne, G. D. (2002). The Systematics of Boron Isotopes in Izu Arc Front Volcanic Rocks. Earth Planet. Sci. Lett. 198, 25–39. doi:10.1016/s0012-821x(02)00517-4
Straub, S. M. (2003). The Evolution of the Izu Bonin - Mariana Volcanic Arcs (NW Pacific) in Terms of Major Element Chemistry. Geochem. Geophys. Geosystems 4. doi:10.1029/2002gc000357
Straub, S. M., Woodhead, J. D., and Arculus, R. J. (2015). Temporal Evolution of the Mariana Arc: Mantle Wedge and Subducted Slab Controls Revealed with a Tephra Perspective. J. Petrology 56, 409–439. doi:10.1093/petrology/egv005
Sun, S. S., and McDonough, W. F. (1989). “Chemical and Isotopic Systematics of Oceanic Basalts: Implications for Mantle Composition and Processes,” in Magmatism in the Ocean Basins. Editors A. D. Saunders, and M. J. Norry (London: Geological Society of London, Special publications), 42, 313–345. doi:10.1144/gsl.sp.1989.042.01.19Geol. Soc. Lond. Spec. Publ.
Takahashi, N., Kodaira, S., Klemperer, S. L., Tatsumi, Y., Kaneda, Y., and Suyehiro, K. (2007). Crustal Structure and Evolution of the Mariana Intra-oceanic Island Arc. Geol 35, 203–206. doi:10.1130/G23212A.23211
Takahashi, N., Kodaira, S., Tatsumi, Y., Yamashita, M., Sato, T., Kaiho, Y., et al. (2009). Structural Variations of Arc Crusts and Rifted Margins in the Southern Izu-Ogasawara Arc-Back Arc System. Geochem. Geophys. Geosyst. 10, a–n. doi:10.1029/2008GC002146
Tatsumi, Y. (1986). Formation of the Volcanic Front in Subduction Zones. Geophys. Res. Lett. 13, 717–720. doi:10.1029/gl013i008p00717
Taylor, B. (1992). “Rifting and the Volcanic-Tectonic Evolution of the Izu-Bonin-Mariana Arc,” in Proceedings of the Ocean Drilling Program, Scientific Results. Editors B. Taylor, K. Fujioka, and S. S. Party, 627–651. doi:10.2973/odp.proc.sr.126.163.1992
Todd, E., Gill, J. B., and Pearce, J. A. (2012). A Variably Enriched Mantle Wedge and Contrasting Melt Types during Arc Stages Following Subduction Initiation in Fiji and Tonga, Southwest Pacific. Earth Planet. Sci. Lett. 335-336, 180–194. doi:10.1016/j.epsl.2012.05.006
Tollstrup, D. L., Gill, J. B., Kent, A., Prinkey, D., Williams, R., Tamura, Y., et al. (2010). Across-arc Geochemical Trends in the Izu-Bonin Arc: Contributions from the Subducting Slab, Revisited. Geochem. Geophys. Geosyst. 11, a–n. doi:10.1029/2009gc002847
Tucker, J. M., Hauri, E. H., Pietruszka, A. J., Garcia, M. O., Marske, J. P., and Trusdell, F. A. (2019). A High Carbon Content of the Hawaiian Mantle from Olivine-Hosted Melt Inclusions. Geochimica Cosmochimica Acta 254, 156–172. doi:10.1016/j.gca.2019.04.001
Turner, S. J., Langmuir, C. H., Katz, R. F., Dungan, M. A., and Escrig, S. (2016). Parental Arc Magma Compositions Dominantly Controlled by Mantle-Wedge Thermal Structure. Nat. Geosci. 9, 772–776. doi:10.1038/ngeo2788
Turner, S. J., and Langmuir, C. H. (2015a). The Global Chemical Systematics of Arc Front Stratovolcanoes: Evaluating the Role of Crustal Processes. Earth Planet. Sci. Lett. 422, 182–193. doi:10.1016/j.epsl.2015.03.056
Turner, S. J., and Langmuir, C. H. (2015b). What Processes Control the Chemical Compositions of Arc Front Stratovolcanoes? Geochem. Geophys. Geosyst. 16, 1865–1893. doi:10.1002/2014gc005633
van der Hilst, R., and Seno, T. (1993). Effects of Relative Plate Motion on the Deep Structure and Penetration Depth of Slabs below the Izu-Bonin and Mariana Island Arcs. Earth Planet. Sci. Lett. 120, 395–407. doi:10.1016/0012-821x(93)90253-6
Vitale Brovarone, A., Chu, X., Martin, L., Ague, J. J., Monié, P., Groppo, C., et al. (2018). Intra-slab COH Fluid Fluxes Evidenced by Fluid-Mediated Decarbonation of Lawsonite Eclogite-Facies Altered Oceanic Metabasalts. Lithos 304-307, 211–229. doi:10.1016/j.lithos.2018.01.028
von Huene, R., and Scholl, D. W. (1991). Observations at Convergent Margins Concerning Sediment Subduction, Subduction Erosion, and the Growth of Continental Crust. Rev. Geophys. 29, 279–316. doi:10.1029/91rg00969
von Strandmann, P. A. E., Burton, K. W., James, R. H., van Calsteren, P., and Gislason, S. R. (2010). Assessing the Role of Climate on Uranium and Lithium Isotope Behaviour in Rivers Draining a Basaltic Terrain. Chem. Geol. 270, 227–239. doi:10.1016/j.chemgeo.2009.12.002
Wallace, L. M., McCaffrey, R., Beavan, J., and Ellis, S. (2005). Rapid Microplate Rotations and Backarc Rifting at the Transition between Collision and Subduction. Geol 33, 857–860. doi:10.1130/g21834.1
Wong, K., Mason, E., Brune, S., East, M., Edmonds, M., and Zahirovic, S. (2019). Deep Carbon Cycling over the Past 200 Million Years: A Review of Fluxes in Different Tectonic Settings. Front. Earth Sci. 7. doi:10.3389/feart.2019.00263
Wu, J., Suppe, J., Lu, R., and Kanda, R. (2016). Philippine Sea and East Asian Plate Tectonics since 52 Ma Constrained by New Subducted Slab Reconstruction Methods. J. Geophys. Res. Solid Earth 121, 4670–4741. doi:10.1002/2016jb012923
Wu, J. T.-J., and Wu, J. (2019). Izanagi-Pacific Ridge Subduction Revealed by a 56 to 46 Ma Magmatic Gap along the Northeast Asian Margin. Geology 47, 953–957. doi:10.1130/g46778.1
Keywords: Izu convergent margin, arc magmatic evolution, climate changes, Paleo-climate, long-term steady-state, arc magmatic history
Citation: Ribeiro JM, Ishizuka O, Pogge von Strandmann PAE, Tamura Y and Xu Y-G (2022) Temporal Evolution of Island Arc Magmatism and Its Influence on Long-Term Climate: Insights From the Izu Intra-Oceanic Arc. Front. Earth Sci. 10:810597. doi: 10.3389/feart.2022.810597
Received: 07 November 2021; Accepted: 22 June 2022;
Published: 26 July 2022.
Edited by:
Jaime Barnes, University of Texas at Austin, United StatesReviewed by:
Wenrong Cao, University of Nevada, Reno, United StatesCopyright © 2022 Ribeiro, Ishizuka, Pogge von Strandmann, Tamura and Xu. This is an open-access article distributed under the terms of the Creative Commons Attribution License (CC BY). The use, distribution or reproduction in other forums is permitted, provided the original author(s) and the copyright owner(s) are credited and that the original publication in this journal is cited, in accordance with accepted academic practice. No use, distribution or reproduction is permitted which does not comply with these terms.
*Correspondence: Julia M. Ribeiro, anVsaWFyaWJlaXJvQGdpZy5hYy5jbg==
Disclaimer: All claims expressed in this article are solely those of the authors and do not necessarily represent those of their affiliated organizations, or those of the publisher, the editors and the reviewers. Any product that may be evaluated in this article or claim that may be made by its manufacturer is not guaranteed or endorsed by the publisher.
Research integrity at Frontiers
Learn more about the work of our research integrity team to safeguard the quality of each article we publish.