- 1Extreme Events Research Group, Max Planck Institutes for Geoanthropology, Chemical Ecology, and Biogeochemistry, Jena, Germany
- 2Department of Archaeology, Max Planck Institute for the Science of Human History, Jena, Germany
- 3Institute of Prehistoric Archaeology, University of Cologne, Cologne, Germany
- 4Department of Classics and Archaeology, University of Malta, Msida, Malta
The term “extreme event” is commonly used to describe high-impact, unanticipated natural events, like floods, tsunamis, earthquakes, and volcanic eruptions. It first appeared in the scientific literature in the 1950s and has since spread to disciplines as diverse as economics, psychology, medicine, and engineering. The term is increasingly being applied to the study of historical, prehistorical, and deep-time events across a broad range of scales, and it is widely acknowledged that such events have had profound impacts on the Earth’s biodiversity and cultures. Understandably, then, how people think about, define, and study extreme events varies considerably. With extreme events expected to become more frequent, longer lasting, and more intense in the coming decades as a result of global warming, the differing extreme event definitions—both across and within disciplines—is likely to lead to confusion among researchers and pose significant challenges for predicting and preparing for extreme events and their impacts on natural and social systems. With this in mind, we conducted a systematic quantitative review of 200 randomly selected, peer-reviewed “extreme event” research papers (sourced from Web of Science, accessed January 2020) from the biological, societal, and earth sciences literature with the aim of quantifying several pertinent features of the research sample. On the one hand, our analysis found a great deal of variability among extreme event papers with respect to research interests, themes, concepts, and definitions. On the other hand, we found a number of key similarities in how researchers think about and study extreme events. One similarity we encountered was that researchers tend to view extreme events within a particular temporal context and quite often in terms of rates of change. Another similarity we encountered was that researchers often think of and study extreme events in terms of risks, vulnerabilities, and impacts. The similarities identified here may be useful in developing a common and comprehensive definition of what constitutes an extreme event, and should allow for more comparative research into extreme events at all spatio-temporal scales which, we predict, will provide important new insights into the nature of extreme events.
Introduction
Extreme events are expected to become more frequent, longer lasting, and more intense through the 21st century as a result of human-induced climate change (IPCC 2021). These changes will almost certainly have devastating societal, environmental, and economic consequences. Already we are witnessing an uptick in the number of extreme heat and precipitation events as a result of climate change (IPCC 2021). Take for instance the 2003 European heat wave. This record-breaking event put a tremendous strain on health, resource, and energy systems. The loss to the agricultural sector was estimated at around €13 billion (IPCC 2021) and the event claimed upwards of 30,000 lives (Kosatsky, 2005). Data suggest that human-induced climate change contributed significantly to the 2003 European summer warming (Stott et al., 2004), and climate model simulations suggest that late 21st century summers may see average temperatures that resemble the extreme temperatures of this devastating heat wave (Beniston, 2004). These expectations have put extreme events and extreme event attribution at the center of a growing body of scientific research and spotlighted them with respect to planning and policy (Kraas, 2008; IPCC 2021).
Human populations are also experiencing changes in their risk, vulnerability, and exposure to extreme events due to non-climatic factors (for definitions of key terms see Table 1). For instance, urbanization and population growth concentrate people and activities into small areas, increasing vulnerability and exposure of people and populations to extreme events (Kraas, 2008; IPCC 2021). Moreover, human activities such as “soil sealing” and extensive cover of urban areas with impermeable materials increases flood risks (e.g., Pistocchi et al., 2015). There are also other societal changes such as rapidly aging populations in developed nations (e.g., Li et al., 2016). Variables such as these, all of which may be overlapping and compounding, make clarifying the meaning and character of extreme events a pertinent research topic (e.g., McPhillips et al., 2018; Broska et al., 2020).
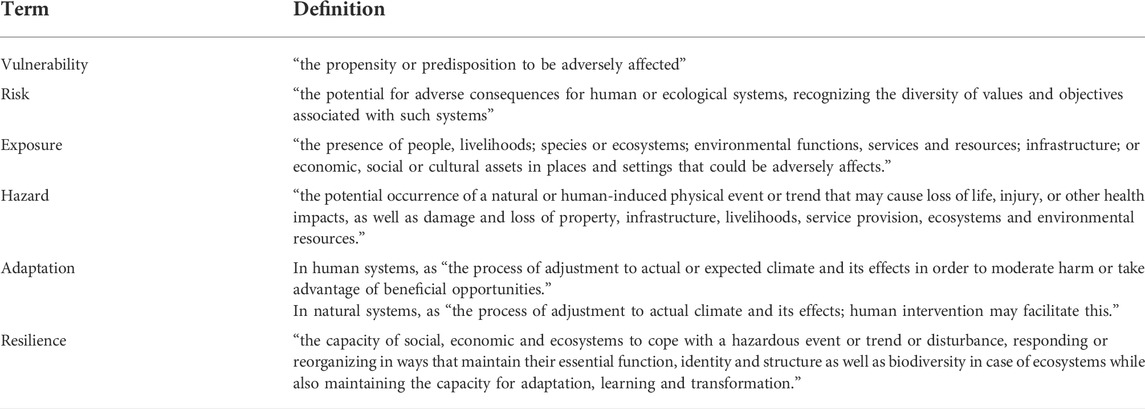
TABLE 1. Some definitions of terms commonly used in extreme events literature as provided in the IPCC (2022).
Analyzing extreme events and planning for them, however, is challenging because events considered “extreme” can be decidedly different (Albeverio et al., 2006). Environmental hazards, for instance, can include extreme events like volcanic eruptions, earthquakes, asteroid impacts, heat waves, floods, and firestorms. Economic events can also be considered extreme, like recession, depression, and investment bubbles (e.g., Longin, 2000; Pagan and Sossounov, 2003). Events of a more sociological character, like crime waves, riots, demonstrations, and mass migration can be extreme, and so can political events like regime changes, state failure, acts of terrorism, and warfare (e.g., Comfort, 2002; Andrade et al., 2019). Finally, medical and epidemiological events can justifiably be called extreme as well, with examples like seizures, cardiac events, historical plagues, and the ongoing COVID-19 crisis (e.g., Lehnertz, 2006; Wang and Su, 2020). The variety of events that can and have been labelled “extreme” is surprisingly vast, making it hard to see the forest for the trees.
Even among events of a kind, there can be important differences. Flooding, for example, is an event commonly labelled “extreme,” but floods can differ dramatically despite the fact that they all involve relatively large increases in the volume of water. In the summer of 2021, 15 cm of rain fell over parts of Germany and Belgium in as little as 24 h, leading to at least 196 deaths and millions of Euros in immediate damage to property and infrastructure. Compare that relatively localized extreme event with the expected scale and extent of global coastal flooding caused by sea level rise over the next century (e.g., Goddard et al., 2015; Frederikse et al., 2020). Scientists expect mean sea level to rise by as much as 2.5 m by 2100 with as many as 1.4 billion people directly affected by increasingly severe storm surges, flooding, saltwater intrusion, and coastal erosion. The economic costs could be astronomical and there will almost certainly be a grim human toll. These two extreme events are related by the involvement of climate change and flood water, but they are in many ways very different from each other in terms of causal chains, scale, time horizons, and the magnitude of costs, both economic and human.
Crucially, differences in temporal scale occur as well, and these have not been adequately discussed in the literature as far as we are aware. The timescales over which extreme events are thought to occur can sit at opposite ends of a long continuum. At one end are events occurring over seconds to minutes, like epileptic seizures within the brain (Lehnertz, 2006). At the other end, events may unfold over millennia or even millions of years, with classic examples being the “big five” mass extinction events (Raup and Sepkoski, 1982). These timescale differences highlight an understudied aspect of extreme events, namely the degree to which they are “event-like.” English speakers usually only refer to an occurrence as an “event” if it (whatever is observed) is bounded in time and of some significance (Collins English Dictionary, 2018)—events have to be temporally discrete in some way in order for the label to make much sense in contrast to a common state of affairs or general condition. They also tend to recur—floods and heatwaves, for instance, happen every year somewhere in the world. Consequently, an event considered extreme when viewed on one timescale may be humdrum when viewed on another. Take for example the greatest mass extinction event, the end-Permian Extinction. Around 252 million years ago, ∼60% of all biological families went extinct (Benton, 1995), an event sometimes called “The Great Dying.” It was extreme by any sensible standard, but it occurred over a period of roughly 200,000 years. So, while all events have to be temporally bounded in order to be an “event” (in contrast to some standing reference condition), the ones we know about tend to only appear bounded at some temporal scales while being continuous at others.
Furthermore, scientists studying extreme events do so from perspectives that differ in important ways. In a recent review, McPhillips et al. (2018, pp. 443–445) state that climate scientists are primarily concerned with “mapping, characterizing, and modeling [natural] hazards,” whereas social scientists are mostly concerned with understanding the “underlying social conditions that influence and/or are influenced by extreme events.” These different motivations and natural/physical domains of interest give rise to different conceptions of what an “event” is and what makes one “extreme.” They also lead researchers to emphasize different aspects of events and their effects. Some, for instance, emphasize “vulnerability” as a core component of extreme event research. As Brooks (2003) notes, social scientists typically think of vulnerability in terms of the “set of socio-economic factors that determine people’s ability to cope with stress or change,” whereas climate scientists tend to view vulnerability as the “likelihood of occurrence and impacts of weather and climate related events.” These differences in terms of research interest and natural/physical domains make one wonder whether scholars from these separate fields are studying specific subtypes of a more general phenomena (i.e., extreme events) or phenomena that are altogether different.
Differences regarding research perspectives have led to a variety of conceptual and operational definitions for extreme events. Again, according to McPhillips et al. (2018), a variety of concepts related to extreme events have been used in the academic literature and, importantly, that different fields of research seem to employ different terms. They found, for instance, that “disturbance” was most common in ecological research, “hazard” more common in the Earth sciences, and “disaster” was most common in the social sciences. While it is easy to see how these terms are related to “extreme events” more broadly, they are not exact synonyms. Each term emphasizes a different perspective of extreme events and implies a slightly different conceptual definition—e.g., a given event may be “extreme” to a social scientist only if it results in a “disaster.” McPhillips et al. also found distinctly different operational definitions—that is, definitions that determine how events are identified and measured. In some cases, events were identified by exceedances of absolute thresholds, while in other cases researchers determined a given event was extreme because it was considered statistically unlikely within a set of observations. The definitions identified by McPhillips’ et al. were so variable that no normative definition(s) could be identified even within a given academic discipline.
Another key difference relates to whether extreme events should be defined by their impacts (realized or projected) or not. Sarewitz and Piekle (2001), for example, define extreme events as “an occurrence that, with respect to some class of occurrences, is either notable, rare, unique, profound, or otherwise significant in terms of its impacts, effects or outcomes.” Similarly, from an ecological sciences perspective, Smith (2011, p. 656) defines extreme climatic events as “an episode or occurrence in which a statistically rare or unusual climatic period alters ecosystem structure and/or function well outside the bounds of what is considered typical or normal variability.” In both these definitions, the impact (or response) is integral to the definition of extreme event. In other words, if a system’s response falls within its natural range of variability—regardless of the natural properties of the event itself—then the system has not experienced an extreme event. In direct contrast to these definitions, McPhillips and others (2018) call for the separation of events and impacts when defining extreme events. Essentially, the authors argue that if the motivation for studying extreme events is ultimately to lessen their impacts, then it is important to distinguish between events on the one hand, and impacts on the other, so that successful management and mitigation strategies can be properly recognized and developed.
Based on these conflicting definitions, it is easy to see how an event could be classified as extreme or not depending on which definition one subscribes. Take for instance the Canterbury earthquake that struck the South Island of New Zealand in 2010. Despite having a magnitude of 7.1—and therefore being classified as “extreme” on the modified Mercalli intensity scale—this earthquake resulted in only two deaths. A definition based on the natural properties of the earthquake would describe this event as extreme, whereas a definition that incorporates the event’s impacts may not. Now compare this event to the earthquake that hit Port-au-Prince, Haiti, in the same year (see Matthewman, 2016). This earthquake was of a similar magnitude, depth, and other characteristics, but saw some three million people affected and upwards of 100,000 casualties. That is to say, these events were similar in their natural characteristics but had profoundly different impacts. A definition that includes impacts would, therefore, describe the Canterbury earthquake as being much less extreme of an event (or not an extreme event at all) than the Port-au-Prince earthquake, whereas a definition based solely on the natural characteristics of the events would describe these events as being of similar “extremeness.”
At first glance, the variability in extreme event research could be seen as a hinderance. The seemingly irreconcilable differences between research goals, scales, and definitions limit our ability to generalize and, therefore, to predict and prepare for extreme events. And yet, this variability also creates an opportunity for consilience. Here we mean “scientific consilience” whereby observations made in different fields with different tools and perspectives may all point to a single conclusion, indicating a convergence of knowledge. When knowledge convergence occurs, the differences among individual findings strengthen a given conclusion more than would a similar number of repeated, identical findings (Wilson, 1999). Consilience regarding extreme event phenomena, then, would allow us to better understand those phenomena individually and collectively. We could, in turn, better predict the occurrence of extreme events and their effects in the long-term. The costs of such events compounded by their increasing frequency and severity make it important to determine whether consilience is possible. This, in our view, is an urgent task.
With this in mind, we conducted a systematic review of 200 randomly selected scientific extreme events articles and book chapters in the biological, societal, and earth sciences as part of the special issue Extreme Events in Human Evolution: From the Pliocene to the Anthropocene. In essence, we wanted to capture extreme events literature that intersected these three disciplines, as well as provide a long-term perspective to extreme events to address the important issue of temporal scale we described above. To that end, this review had three main goals. The first was to synthesize how researchers across these disciplines defined and conceptualized extreme events. Importantly, we wanted to try and determine how “events” are being defined and what makes them “extreme” across disciplines, while at the same time avoiding imposing our own definition of these terms and the compound term “extreme event” on the literature. The second goal was to identify and scrutinize clusters in the literature based on the themes identified in our analysis. And finally, we aimed to determine whether a cohesive, multidisciplinary, multiscalar definition of extreme events could be developed and, if so, lay out a research trajectory to that end.
Materials and methods
To explore how researchers conceptualize and operationalize extreme events in biological, societal, and earth sciences, we conducted a review of academic literature using Web of Science (WoS; accessed January 2020) and following, where applicable, the Preferred Reporting Items for Systematic Reviews and Meta-analyses (PRISMA) statement (Moher et al., 2009). On beginning, we had to establish some search limits. In terms of time, we wanted to emphasize more recent research without excluding early studies. We also know from other reviews (e.g., McPhillips et al., 2018; Broska et al., 2020) that the publication of the first IPCC report in 1990 may have coincided with a marked increase in public and scholarly interest in human–environment interactions, including anthropogenic climate change and its impacts on society. So, as a compromise, we decided to limit our literature search to the three decades following 1990. Moreover, to reduce picking up articles outside of the scope of this review, we then further restricted our search to the following WoS categories: archaeology, anthropology, environmental sciences, ecology, geology, evolutionary biology, geography, and multidisciplinary studies.
In order to isolate papers focused on extreme events, we used a WoS topic query. Terms were searched for within the keywords, titles, and abstracts of papers indexed by WoS. As discussed above, different disciplines oftentimes emphasize different aspects of extreme events, sometimes explicitly using the term “extreme event,” while other times using similar words with slightly different connotations (McPhillips et al., 2018). To bridge this divide we included additional search terms. On preliminary reading of the literature, it was clear that the terms “abrupt” and “rapid” were commonly used to describe extreme climatic events in history and prehistory, and so these terms were added to capture the long-term perspective mentioned above. Terms related to people, the climate, and the environment were also added to emphasize the disciplines of interest. The final WoS search query was: TS = (human or homin*) AND TS = (climat* OR environment*) AND TS = (extreme OR rapid OR disaster* OR abrupt OR catastroph*) AND event. Asterisks acted as wildcards to capture alternative endings like “environment-al,” climat-ic, “catastroph-ic,” and “homin-in.”
The search returned 1,626 articles. Metadata for these articles were compiled into an Excel spreadsheet. The rows of the spreadsheet were then randomized and two of the authors (MS, WCC) independently analyzed the articles in the shuffled spreadsheet, one starting at the top and the other at the bottom. Articles outside the scope of the review were excluded—for example, a study on the effects of rearing methods on the post-release survival of southern sea otters mentioned the vulnerability of populations of this species to catastrophic oil spills, however, the study itself was not concerned with extreme events (Nicholson et al., 2007). Articles were analyzed until each analyst had reviewed 100 articles (for a total of 200).
The articles were analyzed with QSR International’s Citavi reference manager version 6.3.0.0 (https://www.citavi.com). This program allows text in documents to be highlighted, tagged, and coded so that themes in the literature could be identified and explored. Using these functions, we coded text in each article to answer a series of questions designed to capture how extreme events are conceptualized and operationalized by scholars (Table 2). This included manually grouping each article into disciplines, similar to McPhillips et al. (2018). As our study was not concerned with research quality, no quality checks were performed. Once all relevant articles were tagged and coded, all authors consulted the data to check for instances where related tags might be placed under “senior” labels. As an example, “ecological management,” “risk management,” and “hazard management” were placed under “management.” After tags were collated, the data was then exported using Citavi’s “Export to Microsoft Excel” function and imported into R version 3.6.3 (R Core Team, 2013). The patterns in the data were then explored in R using igraph (Csardi and Nepusz, 2006), gplots (Warnes et al., 2009), tidyverse (Wickham et al., 2019), tm4ss (Wiedemann and Niekler, 2017), and their associated packages.
To assess how sufficiently the literature had been sampled, we constructed collector’s plots (also known as species accumulation curves) for event types, data types, concepts, and themes. This approach charts the increase in some variable of interest—the number of species of beetles in a forest plot, for example—relative to sampling effort. Typically, the resulting curve rises rapidly at the start, and then more slowly in later samples (in this case articles) as increasingly rare occurrences (in this case tags) are added, before ultimately reaching a plateau (Gotelli and Colwell, 2001). The curve, therefore, reflects the diminishing returns of sampling effort and gives an idea as to how sufficiently a variable of interest has been sampled for—that is, if the curve reaches a stable plateau, it could be argued that the total sample is representative of the variable of interest.
We also assessed the through-time trend in research interest in extreme events. This required correcting for through-time increases in publication volume more generally. We therefore divided the number of articles published each year in our sample by the number of articles published each year in five of the most common journals in our dataset. Number of per year publications was sourced from Clavarites’ InCites tool, which provides data going back to 1997, and we included only those journals with data available going back to this time. While this excludes newer journals like PLOS One (active since 2006), it likely captures the general through-time trends in publication volume.
Lastly, we ran a simple clustering analysis using the heatmap.2 function (gplots) to explore co-occurring themes in the literature sample. This function first computes a distance (dissimilarity) matrix for the objects being clustered—in this case themes and articles—and then performs a hierarchical clustering analysis using the calculated distances. The output is then visualized as two dendrograms—one each for the rows and columns—and a heatmap. No specific dissimilarity cutoff value was used to define the clusters in our analysis. Instead, the heatmap was visually inspected to identify groups of papers that covered similar topics, which were in turn used to structure the discussion. And so, while the clusters are somewhat arbitrarily defined, we considered it to be an appropriate and useful approach for our purposes, and one that provided more objectivity to the analysis than more traditional reviews.
Results
The WoS search returned 1,626 articles. Of these, 124 were excluded as they did not fall within the scope of the literature review—that is, their focus was not on extreme events (for an example of such an article see Methods sections) (Supplementary Figure S1). A total of 200 articles were analyzed and the results of the systematic review are presented below. A full list of the articles can be found in Appendix 3.
Sampling adequacy
As expected, all five curves show a rapid rise at the start of the simulation as new tags were quickly added, followed by a deceleration in later articles with the addition of rarer tags (Supplementary Figure S2). The concepts curve appears to be approaching a plateau suggesting that a larger dataset would not result in significantly more concepts being added. The other three curves, however, still appear to have been increasing towards the end of the simulation. This is perhaps unsurprising given the broad scope of this review. Still, given that we focus on the most common concepts, themes, event types, and data types, it seems likely that we have adequately captured the structure of the data and that a significantly larger literature sample would show broadly the same patterns.
This is also supported by the almost exponential distribution of tags in each of these cases. For example, over half of the event types have only a single occurrence, whereas the top five occur in 29–62 articles (15–31% of articles). Similarly, around half of the concepts have three or fewer occurrences, whereas the top five concepts occur in 43–73 articles (22–37% of articles). It therefore seems unlikely that a significantly larger sample size would drastically alter the structure of the data with respect to the most common event types, data types, concepts, and themes.
Summary of the literature
It is clear from the data that interest in extreme events has been increasing in recent decades, with around one sixth of all papers in the literature sample being published in 2019 alone (Figure 1). Even when accounting for through-time increases in publication volume, the number of extreme event publications has been growing since at least the 1990’s. Among the articles analyzed, a wide range of extreme events was covered including natural hazards (e.g., earthquakes, floods), ecological events (e.g., plagues, extinctions), societal events (e.g., conflicts, war), and existential threats (e.g., extraterrestrial impacts, nuclear fallout). The top five most common events were 1) floods, 2) heat waves, 3) droughts, 4) severe precipitation, and 5) hurricanes (Figure 2).
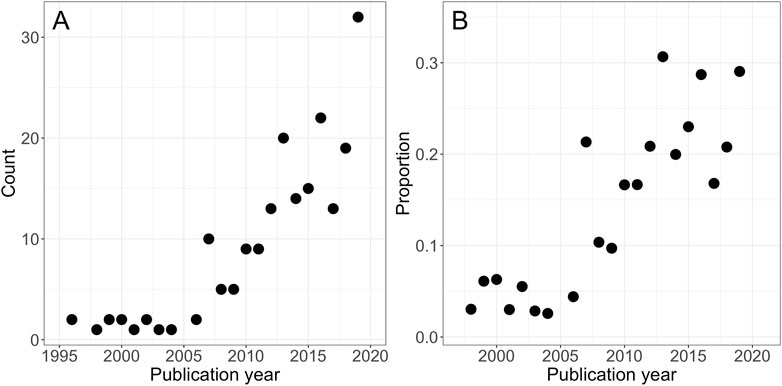
FIGURE 1. Number of publications per year (A) and adjusted for through-time increases in publication volume (B). Note that the two graphs have different starting years (Graph A starts at 1996, whereas Graph B starts at 1997). This is because of the way by which we attempted to correct for through-time increases in publication volume (see Methods).
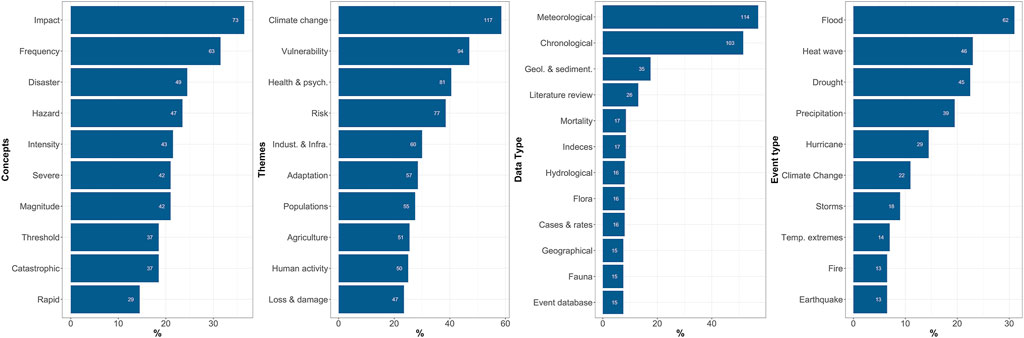
FIGURE 2. The top most common concepts, themes, data types, and event types in the literature sample (n = 200). Numbers within the bars denote the number of papers each occurred in.
Studies based in United States, China, and India were best represented, whereas those in countries in Africa, Oceania, eastern Europe, and western Asia were drastically underrepresented. Around one fifth of the world’s countries were represented by at least one study (21%, or 41 out of 195 countries), and around one fifth of all studies in the literature sample were global in focus (Figure 3). Two thirds of the articles focused on humans and human populations, followed by the environment/climate (49%), policy (33%), plants/animals (11%), and finally infrastructure (2%). Unsurprisingly, there was a great deal of overlap in article focus as, for example, articles that were concerned with the role of climate change in altering the frequency and magnitude of extreme events were also often concerned with the impacts that these changes might have on contemporary and future human populations.
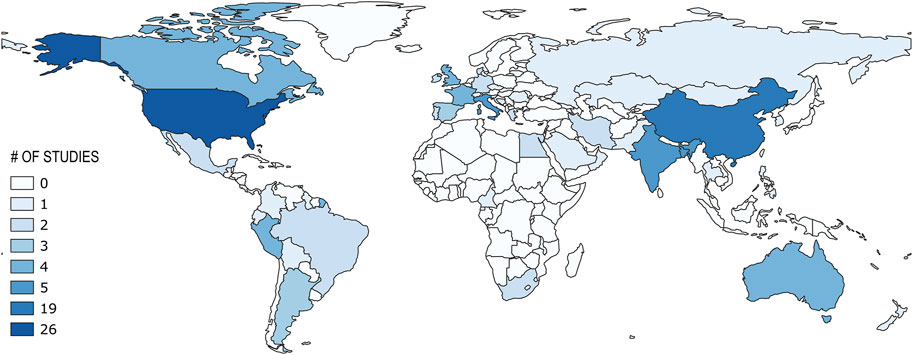
FIGURE 3. The number of extreme event studies by country. Note that 40 articles in our literature sample had a “global” focus and are included in the counts here, making the minimum count for any particular region 40. Studies of inter-country regions (e.g., the Mediterranean) were excluded.
Studies of contemporary (>1900 AD) extreme events were most common, followed by events from the early Holocene to the start of the early modern period (10,000 BC to 1500 AD), future events, Modern Era historical events (1500–1900 AD), and lastly early prehistorical events (<10,000 BC) (Table 3). In terms of scale, contemporary and future studies were primarily concerned with natural hazards, their impacts, and the role of anthropogenic climate change in altering the nature of hazardous events. Studies of past events were also concerned with natural hazards, but also focused on longer-term climatic events driven by broad scale changes in the Earth’s climate systems.
About half of all studies provided only a qualitative definition of extreme events, or no definition at all. In these instances, extreme events were described as simply being “rare” or “anomalous”; as an “abrupt,” “sharp,” or “rapid” change in some variable of interest like temperature; some peak in a time-series; or passing some vague threshold. Quantitative definitions of extreme events were the next most common (37% of papers) and included significant changes in some variable of interest, usually expressed as an absolute numerical value or ratio; passing some defined threshold such as daily precipitation; or the most extreme or some set of extreme values. Least common were statistical definitions of extreme events (22% of papers) which included exceeding some pre-defined statistical threshold (e.g., 95th percentile) or number of standard deviations, or were defined using more sophisticated analytical techniques (e.g., Peak-Over-Threshold). In terms of analyses, most studies (87% of papers) presented some sort of numerical or statistical analysis. Around a third of articles were primarily qualitative, most of these being literature reviews.
A total of 50 data types were used in the study of extreme events. The top five most common were 1) meteorological, 2) chronological, 3) geological and sedimentological, 4) literature review, and 5) mortality and indices data (tied) (Figure 2). Meteorological data consists of physical measures like temperature and wind velocity, as well as climate models. Chronological data comprises mostly time-series data, as well as radiocarbon data used in pre-Modern Era studies. Geological and sedimentological data includes a variety of measures related to the Earth’s surface and sediments (e.g., slope, grain size, magnetic susceptibility). Literature review and mortality data are self-explanatory. And lastly, index data relates to a variety of indices often used in climate studies (e.g., heat index).
We identified 105 concepts related to extreme events (Supplementary Table S2). The top five most common were 1) impact, 2) frequency, 3) disaster, 4) hazard, and 5) intensity (Figure 2). We also conducted a simple analysis of collocation strength (CS), collocations essentially being commonly co-occurring word pairs, such as “fast food.” The results show that impact is most strongly associated with the concepts magnitude (CS = 0.43), hazard (CS = 0.38), and disaster (CS = 0.38); frequency is most strongly associated with the concepts intensity (CS = 0.55), disaster (CS = 0.38), and impact (CS = 0.35); and disaster is most strongly associated with the concepts hazard (CS = 0.48), impact (CS = 0.38), and frequency (CS = 0.38).
A total of 90 themes related to extreme events were identified (Supplementary Table S3). The top five themes were 1) climate change, 2) vulnerability, 3) health and psychology, 4) risk, and 5) industrialization and infrastructure (Figure 2). Climate change was most strongly associated with the themes vulnerability (CS = 0.55), risk (CS = 0.49), and health and psychology (CS = 0.45); vulnerability was most strongly associated with health and psychology (CS = 0.61), risk (CS = 0.58), and climate change (CS = 0.55); and health and psychology was most strongly associated with vulnerability (CS = 0.61), risk (CS = 0.57), and industrialization and infrastructure (CS = 0.54).
Lastly, we ran a simple clustering analysis to explore co-occurring themes in the literature sample (Figure 4 and Supplementary Figure S3). The clustering analysis revealed little in terms of structure, although this is perhaps unsurprising given the broad scope of the literature surveyed and the type of data being analyzed. Still, some clusters do stand out that are worth exploring. The most apparent is a cluster of 48 articles focused on climate change on the one hand, and human health, vulnerability, and risk on the other (hereafter health, vulnerability, and risk). Three other clusters can be made out but are narrower in focus with respects to our tags. These are 27 articles related to human activity (hereafter human-environment interactions), 44 articles concerned mainly with human health and well-being (hereafter human health and well-being), and 64 articles focused on climate change (hereafter climate change dynamics). These clusters are discussed in detail below.
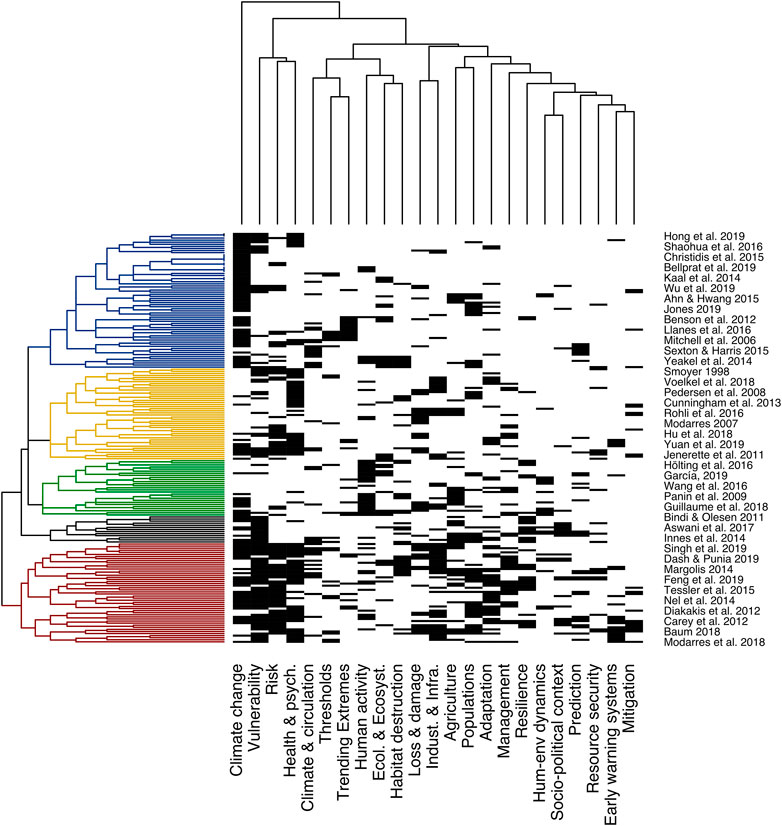
FIGURE 4. Heatmap showing paper likeness and theme clustering. Papers on the x-axis and themes on the y-axis. Only the top 20 themes were used in this clustering analysis. The clusters are color-coded as follows: health, vulnerability, and risk in red; human-environment interactions in green; human health and well-being in yellow; and climate change dynamics in blue. The full figure, with all 200 papers displayed, is presented in the Supplementary Material (Supplementary Figure S3). Please note that this figure was generated prior to some merging of themes and this dataset is provided as Appendix 4.
Breaking down the most common concepts by discipline revealed some interesting differences in how extreme events are defined and conceptualized (Figure 5) (see McPhillips et al., 2018 for a similar analysis). Impact, and the related concept disaster, are commonly used to define extreme events in the social sciences but occur much less frequently across the other disciplines, although impact was still the most common concept in earth sciences. Thresholds are also commonly applied in the earth sciences. In the climate and ecological sciences, the frequency of an extreme event appears to be particularly pertinent. Whereas in the palaeo sciences, the abruptness, rapidity, and magnitude are key to defining extreme events.
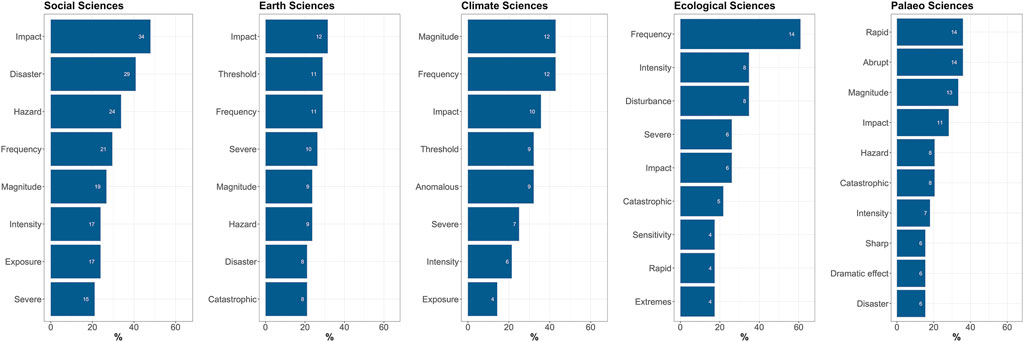
FIGURE 5. The common most concepts across the social, earth, climate, ecological, and palaeo sciences. Numbers within the bars denote the number of papers each concept occurred in.
Likewise, there were some evident differences when breaking down the most common themes by discipline (Figure 6). Social sciences were mostly concerned with the themes of human health and well-being, vulnerability, and risk. Earth sciences were also concerned with risk as well but also with the themes of human activity and loss and damage. Climate sciences were concerned with health and psychology and vulnerability, but also the themes of trending extremes and climate and circulation. Ecological sciences, understandably, had a strong focus on the theme ecology and ecosystems, as well as human activity and vulnerability. And lastly, the palaeo sciences had a strong focus on the themes of populations, agriculture, habitat destruction, and ecology and ecosystems. Climate change as a theme was common across all disciplines.
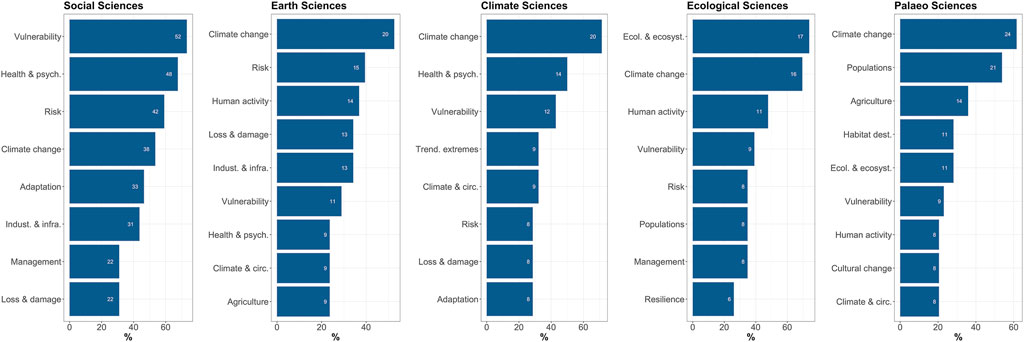
FIGURE 6. The common most themes across the social, earth, climate, ecological, and palaeo sciences. Numbers within the bars denote the number of papers each concept occurred in.
Discussion
Defining and conceptualizing extreme events
One of the primary goals of this review was to scrutinize how “extreme events” were being defined across biological, societal, and earth sciences. Concerningly, only half of all papers provided any sort of quantitative definition of what constitutes an extreme event, while the remainder gave only qualitative definitions or no definitions at all. Concerns over the lack of definitions in the extreme events literature have been raised elsewhere (McPhillips et al., 2018), and the present review suggests that the situation is even less clear when considering extreme events across an exceptionally broad range of spatial and temporal scales.
One key finding of this review was that studies of historical and prehistorical events rarely provide explicit, quantitative definitions of what constitutes an extreme event. This is perhaps unsurprising given the types of events commonly investigated and the resolution of the data available. Take for instance one commonly studied type of extreme event in history and prehistory—long-term climatic events. Such events are typically described as being an abrupt or rapid change in one or some set of climate parameters, and are often defined or discussed in relation to their magnitude and impacts (e.g., Innes et al., 2014; Crombé, 2018). These descriptions imply that some measure was used to determine magnitude and impact, and they further imply that comparisons must have been made to some baseline or reference period. However, rarely do scholars quantify what about a climate change event and/or its impacts qualify it as an extreme event and neither do they explicitly describe the baseline compared to which the given event is evidently extreme in some way. The same is often the case for more recent events. In our literature sample, observations about natural hazards like floods, hurricanes, and earthquakes were rarely accompanied by definitions for the relevant events. Only a handful of articles provided some sort of quantitative definition, which included metrics like total area affected, number of lives lost, and damage to property or income (e.g., Messerli et al., 2000; Gray and Mueller, 2012), and statistical definitions were even rarer. Again the baseline or reference condition is also rarely if ever explicitly described in the research.
Statistical definitions were far more common in studies of heat waves (e.g., Anderson and Bell, 2011; Baldwin et al., 2019) and severe precipitation (e.g., Coumou and Rahmstorf, 2012; Fischer and Knutti, 2015). The key reason for this being that these events are easy to measure on a continuum with simple metrics. Particularly common was the use of threshold values such as the 90th, 95th, or 99th percentile. In these instances, threshold values were typically based on the underlying statistical distribution derived from historic data relevant to the area of study—the previous 30 years temperature record from New York City, for example (e.g., Jegasothy et al., 2017; Matthews et al., 2017). Importantly, this considers the spatio-temporal dynamics of extreme events, as what is considered extreme in one place and/or time may not be considered extreme in another (Goodess, 2013). A few papers discussed other statistical approaches for identifying and modelling extreme events using Extreme Value Theory—such as the Peak Over Threshold method—although these were rarely applied in our sample (e.g., Evin et al., 2018; Singh et al., 2019). For a detailed discussion on statistical approaches for studying extreme events we refer readers to Ghil et al. (2011).
Indices were another common approach for describing and defining extreme events. Again, these were particularly common for climate-related events such as heat waves (e.g., Modarres et al., 2018; Sun et al., 2019), droughts (e.g., Modarres, 2007; Wu et al., 2015), and severe precipitation (e.g., Bartholy and Pongrácz, 2010; Gado et al., 2019). Most often, these indices combined two or more physical measures in an attempt to better capture the multivariate aspects of weather-related events and their impacts on human health and natural systems. The heat index (HI), for example, combines air temperature and relative humidity to provide an “apparent” or “feels like” temperature, with HI values >40.6°C considered to represent an extreme heat event with respects to human health and well-being (e.g., Matthews et al., 2017). Drought indices like the Palmer drought severity index (PDSI) and the Palmer hydrological drought index (PDHI), which combine various measures including temperature, soil moisture, and evapotranspiration, were popular tools for assessing drought exposure and damage in the agricultural sector (e.g., Rohli et al., 2016). Lastly, several studies defined extreme events using simple univariate thresholds, such as exceeding some daily rainfall amount or maximum temperature (e.g., Cunningham et al., 2013; Nel et al., 2014). As with the statistical distributions of single metrics (e.g., temperature or rainfall) described above, the studies involving indices must have employed a baseline or reference in order to judge when a given index reached some critical level. But in many cases the baseline was either not described in detail or seemed to have been defined arbitrarily or pragmatically given the data available (e.g., Sung et al., 2013; Wang et al., 2016).
It is clear from the above that the motivations for studying extreme events dictate how extreme events are defined, measured, and identified. This is made more apparent if we observe the most commonly used concepts across the various disciplines (Figure 5). As noted elsewhere (McPhillips et al., 2018), one of the key inter-disciplinary differences in the study of extreme events is the consideration of their impacts. In the social sciences, and to a lesser extent the earth sciences, the concepts of impact and disaster are key components of extreme events. Frequency appears to be a particularly important characteristic of extreme events in the climate and ecological sciences. Disturbance is unique to the latter and has a long history in the discipline (see McPhillips et al., 2018). Whereas rapidity, abruptness, and magnitude appear to be key concepts in the definition of extreme events in the palaeo sciences.
Thematic clusters in the literature
To further explore the thematic clusters in our literature sample we conducted a simple hierarchical clustering analysis using the top 20 themes only (see above). Although this clustering analysis is somewhat arbitrary, we believe that it captures well the extreme events themes in our literature sample. As mentioned above, we identified four broad thematic clusters: Health, vulnerability, and risk; Human-environment interactions; Human health and well-being; and Climate change dynamics.
Health, vulnerability, and risk
Papers in this cluster focus on the interaction between climate change on the one hand, and human health, vulnerability, and risk on the other. Climate change in these articles is typically discussed in relation to natural hazards. In fact, in many of these articles the authors make some reference to the role of climate change in increasing the frequency, duration, and intensity of natural hazards, now and into the future.
In at least 43 papers, the authors discussed the vulnerability of people, populations, and, to a lesser extent, systems, to extreme events. About half of these were concerned with the specific socioeconomic factors that make people particularly vulnerable to extreme events. This included vulnerable populations, such as those living in developing nations (e.g., Sena et al., 2014; Wang et al., 2019), as well as vulnerable individuals, typically the very young, the elderly, the poor, and people with underlying mental or physical health conditions (e.g., Baldwin et al., 2019; Smid et al., 2019). Some studies examined the specific link between natural hazards and human health (e.g., Green et al., 2010; Bush et al., 2014; Margolis, 2014), while others investigated the impacts of natural hazards on human livelihoods more generally (e.g., Gray and Mueller, 2012; Arias et al., 2016; Middleton et al., 2019).
Risk was the second most common theme in this cluster, occurring in at least 40 papers (e.g., Bush et al., 2014; Tessler et al., 2015). Several studies in this cluster were primarily concerned with determining the risks associated with present and future extreme events (e.g., Ameca y Juárez and Jiang, 2016; Abadie et al., 2019). Baldwin et al. (2019), for instance, argued that effective risk assessment of compound heat waves—which are predicted to become more frequent in the future—will require factoring in vulnerability from prior heat waves. In a similar vein, Coffel et al. (2018) modelled future wet bulb temperatures to assess which regions will be most at risk to extreme heat waves in the second half of the 21st century.
More common in this cluster, however, were studies focused on adaptive strategies for reducing risk. Included in these were a number of related concepts such as mitigation, risk management, climate change adaptation (CCA), disaster risk reduction (DRR), and early warning systems (EWS) (for reviews of these concepts we refer readers to Few, 2007; Birkmann and von Teichman, 2010). The approaches proposed in this cluster were understandably diverse and included various social, ecological, and infrastructural adaptive strategies. With regards to heat waves, Margolis (2014) lists a number of effective strategies for reducing heat wave risk. These included promoting good health, access to quality health care, acclimatizing to heat, reducing exposure to ambient heat and air pollution, formal emergency heat wave response plans, among other things, all of which have been shown to reduce risk and increase resilience to extreme heat events.
In at least 18 papers, the risks that climate change and natural hazards pose to agricultural activity was discussed (e.g., Feng et al., 2019; Middleton et al., 2019). This is perhaps unsurprising given 1) the obvious effects that events like floods and droughts can have on agricultural productivity, 2) the fact that data strongly suggests that such events are becoming more frequent and intense as a result of climate change, and 3) the economic and societal importance of agriculture. In one review, Bindi and Olesen (2011) suggested that climate change and extreme weather events will have varying impacts on agriculture across Europe in the coming decades, with increases in crop yields in northern Europe and large reductions in southern Europe. Other studies investigated the impacts of abrupt climate change on agricultural productivity in the past (e.g., Messerli et al., 2000; Feng et al., 2019). One such study found that over the last two millennia in China’s Hexi Corridor, abrupt cooling events have resulted in significant declines in grain yield which ultimately led to rises in socioeconomic crises and social disturbances (Feng et al., 2019). Several studies, including the one just mentioned, advocated for studying natural archives and palaeontological and archaeological records as models for examining how human populations and natural systems will respond to future climate change and extreme events (e.g., Riede, 2014; Hoffmann et al., 2015). We return to this point below.
Human-environment interactions
The second theme-group comprises papers focused primarily on the interactions between human activity and the environment. In at least 11 papers, the authors discussed the direct impacts that agricultural practices, such as land clearing, compaction, cropping, terrace construction, and so on, can have on the landscape (e.g., Giguet-Covex et al., 2012; Hölting et al., 2016). A clear message in these papers is that the impacts of such activities can be quite severe, for example, by promoting floods, erosion, dust storms, and denudation. Particularly problematic is when such activities coincide with significant climatic changes. In one study, Viglizzo and Frank (2006) discussed the role that land-clearing practices played in the late 20th century ecological and socio-economic collapse of the Argentine Pampas. Despite only being colonized by agriculturalists in the 19th century, intensive deforestation, over grazing, and over cropping, combined with particularly wet conditions starting in the 1970’s, increased the frequency and severity of floods so much so as to disrupt farming practices and send the region into a socio-economic crisis. Along similar lines, Giguet-Covex et al. (2012) found that flood frequency in the French Alps increased during the warmer periods of the Iron Age and the Roman Period, which the authors attribute to increased rainfall on the one hand, and intensive land-clearing practices that reduced rainfall infiltration and increased surface runoff on the other.
Several papers in this cluster highlighted the challenges associated with studying and understanding compound events such as those just mentioned. The spatial and temporal interactions between drivers and/or hazards can make predicting extreme events and their impacts more difficult than if all the drivers and hazards were independent, oftentimes leading to drastic underestimations of risk. In one study, Cuenca Cambronero et al. (2018) tested the impacts of 1) extreme temperature as a single stressor and 2) extreme temperature combined with biotic (i.e., food) and abiotic (i.e., insecticide) stress on the mechanisms of thermal tolerance in the water flea Daphnia magna. Importantly, the physiological and molecular responses to the combined stress environment were not predictable from the response to warming alone, and, therefore, if we want to accurately predict how species will respond to rising temperatures, it will be necessary to understand how multiple stressors act synergistically. Other ecological studies investigated the compounding impacts of extreme climatic events on the one hand, and environmental and human disturbances on the other, on various animal communities (e.g., Oliveira et al., 2014; Hölting et al., 2016).
Human health and well-being
Similar to the first cluster, the cluster human health and well-being addressed human health, but rather than treating it as a dimension of vulnerability, the research in this cluster focused specifically on the biophysical and psychological impacts of extreme events. In at least 21 papers, for instance, extreme events as a cause of mortality were investigated. Several papers analyzed mortality data directly. This included deaths caused by temperature extremes (e.g., Kalkstein et al., 2011; Li et al., 2016; Smith and Sheridan, 2019), floods (Thieken et al., 2016), air pollution (Vanos et al., 2015), earthquakes (Pawson, 2011), and tsunamis (Vött et al., 2019). A number of studies investigated how mortality rates have changed, or will change, in response to climate change and more frequent and intense extreme events. Kalkstein et al. (2011, p. 126), for example, developed time-series of meteorological and mortality data to assess how heat-related mortalities changed between 1975 and 2004 across 40 major U.S. cities. Encouragingly, their findings showed a significant decrease in heat-related deaths since 1996 for most of the major cities investigated, which the authors suggest is the result of “improvements in [excessive heat event] forecasting/recognition combined with an increased interest and commitment of public and private resources to [excessive heat event] education, notification, and response measures.”
Around 20 papers were concerned with human physical and mental health. Several studies investigated how extreme events might result in toxic levels of pollution and the possible impacts on human health (e.g., Pedersen et al., 2008; Li et al., 2010; Vanos et al., 2015). Through a combined literature review and geographic information systems (GIS) analysis, Plumlee et al. (2016) evaluated the impacts that an ARKStorm—a hypothetical but realistic “megastorm” scenario for California—could have on environmental contamination. Their results indicated that a number of anthropogenic and natural sources of contamination are vulnerable under an ARKStorm scenario including: numerous small (e.g., gas stations, dry cleaners) and large-scale (e.g., chemical manufacturing plants, agricultural operations) industrial and commercial facilities; livestock agricultural operations that, in addition to contaminants, have the potential to release deadly pathogens; urban centers and mining operations that have the potential to leach dangerous heavy metals and asbestos; and a number of other sources. Other studies were concerned with the psychological impacts that extreme events have on mental health (e.g., Pedersen et al., 2008; Thieken et al., 2016). In a study on the economic and psychological impacts of the 2003 European floods, survey respondents frequently reported feelings of “uncertainty about the future, worries with regard to family, existence and subsistence, and the future, fears of loss, panic, trauma, shock, crying fits or nervous breakdowns” (Thieken et al., 2016, p. 1527).
Lastly, a handful of studies were primarily concerned with the interaction between extreme events and disease (e.g., Andrade et al., 2019; Cheung et al., 2019). What is clear from these studies is that extreme events can, under the right circumstances, result in the proliferation of deadly diseases and other ailments. In one such study, Lara et al. (2009) investigated the impacts that cyclones and landslides have on counts of Vibrio—the genus of bacteria that causes cholera, among other illnesses—in an estuarine setting in Bangladesh. They found significantly higher abundances of Vibrio following both cyclones and landslides which the authors attribute to a higher amount of suspended particulate matter as a result of sediment disturbance.
Climate change dynamics
The final cluster comprises 64 articles intently focused on climate change, as defined by our tags. Around half of these articles were concerned with identifying trends in climate processes over the last century or so, or were aimed at predicting how these trends will change throughout the 21st century in response to climate change.
With respect to recent trends in extreme events, studies of precipitation were the most common (e.g., Cárdenas et al., 2016; Christy, 2019). In one study, Gado et al. (2019) analyzed precipitation data from 31 weather stations to examine the spatial and temporal changes in rainfall in Egypt between 1955 and 2016, showing that despite recent increases in extreme rainfall events in Egypt, rainfall trends have been on the whole decreasing since the 1950’s. In another study, Räsänen et al. (2016) analyzed tree-ring based drought indices to assess long-term variations in ENSO over Mainland Southeast Asia between 1650 and 2004. A key finding of this study was that most extremely wet and dry seasons in the past 355 years occurred during ENSO events, but that there has been considerable spatial and temporal variability in ENSO teleconnection and that this variability may be increasing. Given the strong influence that ENSO has over precipitation, and the potential impacts on heavily agricultural-dependent regions like Mainland Southeast Asia, a better understanding of the relationships between ENSO and the hydroclimate—now and into the future—is paramount (Räsänen et al., 2016)
Future projections of climate change and weather events were central to around 12 papers in this cluster. These included projections of extreme temperature (e.g., Huguet et al., 2008; Matthews et al., 2017), precipitation (Bartholy and Pongrácz, 2010), storm surge (Gaslikova et al., 2013), and air pollution events (Hong et al., 2019). Matthews et al. (2017), for instance, used global climate models (GCMs) to assess the impacts that 1.5°C and 2.0°C warming will have on the hazards of extreme events in China. Presently, heat waves occur in approximately half of the country—mainly in southern, eastern, and the southern part of northern China—and impact around 20% of people. Under a 2°C warming scenario, Matthews et al. (2017) predicted that approximately 80% of the country would experience heats waves—excluding only mountainous regions like the Tibetan Plateau and Changbai Mountains—and would impact roughly 80% of China’s population. In another study in China, Hong et al. (2019) combined climate, air quality, and epidemiological models to assess how China’s air quality will change under a RCP 4.5 climate change scenario. Alarmingly, the study found that fine particulate matter and ozone concentrations are expected to rise drastically across much of the country, resulting in an estimated additional ∼9,000–12,000 deaths. Despite focusing on different climate and hydrological phenomenon, all of these studies reached a similar conclusion—that is, as the climate continues to warm, extreme climatic events are going to become more frequent, more severe, longer lasting, and impact a greater number of people worldwide.
Precisely what is driving the uptick in extreme events was a theme in at least five papers in this cluster (e.g., Fischer and Knutti, 2015; Bellprat et al., 2019). In other words, to what degree, if at all, has human activity contributed to the rise in extreme events. In one analysis, Christidis et al. (2015) found that anthropogenic forcing has led to a considerable shift in the temperature distribution, indicating that human activity has had a strong influence on the frequency of extremely hot summers in Europe over the past 15 years. Moreover, and rather concerningly, the study suggested that summers like the 2003 European heat wave will become increasingly common by the mid-century and may even, under particularly severe climate and anthropogenic forcing scenarios, come to represent rather cold summers by the end of the century. Similarly, Fischer and Knutti (2015) found that 18% of moderate daily precipitation events are attributable to post-industrial anthropogenic warming.
Other studies instead emphasized the difficulties in detecting extreme events and linking a causal mechanism to them, which Coumou and Rahmstorf (2012) refer to as the “detection problem” and “attribution problem” (Coumou and Rahmstorf, 2012). Statistically speaking, detecting extreme events is difficult because extreme events are, by definition, rare occurrences, “living” in the tails of poorly constrained probability density functions. Even once detected, attributing an extreme event to a particular external forcing requires models to “get the unforced internal variability of extremes right as well as the spatiotemporal pattern of the forced response” (Coumou and Rahmstorf, 2012, p. 492). This typically requires multiple climate models/time series of sufficient length, as well as properly accounting for model errors in simulating the probabilities of extreme event occurrences, the latter often not being achieved in extreme event attribution studies (Bellprat et al., 2019). And lastly, there remain philosophical and epistemological questions surrounding extreme event attribution. These extend from what it means for something to be caused by something else in complex systems, and to what extent answers to such questions are influenced by politics and moral considerations (Hulme, 2014).
Lastly, around a third of all papers in this cluster focused on climate change and extreme events in history and prehistory. The reason for this cluster being so heavily dominated by these papers likely relates to themes common in these papers not being included in our hierarchical clustering analysis. Given the growing interest in past extreme events, for not only understanding human and ecosystem responses to climate change and extreme events in the past, but also as models for understanding how humans and ecosystems may react to climate change and extreme events now and into the future, we dedicate the following section to examining how extreme events are conceptualized and operationalized in the palaeo sciences.
Extreme events in history and prehistory
A total of 39 papers, or around one fifth of papers in our literature sample, were concerned with pre-Modern Era extreme events, which we define here as pre-dating 1500 AD. Progressively higher resolution pollen, sediment, ice core, and speleothem data mean that scientists are increasingly better positioned to identify and characterize past extreme climate events and natural hazards. Likewise, higher-resolution archaeological data and growing access to large online databases are providing archaeologists with new means by which to test the impacts that extreme events had on past human populations. The idea that past climate events and natural hazards can act as model systems for exploring how humans and ecosystems may respond to extreme events now and into the future is also gaining traction. In a recent review, not included as part of our literature sample, Burke et al. (2021) p. 1 make the case that studying the archaeological record and earth archives “offers opportunities to identify the factors that promoted human resilience in the past and apply the knowledge gained to the present, contributing a much-needed, long-term perspective to climate research.” Important to such a goal will be a common language to ensure that ideas and concepts are communicated effectively between and within disciplines. It is clear from our analysis, however, that no common language has yet emerged. With that in mind, we review the 39 palaeo sciences papers in a similar way to the clusters above to see specifically how researchers in the palaeo sciences define, think about, and study extreme events.
As stated above, a key distinguishing trait of palaeo science extreme event research is an emphasis on long-term climatic events. Studies of these are typically concerned with two main objectives. The first is trying to identify these climate events in pollen, sediment, speleothem, ice core, and other proxy records, to determine if a particular region experienced the event in any meaningful way (e.g., Huang et al., 2013; Innes et al., 2014). And the second is identifying, if any, the impacts that these events had on ecosystems and human populations, the latter often regarding the possible role of abrupt climatic change in driving cultural change, settlement abandonment, societal conflict, and population collapse (e.g., Madsen et al., 2007; Yeakel et al., 2014).
Two climatic events that have received considerable attention in this respect are the Medieval Climate Anomaly (MCA; c. 950–1250 AD) and the Little Ice Age (LIA; c. 1300–1850 AD), not least because they appear to have had significant impacts on human populations around the globe (e.g., Panin et al., 2009; Feng et al., 2019). Based on pollen assemblages from Anatolia, Bakker et al. (2013) argued that the MCA—which saw a rise in temperature across the North Atlantic region—played a key role in the resurgence of agriculture and revival of the Byzantine Empire around the 10th century AD. In contrast, the LIA—which saw a drop in temperatures following the MCA—has been linked to significant decreases in food production that resulted in “famines and plagues and, secondarily, conflicts and social disturbances” (Feng et al., 2019 p. 958).
Two other climatic events are the 8,200 BP and 4,200 BP events, both of which are thought to have played important roles in precipitating cultural change and population collapses around the globe (e.g., Ghilardi et al., 2012; Yeakel et al., 2014; Cheung et al., 2019). Broadly speaking, these events seemingly involved abrupt (i.e., within decades) transitions to much cooler and drier conditions and each had significant ecological and societal consequences, although detailed multidisciplinary studies often reveal the complex nature of these interactions (e.g., Groucutt et al., 2022, this issue). At the Tanjialing site in eastern China, for instance, pollen and biogeochemical data show that between c. 4,200–4,000 BP the region experienced a chronic drought which some have suggested reduced agricultural productivity and ultimately led to the collapse of the Shijiahe culture (Li et al., 2013). At the same time in central-western Korea, Ahn and Hwang (2015) found a significant decrease in archaeological radiocarbon dates, which the authors interpreted as a significant population decline or return to less sedentary lifeways in response to deteriorating climatic conditions.
The warm Bølling-Allerød (B-A; c. 14,700–12,900 BP) and the cold Younger Dryas (YD; c. 12,900–11,600 BP) are yet another two examples of extreme climatic events that had substantial and far reaching ecological and societal impacts (e.g., Wang et al., 2012; Mayewski et al., 2014). In one study, Wang et al. (2012) examined charcoal records from the Chinese Loess Plateau and found that the YD was associated with intense fire activity, possibly due to the expansion of grasslands, increased fuel loads, and longer fire seasons. Based on sediment marker and ice core data, the YD has been argued to have been triggered by an extraterrestrial impact that exploded over North America c. 12,900 BP, which, from the blast and ensuing climate cooling, caused the extinction of the vast majority of North America’s megafauna, as well as significant population declines and cultural changes among Native American populations (Firestone et al., 2007).
Several studies warned about the difficulties in separating climatic and anthropogenic influences in the proxy records often used to identify extreme climatic events (e.g., Bakker et al., 2013; Azuara et al., 2015). By studying lake sediments, pollen, and chironomids—a family of insects also known as midges or lake flies—from the Inner Hebrides in Scotland, Edwards et al. (2007) found that the opening of woodlands at around 8,200 BP was likely caused by human activity (e.g., land clearing for grazing) and not by climatic deterioration, as is often suggested. In two similar studies, Wang et al. (2012) and Miao et al. (2017) studied sedimentary charcoal records in East Asia to investigate how fire frequencies changed throughout the late Pleistocene and Holocene, arguing that increased fire activity starting in the mid-Holocene was likely driven by intense land-use practices and not climate change. In another study, Bakker et al. (2013) argued, based on sediment, pollen, and archaeological data, that the mid 12th century decline in agricultural activity was linked to socio-political change and not to the onset of the Little Ice Age. Studies such as these caution against conclusions drawn from simple correlations between observed changes in proxy records and known climatic events.
Other studies warned about separating truly abrupt climate changes from more gradual ones. Similar to the 8,200 BP and 4,200 BP events, albeit less well-known, the 9,300 BP event is also considered to have been an abrupt cooling event that precipitated ecological and cultural changes. In northwestern Europe, this event is associated with the drying up of lake and river beds and more frequent wildfires, and, from an archaeological standpoint, significant changes in raw material use, site distribution, and hunting strategies (Crombé, 2018). Crombé (2018, p. 353) argued, however, that some of these changes may have been initiated by climate changes in the preceding millennia, stating that without a high resolution chronology “a serious problem of equifinality remains.” Madsen et al. (2007) make a point about long-term climate cycles being the overall driver of the shift from the Paleolithic to the Neolithic, but that shorter-term centennial-to millennial-scale climate shifts acted as triggers for abrupt cultural and social change.
Lastly, as indicated at the start of this section, there appears to be a growing emphasis on drawing on past climate events and their impacts as a way for understanding how ecosystems and human populations may respond to extreme events in the future. Feng et al. (2019, p. 957), for instance, stated that to build “resilience against the impacts of climate change requires a deep understanding of social and environmental feedbacks to create a reliable buffer against future changes.” In that study, the authors examined the relationship between climate change on the one hand, and food security and social stability on the other, over the past two millennia in China’s Hexi Corridor. Based on their findings, the authors described a “domino effect” whereby society’s failure to respond to climate change led to cascading feedbacks that ultimately resulted in socioeconomic crises such as famine, plague, migrations, and conflicts. Paulette (2012) made a similar case for Bronze Age Mesopotamia, stating that “it is possible that an examination of ancient forms of hazard management and mitigation could resurrect some forgotten techniques that could be directly applied to the modern world.” And finally, by using historic and prehistoric volcanic eruptions as case studies, Riede (2014, p. 355) made that case that by studying the societal impacts of past natural disasters “we can derive historically informed evidence-based policy recommendations that can provide otherwise purely symbolic planning with operational and functional dimensions and that can be part of culturally sensitive social resilience strategies.”
Consilience
In addition to the differences highlighted above, we also identified some regularities and highlighted clusters of themes and concepts that appeared in the sample. Both the variability and the regularities are important for the useful convergence of knowledge. As we argued in the introduction, having multiple distinct pieces of evidence pointing to a particular conclusion lends more weight to that conclusion than would a similarly-sized collection of identical pieces of evidence (Wilson, 1999). The differences among extreme events in terms of type, scale, cause, and effect(s) can be an advantage for research. That advantage, however, can only be realized if different extreme events can be made comparable somehow, and that requires at least some broad similarities. Essentially, consilience in the study of extreme events requires some common denominators.
One broad similarity apparent from the patterns we observed is that researchers view extreme events within a particular temporal context. The vast majority of extreme event research we encountered involved the identification of a “rapid” change over time—e.g., a major swing in climate conditions, increase in local temperature, change in species composition, or the breach of a key threshold. The presence of “time” in the event definition has not always been explicitly recognized, but it is almost always present. Relatedly, a baseline or reference period of some kind was always present or implied, and it was the rate of change in conditions from the baseline to some state considered extreme that defined extreme events. So, “time” provides a context to all extreme event research, and importantly it usually appears in the denominator of an explicit or implicit rate function. Some of the papers we read included an explicit mathematically defined rate of change in some variable of interest (e.g., Marriner et al., 2013; Zhang et al., 2015), while others included discussions and word choice that only implied authors were thinking about rates of change (e.g., Innes et al., 2014; Moreno et al., 2019). A climate swing, for example, is considered extreme not just because of the magnitude of the difference in climate parameters between one time and another, but because of the rapidity of the observed change. Therefore, most of the extreme event research we encountered contextualizes extreme events in terms of rates of change. Consequently, the differences we observed in terms of temporal scale between event types or research domains can be marginalized by focusing on the rates of change. It may, at least in-part, be the rate of change that makes an event “extreme.” So, comparing events that appear to be incomparable because of vastly different temporal scales (e.g., mass extinctions and heatwaves) could be made possible by shifting the focus of extreme event research onto time-series analyses aimed at understanding rates of change.
The other broad similarity we observed is that many researchers discussed their particular events in terms of risk, vulnerability, and impact. To us, this broadly similar way of thinking indicates that extreme events may be “extreme” primarily because one or more observable phenomena are acutely sensitive to changes in another phenomenon. For example, humans have thermal tolerances, which means that changes in temperature matter for human health—and, crucially, an acute change in temperature can lead to an acute change in health status. This link between observable phenomena implies that extreme events might usefully be viewed as features of certain systems. Briefly, a system (vis. Systems Theory) is any collection of interrelated parts that make up a unitary whole—e.g., an ecosystem, a circulatory system, a computer system. Systems usually also exhibit the flow of materials, energy, or information between the interrelated components. Changes in one component can affect (cause changes in) another component. When isolating two components for study, the relationship between the components can be abstractly viewed like a mechanical device, say a circuit including a switch and bulb—flip the switch, turn on the bulb. And it may be the nature of the relationship between inputs and outputs in certain systems that gives rise to extreme events concerning the system. In fact, there is an extensive literature on taking a (dynamical) systems theory approach to climate change modelling that could serve as a jumping off point for building a unified theory of extreme events across domains (e.g., Scheffer, 2020). That research has indicated that environmental, ecological, and social systems can contain feedbacks between components, and that changes in one component can lead to cascades of changes in all inter-connected components. The measurements of components in these systems, we argue, can be scaled with respect to time—a change in health status per unit time, for example, compared to a change in temperature over the same interval—which would allow for potentially very different “extreme event systems” to be compared in terms of the nature of the relationship(s) between components in an abstract system. Essentially, the comparisons would take place between input and output rates (or changes therein) of measured variables. Exciting potential may lie in taking a time-series based approach to studying rate changes in dynamical systems across many domains—see for example recent work on predicting regime shifts in systems as varied as epileptic seizures and the Earth’s climate (Bury et al., 2021), based largely on studying rates of change over time in one or more variables.
Together, these two broad similarities suggest there are common denominators that could be used in comparative research of extreme events. More research is needed, but it is encouraging to see that “extreme events” comprise a broad class of phenomena and that radically interdisciplinary research into them could be fruitful. Importantly, comparative extreme event research could potentially yield new insights into extreme events of all types, at all spatio-temporal scales. With better general insights in-hand, we expect that predictions concerning future extreme events will be more accurate and, thereby, more useful for planning and preparation. Better predictions are clearly needed if climate change continues to increase the magnitude and frequency of extreme events over the next century or longer.
Future directions
In our view, the present review identified several important lacunae and biases in the extreme events literature. The first of these relates to the fact that many studies do not provide an explicit definition of what constitutes an extreme event, an issue that has been raised recently by others (e.g., Smith, 2011; McPhillips et al., 2018; Broska et al., 2020). Moreover, even when provided, definitions of extreme events vary greatly between, and oftentimes within, disciplines. According to some, the growing interest in extreme events across a broadening range of disciplines makes a “common and comprehensive definition more crucial than ever” (Broska et al., 2020, p. 1). It may also be important to develop a common nomenclature in describing and studying extreme events to help bridge interdisciplinary divides. As we noted, we think there are key similarities that could support a general definition and bring consilience to the study of extreme events, but further research is going to be necessary. That research will have to include attempts to compare events from across a range of spatiotemporal scales and possibly from domains of research that may appear to be largely unrelated.
A key part of this research may involve taking a dynamical systems approach (see Scheffer, 2020), as we suggested earlier. Dynamical systems have been an active area of research across academic disciplines for decades and a branch of mathematics for a century. Its primary focus is on the study of change (usually over time) in the state of a system measured by one or more variables (Strogatz, 2018). It is abstract and, so, can be applied in a variety of contexts. Dynamical systems research is often concerned with the identification of certain system features like “tipping points,” “regimes,” and “attractors,” which are now familiar terms in ecology and climate change literature (Lenton et al., 2008) and can be regularly found in popular media reports. A tipping point, for instance, is a parameter value (e.g., global temperature) at which a system will change states from one region of parameter space (set of potential values) to another. Different regions of potential parameter values are often called “regimes.” And “attractors” are parameter values that a system will tend toward. These and other features of dynamical systems—indeed the theory and math of Dynamical Systems more generally—are becoming an integral part of climate change science (Ghil and Lucarini, 2020). The famous hypothesized collapse of the thermohaline circulation (Marotzke, 2000) is an example of a complex climate system reaching a tipping point that shifts the Earth’s climate from one regime into another. A dynamical systems analysis is concerned with studying the long-term evolution and behavior of a system in abstract terms with a focus on understanding the qualitative features of potential trajectories the system might take. This sort of abstraction makes it an attractive approach for comparing extreme events in different domains with different time scales. To compare extreme events, we propose viewing them as features of dynamical systems and then abstractly defining a multicomponent system model to represent the relevant processes. For example, heatwaves could be compared to floods by considering each phenomenon as a two-component system. One component would represent the environment and the other humans in the environment. Changes in the first component (an increase in heat, or rainfall) affect changes in the second (hospital visits, or property damage respectively). Each component has traits that can be measured and quantified, and those quantifiable traits can be used to find parameters in a statistical or dynamical systems model. If the model is parameterized in terms of rates, as we explained earlier, then the two quite different systems can be compared directly. One possible outcome of this exercise is that the two systems, even when modelled with the same framework, share no similarities. In that case, the conclusion would be that the events are not part of a common class of “extreme event” phenomena, and perhaps no common class exists. Another outcome, however, might be that the two models share important features—like parameter values, attractors, and/or tipping points. In that case, we could conclude that these two distinct phenomena frequently labelled “extreme” in the literature do in fact belong to a common class. While we cannot, as yet, confidently define extreme events in an inclusive way, we see a clear path toward determining whether a single definition is useful and/or warranted. That way forward involves taking a systems approach and leveraging our observation that rates of change seem to be a common feature in the research on extreme events we examined.
Another area in need of attention relates to geographical biases. Studies based in United States, China, and India were best represented, whereas those in countries in Africa, Oceania, eastern Europe, and western Asia were drastically underrepresented (Figure 3). Around one fifth of the worlds countries were represented by at least one study (21%, or 41 out of 195 countries), and around one fifth of all studies in the literature sample were global in focus (Figure 3). What is particularly interesting about this bias is that it is at odds with the apparent interest in vulnerability, as expressed in the literature. That is to say, that for some of arguably the most vulnerable groups of people in the world, there has been very little research into how they might be impacted by climate change and extreme events, at least as far as our literature sample is concerned. The clearest example here is Africa. Only eight papers in our literature sample (4% of papers) focused specifically on Africa: two of these were concerned with events earlier in the Holocene (Marriner et al., 2013; Yeakel et al., 2014), and one examined the impacts of heat waves on birds (Cunningham et al., 2013). That is to say, the number of studies concerned with the impacts of extreme events on contemporary Africans can be counted on one hand. Another clear example here is islands. According to the IPCC (2021), small island states are some of the most vulnerable to climate change and extreme events, notably sea level rise, droughts, and tropical storms. And yet, only four studies in our literature sample focused specifically on small island states (Yamano et al., 2007; Green et al., 2010; Aswani et al., 2017; Smith and Dressler, 2019). Therefore, a greater focus on vulnerable regions is critical if we are to reduce the impacts of climate change and extreme events on some of the world’s most vulnerable groups.
A third area in need of attention is causality. Most studies in our literature sample either explicitly or implicitly dealt with causality in some way—humans and their activities as drivers of climate change, for instance. However, very few studies tackled the epistemology of causality directly, and those that did tended to highlight the difficulties in identifying causal relationships in complex systems (e.g., Schneider and Root, 1996; Coumou and Rahmstorf, 2012; Lu et al., 2016). One of the key reasons for this is that extreme events are, by definition, rare, and most observational records relatively short, making detecting systematic changes in extreme event occurrence difficult (Stott et al., 2016). To this we would also add the sizable challenge of identifying causal relationships between extreme events given only very infrequent, sometimes isolated co-occurrences. Take for example research on societal collapses in the past thought to be triggered by climatic events, such as the abovementioned abrupt cooling episodes at 4,200 BP and 8,200 BP and dramatic societal upheavals that followed (e.g., Yeakel et al., 2014; Cheung et al., 2019). Dramatic societal collapses tend to be rare and so do major climate transitions or events—at least, rare on the scale of human generations as far as we know. Co-occurrences, then, between major climate events and societal collapses can be expected to be even rarer, assuming the two event types are not universally related. Their rarity, both individually and in combination, makes a causal relationship between them hard to demonstrate. Distinguishing potential casual factors from mere coincidence may, therefore, require special methodological, philosophical, and theoretical frameworks (e.g., Hulme, 2014; Stott et al., 2016). Some of the theoretical work may relate to extreme event prediction, which is an area of research that has included methodological attention to the challenges raised by event rarity in statistical analyses (e.g., “extreme value theory”). Future research could proceed from that work and recent work on extreme event attribution (Shepherd, 2016). In particular, the latter would be highly relevant for systems approaches seeking to explain ecological or societal responses to climatic or meteorological extreme events, but we must also highlight that there are important challenges and limitations related to event attribution methods (van Oldenborgh et al., 2021). Needless to say, though, understanding extreme events and planning for them will require a firm understanding of the relevant causal relationships and our review indicates there is a lot of room for methodological and theoretical development.
A fourth area in need of attention are compound extreme events. While only a handful of studies in our literature sample focused on compound events (e.g., Oliveira et al., 2014; Baldwin et al., 2019), a key takeaway from these studies was that their impacts oftentimes equate to more than the sum of their parts. In other words, the impacts of compound extreme events—successive flooding and droughts, for example—can have particularly devastating impacts on biological, societal, and earth systems. As argued recently (Raymond et al., 2020), successive events and events of different types that co-occur can rapidly overwhelm even well-prepared societies and, as with isolated events, will tend to affect most those communities with the highest baseline risk levels. Raymond and others (2020) urge deeper interdisciplinary research and collaboration among policy makers and other stakeholders in order to understand the potential impacts of compound extreme events on various societies at local, regional, and larger scales. Crucially, as extreme events become more frequent, longer lasting, and more intense, so too will compound extreme events, and there is now strong evidence to suggest that this is already the case (e.g., Baldwin et al., 2019). Critical, then, is a good understanding of how extreme events interact and compound with one another so that their impacts can be effectively mitigated. Achieving that understanding, we argue, may require not just interdisciplinary research, but comparative extreme events research based on some common framework.
Another area in need of attention relates to the use of past extreme events as models for contemporary and future extreme events (e.g., Mayewski et al., 2014; Feng et al., 2019). Looking to the past would expand the database for extreme events and human societal responses to those events. Having a bigger, more varied database would lead to a deeper understanding of extreme events and, thereby, better predictions for future events. Unfortunately, though, while there are now hundreds of scientific papers published every year on long-term human environment interaction based on palaeoclimatological and archaeological data (e.g., Carleton and Collard, 2020; Davis, 2020), most of these report conclusions that are not sufficiently rigorous or reliable to be used as a basis for policy or planning (Smith, 2021). The relevant measurement and chronological uncertainties are generally poorly handled, if at all, in typical case studies and systematic quantitative comparisons between palaeo climate records and archaeological records are uncommon. This problem, we think, has to do in part with the inherent and often irreducible uncertainties in palaeo datasets. But it also stems from a dearth of appropriate statistical models and accessible, user-friendly computational tools that do not require software development skills. Only recently have we seen a greater emphasis on methodological research concerned with all-source uncertainty quantification (e.g., Parnell et al., 2015; Boers et al., 2017). So, while we argue that past extreme events (and human responses to them) should be investigated in order to better understand extreme event phenomena in general, the scientific community needs to devote more time and resources to developing the necessary methodological and computational tools. Only then will we be able to use past events as models for future ones.
And lastly, from a review-oriented methodological standpoint, we recommend using collector’s plots (also commonly known as species accumulation curves) when conducting systematic reviews of literature. These should be done regularly throughout the analysis and the plots inspected. This will 1) ensure that sufficient sample sizes are reached and 2) will reduce sampling redundancy—it may be a waste of time to analyze, say, 200 papers, when the data of interest is adequately captured with fewer papers.
Study limitations
We recognize a number of important limitations and biases that characterize our study. The first of these relates to the scope of the literature search and systematic review. Although our review was intentionally broad with respects to discipline and spatial and temporal scale, it did not include all the ways scholars consider extreme events. In addition to those discussed above, events that have been considered “extreme” in the literature include economic events like recession and depression and medical events like seizures and cardiac arrest (see Introduction). Expanding the scope to include economics and medical science—and any disciplines interested in extreme events for that matter—should provide a more comprehensive insight into how extreme events are conceptualized and operationalized across an even broader range of disciplines. To that end, future work should extend the literature search beyond Web of Science to literature databases like MEDLINE, Embase, PsycINFO, and JSTOR to better capture research across disciplines such as medicine, economics, and history.
A second limitation involves likely biases in the literature favoring sensational, trendy, or otherwise attentional grabbing research topics. Since our literature sample was randomly selected, we likely observed topics, themes, and event types that were most frequently investigated, which means our sample reflects the interests of the research community. In part, that was intentional because we aimed to discover how scientists thought about, identified, measured, and studied extreme events—i.e., in part we intended to understand the scholarly community, biases or not. As a result, any biases affecting what research was common in the literature would have no negative effect on our inferences. But, we also aimed to determine whether extreme events could be studied as a general class of phenomena. This second objective meant that our focus was also in part on extreme events rather than just what researchers thought about them. Thus, research biases could potentially have obscured our view of extreme event phenomena since our sample of event types would have been filtered by the researchers’ biases. In future, it may be worthwhile scouring the literature for more rarely-discussed event types, themes, topics, regions, and time periods in order to investigate whether there are widespread relevant biases potentially skewing our understanding of extreme event phenomena more broadly.
The third limitation we recognize concerns the search terms used in our study. While we selected search terms to capture extreme events literature that intersect the biological, societal, and earth sciences, additional search terms may have improved our literature search and thereby our literature sample. For instance, McPhillips et al. (2018) found the terms “impact,” “frequency,” and “disturbance” to be key components of extreme events in the social, climate, and ecological sciences. To better capture these disciplines, these search terms, and likely others, should be considered in the future.
The final limitation we recognize concerns the lack of cross-validation during the coding and tagging of the articles. So, while the two authors (MS, WCC) that conducted the analysis of the papers worked closely and regularly consulted on the meaning of tags and how and when to apply them, no efforts were made to cross-validate the tags as a test for intra-observer variability. We see this as likely being less of an issue for the most common tags in our data set. For example, concepts such as climate change or vulnerability are fairly unambiguous and we suspect that the two authors would have tagged these similarly. So, while intra-observer variability was likely present, we suspect that it would have had little impact on the most common tags and therefore our results overall.
Conclusion
On the one hand, our systematic analysis revealed a great deal of variability among extreme event papers with respect to research interests, themes, concepts, and definitions. On the other hand, we found a number of key similarities in how people think about and study extreme events across a diverse range of disciplines. Namely, we found that scholars tend to view extreme events within a particular temporal context, and quite often in terms of rates of change. We also found that researchers often discuss their particular events in terms of risk, vulnerability, and impact. We believe that these “common denominators” may be useful in developing a universal and comprehensive definition of what constitutes an extreme event and should allow for more comparative research into extreme events at all spatio-temporal scales. Taken together, a common understanding of extreme events, a greater emphasis on comparative research across disciplines, and addressing the biases identified in this study, we think, will be key for better predicting, planning, and preparing for extreme events now and into the future.
Data availability statement
All code and data needed to run the analyses are provided as appendices with the article and can also be found at https://github.com/Stewie1302/ExtremeEvents-Review.
Author contributions
MS, CC, and HG conceived the idea. MS and CC analyzed the articles. MS conducted the data analysis. All authors contributed to the writing of the manuscript and approved the submitted version.
Funding
The authors thank the Max Planck Society for funding.
Acknowledgments
The authors thank the reviewers for the helpful comments.
Conflict of interest
The authors declare that the research was conducted in the absence of any commercial or financial relationships that could be construed as a potential conflict of interest.
Publisher’s note
All claims expressed in this article are solely those of the authors and do not necessarily represent those of their affiliated organizations, or those of the publisher, the editors and the reviewers. Any product that may be evaluated in this article, or claim that may be made by its manufacturer, is not guaranteed or endorsed by the publisher.
Supplementary material
The Supplementary Material for this article can be found online at: https://www.frontiersin.org/articles/10.3389/feart.2022.786829/full#supplementary-material
References
Abadie, L. M., Chiabai, A., and Neumann, M. B. (2019). Stochastic diffusion models to describe the evolution of annual heatwave statistics: A three-factor model with risk calculations. Sci. Total Environ. 646, 670–684. doi:10.1016/j.scitotenv.2018.07.158
Ahn, S.-M., and Hwang, J. H. (2015). Temporal fluctuation of human occupation during the 7th–3rd millennium cal BP in the central-western Korean Peninsula. Quat. Int. 384, 28–36. doi:10.1016/j.quaint.2015.04.038
Albeverio, S., Jentsch, V., and Kantz, H. (2006). Extreme events in nature and society. Heidelberg: Springer Berlin.
Ameca y Juárez, E. I., and Jiang, Z. (2016). Flood exposure for vertebrates in China’s terrestrial priority areas for biodiversity conservation: Identifying internal refugia. Biol. Conserv. 199, 137–145. doi:10.1016/j.biocon.2016.04.021
Anderson, G. B., and Bell, M. L. (2011). Heat waves in the United States: Mortality risk during heat waves and effect modification by heat wave characteristics in 43 U.S. Communities. Environ. Health Perspect. 119, 210–218. doi:10.1289/ehp.1002313
Andrade, L., O’Malley, K., Hynds, P., O’Neill, E., and O’Dwyer, J. (2019). Assessment of two behavioural models (HBM and RANAS) for predicting health behaviours in response to environmental threats: Surface water flooding as a source of groundwater contamination and subsequent waterborne infection in the Republic of Ireland. Sci. Total Environ. 685, 1019–1029. doi:10.1016/j.scitotenv.2019.06.249
Arias, P. A., Villegas, J. C., Machado, J., Serna, A. M., Vidal, L. M., Vieira, C., et al. (2016). Reducing social vulnerability to environmental change: Building trust through social collaboration on environmental monitoring. Weather, Clim. Soc. 8, 57–66. doi:10.1175/WCAS-D-15-0049.1
Aswani, S., van Putten, I., and Miñarro, S. (2017). Environmental and social recovery asymmetries to large-scale disturbances in small island communities. Nat. Hazards 86, 241–262. doi:10.1007/s11069-016-2685-2
Azuara, J., Combourieu-Nebout, N., Lebreton, V., Mazier, F., Müller, S. D., and Dezileau, L. (2015). Late Holocene vegetation changes in relation with climate fluctuations and human activity in Languedoc (southern France). Clim. Past. 11, 1769–1784. doi:10.5194/cp-11-1769-2015
Bakker, J., Paulissen, E., Kaniewski, D., Poblome, J., De Laet, V., Verstraeten, G., et al. (2013). Climate, people, fire and vegetation: New insights into vegetation dynamics in the eastern mediterranean since the 1st century AD. Clim. Past. 9, 57–87. doi:10.5194/cp-9-57-2013
Baldwin, J. W., Dessy, J. B., Vecchi, G. A., and Oppenheimer, M. (2019). Temporally compound heat wave events and global warming: An emerging hazard. Earths Future 7, 411–427. doi:10.1029/2018ef000989
Bartholy, J., and Pongrácz, R. (2010). Analysis of precipitation conditions for the Carpathian Basin based on extreme indices in the 20th century and climate simulations for 2050 and 2100. Phys. Chem. Earth, Parts A/B/C 35, 43–51. doi:10.1016/j.pce.2010.03.011
Bellprat, O., Guemas, V., Doblas-Reyes, F., and Donat, M. G. (2019). Towards reliable extreme weather and climate event attribution. Nat. Commun. 10, 1732. doi:10.1038/s41467-019-09729-2
Beniston, M. (2004). The 2003 heat wave in Europe: A shape of things to come? An analysis based on Swiss climatological data and model simulations. Geophys. Res. Lett. 31, 2. doi:10.1029/2003gl018857
Benton, M. J. (1995). Diversification and extinction in the history of life. Science 268, 52–58. doi:10.1126/science.7701342
Bindi, M., and Olesen, J. E. (2011). The responses of agriculture in Europe to climate change. Reg. Environ. Change 11, 151–158. doi:10.1007/s10113-010-0173-x
Birkmann, J., and von Teichman, K. (2010). Integrating disaster risk reduction and climate change adaptation: Key challenges—scales, knowledge, and norms. Sustain. Sci. 5, 171–184. doi:10.1007/s11625-010-0108-y
Boers, N., Goswami, B., and Ghil, M. (2017). A complete representation of uncertainties in layer-counted paleoclimatic archives. Clim. Past. 13, 1169–1180. doi:10.5194/cp-13-1169-2017
Brooks, N. (2003). Vulnerability, risk and adaptation: A conceptual framework. Tyndall Centre Clim. Change Res. Work. Pap. 38, 1–16.
Broska, L. H., Poganietz, W.-R., and Vögele, S. (2020). Extreme events defined—a conceptual discussion applying a complex systems approach. Futures 115, 102490. doi:10.1016/j.futures.2019.102490
Burke, A., Peros, M. C., Wren, C. D., Pausata, F. S. R., Riel-Salvatore, J., Moine, O., et al. (2021). The archaeology of climate change: The case for cultural diversity. Proc. Natl. Acad. Sci. U. S. A. 118, e2108537118. doi:10.1073/pnas.2108537118
Bury, T. M., Sujith, R. I., Pavithran, I., Scheffer, M., Lenton, T. M., Anand, M., et al. (2021). Deep learning for early warning signals of tipping points. Proc. Natl. Acad. Sci. U. S. A. 118, e2106140118. doi:10.1073/pnas.2106140118
Bush, K. F., O’Neill, M. S., Li, S., Mukherjee, B., Hu, H., Ghosh, S., et al. (2014). Associations between extreme precipitation and gastrointestinal-related hospital admissions in Chennai, India. Environ. Health Perspect. 122, 249–254. doi:10.1289/ehp.1306807
Cárdenas, O. L., Campos, M. N., Sevilla, P. M., Guerrero, R. R., Ocampo, H. A. G., and Moreno, M. N. H. (2016). Estimating trends and return periods of daily extreme precipitation associated with tropical cyclones in the core North American monsoon. Pol. J. Environ. Stud. 25, 2283–2292. doi:10.15244/pjoes/64161
Carleton, W. C., and Collard, M. (2020). Recent major themes and research areas in the study of human-environment interaction in prehistory. Environ. Archaeol. 25, 114–130. doi:10.1080/14614103.2018.1560932
Cheung, C., Zhang, H., Hepburn, J. C., Yang, D. Y., and Richards, M. P. (2019). Stable isotope and dental caries data reveal abrupt changes in subsistence economy in ancient China in response to global climate change. PLoS One 14, e0218943. doi:10.1371/journal.pone.0218943
Christidis, N., Jones, G. S., and Stott, P. A. (2015). Dramatically increasing chance of extremely hot summers since the 2003 European heatwave. Nat. Clim. Chang. 5, 46–50. doi:10.1038/nclimate2468
Christy, J. R. (2019). Examination of extreme rainfall events in two regions of the United States since the 19th century. AIMS Environ. Sci. 6, 109–126. doi:10.3934/environsci.2019.2.109
Coffel, E. D., Horton, R. M., and de Sherbinin, A. (2018). Temperature and humidity based projections of a rapid rise in global heat stress exposure during the 21st century. Environ. Res. Lett. 13. doi:10.1088/1748-9326/aaa00e
Comfort, L. K. (2002). Managing intergovernmental responses to terrorism and other extreme events. Publius 32, 29–50. doi:10.1093/oxfordjournals.pubjof.a004970
Coumou, D., and Rahmstorf, S. (2012). A decade of weather extremes. Nat. Clim. Chang. 2, 491–496. doi:10.1038/nclimate1452
Crombé, P. (2018). Abrupt cooling events during the Early Holocene and their potential impact on the environment and human behaviour along the southern North Sea basin (NW Europe). J. Quat. Sci. 33, 353–367. doi:10.1002/jqs.2962
Csardi, G., and Nepusz, T. (2006). The igraph software package for complex network research. InterJournal, complex Syst. 1695, 1–9.
Cuenca Cambronero, M., Beasley, J., Kissane, S., and Orsin, L. (2018). Evolution of thermal tolerance in multifarious environments. Molecular Ecology 27, 4529–4541. doi:10.1111/mec.14890
Cunningham, S. J., Kruger, A. C., Nxumalo, M. P., and Hockey, P. A. R. (2013). Identifying biologically meaningful hot-weather events using threshold temperatures that affect life-history. PLoS One 8, e82492. doi:10.1371/journal.pone.0082492
Davis, D. S. (2020). Studying human responses to environmental change: Trends and trajectories of archaeological research. Environ. Archaeol. 25, 367–380. doi:10.1080/14614103.2019.1639338
Edwards, K. J., Langdon, P. G., and Sugden, H. (2007). Separating climatic and possible human impacts in the early Holocene: Biotic response around the time of the 8200 cal. Yr BP event. J. Quat. Sci. 22, 77–84. doi:10.1002/jqs.1018
Evin, G., Curt, T., and Eckert, N. (2018). Has fire policy decreased the return period of the largest wildfire events in France? A bayesian assessment based on extreme value theory. Nat. Hazards Earth Syst. Sci. 18, 2641–2651. doi:10.5194/nhess-18-2641-2018
Feng, Q., Yang, L., Deo, R. C., AghaKouchak, A., Adamowski, J. F., Stone, R., et al. (2019). Domino effect of climate change over two millennia in ancient China’s Hexi Corridor. Nat. Sustain. 2, 957–961. doi:10.1038/s41893-019-0397-9
Few, R. (2007). Health and climatic hazards: Framing social research on vulnerability, response and adaptation. Glob. Environ. Change 17, 281–295. doi:10.1016/j.gloenvcha.2006.11.001
Firestone, R. B., West, A., Kennett, J. P., Becker, L., Bunch, T. E., Revay, Z. S., et al. (2007). Evidence for an extraterrestrial impact 12,900 years ago that contributed to the megafaunal extinctions and the Younger Dryas cooling. Proc. Natl. Acad. Sci. U. S. A. 104, 16016–16021. doi:10.1073/pnas.0706977104
Fischer, E. M., and Knutti, R. (2015). Anthropogenic contribution to global occurrence of heavy-precipitation and high-temperature extremes. Nat. Clim. Chang. 5, 560–564. doi:10.1038/nclimate2617
Frederikse, T., Buchanan, M. K., Lambert, E., Kopp, R. E., Oppenheimer, M., Rasmussen, D. J., et al. (2020). Antarctic Ice Sheet and emission scenario controls on 21st-century extreme sea-level changes. Nat. Commun. 11, 390. doi:10.1038/s41467-019-14049-6
Gado, T. A., El-Hagrsy, R. M., and Rashwan, I. M. H. (2019). Spatial and temporal rainfall changes in Egypt. Environ. Sci. Pollut. Res. Int. 26, 28228–28242. doi:10.1007/s11356-019-06039-4
Gaslikova, L., Grabemann, I., and Groll, N. (2013). Changes in North Sea storm surge conditions for four transient future climate realizations. Nat. Hazards 66, 1501–1518. doi:10.1007/s11069-012-0279-1
Ghil, M., and Lucarini, V. (2020). The physics of climate variability and climate change. Rev. Mod. Phys. 92, 035002. doi:10.1103/RevModPhys.92.035002
Ghil, M., Yiou, P., Hallegatte, S., Malamud, B. D., Naveau, P., Soloviev, A., et al. (2011). Extreme events: Dynamics, statistics and prediction. Nonlinear process. geophys. 18, 295–350. doi:10.5194/npg-18-295-2011
Ghilardi, M., Psomiadis, D., Cordier, S., Delanghe-Sabatier, D., Demory, F., Hamidi, F., et al. (2012). The impact of rapid early- to mid-Holocene palaeoenvironmental changes on Neolithic settlement at Nea Nikomideia, Thessaloniki Plain, Greece. Quat. Int. 266, 47–61. doi:10.1016/j.quaint.2010.12.016
Ghirardi, L., Bisoffi, G., Mirandola, R., Ricci, G., and Baccini, M. (2015). The impact of heat on an emergency department in Italy: Attributable visits among children, adults, and the elderly during the warm season. PLoS One 10, e0141054. doi:10.1371/journal.pone.0141054
Giguet-Covex, C., Arnaud, F., Enters, D., Poulenard, J., Millet, L., Francus, P., et al. (2012). Frequency and intensity of high-altitude floods over the last 3.5 ka in northwestern French Alps (Lake Anterne). Quat. Res. 77, 12–22. doi:10.1016/j.yqres.2011.11.003
Goddard, P. B., Yin, J., Griffies, S. M., and Zhang, S. (2015). An extreme event of sea-level rise along the Northeast coast of North America in 2009–2010. Nat. Commun. 6, 1–9. doi:10.1038/ncomms7346
Goodess, C. M. (2013). How is the frequency, location and severity of extreme events likely to change up to 2060? Environ. Sci. Policy 27, S4–S14. doi:10.1016/j.envsci.2012.04.001
Gotelli, N. J., and Colwell, R. K. (2001). Quantifying biodiversity: Procedures and pitfalls in the measurement and comparison of species richness. Ecol. Lett. 4, 379–391. doi:10.1046/j.1461-0248.2001.00230.x
Gray, C. L., and Mueller, V. (2012). Natural disasters and population mobility in Bangladesh. Proc. Natl. Acad. Sci. U. S. A. 109, 6000–6005. doi:10.1073/pnas.1115944109
Green, D., Alexander, L., Mclnnes, K., Church, J., Nicholls, N., and White, N. (2010). An assessment of climate change impacts and adaptation for the Torres Strait Islands, Australia. Clim. Change 102, 405–433. doi:10.1007/s10584-009-9756-2
Groucutt, H. S., Carleton, W. C., Fenech, K., Gauci, R., Grima, R., Scerri, E. M. L., et al. (2022). The 4.2 ka event and the end of the Maltese “temple period. Front. Earth Sci. 9, 771683. doi:10.3389/feart.2021.771683
Hoffmann, G., Grützner, C., Reicherter, K., and Preusser, F. (2015). Geo-archaeological evidence for a Holocene extreme flooding event within the arabian sea (ras al Hadd, Oman). Quat. Sci. Rev. 113, 123–133. doi:10.1016/j.quascirev.2014.09.033
Hölting, M., Bovolo, C. I., and Ernst, R. (2016). Facing complexity in tropical conservation: How reduced impact logging and climatic extremes affect beta diversity in tropical amphibian assemblages. Biotropica 48, 528–536. doi:10.1111/btp.12309
Hong, B., Gasse, F., Uchida, M., Hong, Y., Leng, X., Shibata, Y., et al. (2014). Increasing summer rainfall in arid eastern-Central Asia over the past 8500 years. Sci. Rep. 4, 5279. doi:10.1038/srep05279
Hong, C., Zhang, Q., Zhang, Y., Davis, S. J., Tong, D., Zheng, Y., et al. (2019). Impacts of climate change on future air quality and human health in China. Proc. Natl. Acad. Sci. U. S. A. 116, 17193–17200. doi:10.1073/pnas.1812881116
Huang, C. C., Pang, J., Zhou, Y., Su, H., Zhang, Y., and Wang, L. (2013). Palaeoenvironmental implications of the prehistorical catastrophes in relation to the lajia ruins within the guanting basin along the upper yellow river, China. Holocene 23, 1584–1595. doi:10.1177/0959683613499052
Huguet, F., Parey, S., Dacunha-Castelle, D., and Malek, F. (2008). Is there a trend in extremely high river temperature for the next decades? A case study for France. Nat. Hazards Earth Syst. Sci. 8, 67–79. doi:10.5194/nhess-8-67-2008
Hulme, M. (2014). Attributing weather extremes to ‘climate change. Prog. Phys. Geogr. 38, 499–511. doi:10.1177/0309133314538644
Innes, J. B., Zong, Y., Wang, Z., and Chen, Z. (2014). Climatic and palaeoecological changes during the mid- to late Holocene transition in eastern China: High-resolution pollen and non-pollen palynomorph analysis at pingwang, yangtze coastal lowlands. Quat. Sci. Rev. 99, 164–175. doi:10.1016/j.quascirev.2014.06.013
Jegasothy, E., McGuire, R., Nairn, J., Fawcett, R., and Scalley, B. (2017). Extreme climatic conditions and health service utilisation across rural and metropolitan New South Wales. Int. J. Biometeorol. 61, 1359–1370. doi:10.1007/s00484-017-1313-5
Kalkstein, L. S., Greene, S., Mills, D. M., and Samenow, J. (2011). An evaluation of the progress in reducing heat-related human mortality in major U.S. cities. Nat. Hazards 56, 113–129. doi:10.1007/s11069-010-9552-3
Kosatsky, T. (2005). The 2003 European heat waves. Euro Surveill. 10, 3–4. doi:10.2807/esm.10.07.00552-en
Kraas, F. (2008). “Megacities as global risk areas,” in Urban ecology: An international perspective on the interaction between humans and nature. J. M. Marzluff, E. Shulenberger, W. Endlicher, M. Alberti, G. Bradley, and C. Ryan. Editors (Boston, MA: Springer US), 583–596. doi:10.1007/978-0-387-73412-5_38
Lara, R. J., Neogi, S. B., Islam, M. S., Mahmud, Z. H., Yamasaki, S., and Nair, G. B. (2009). Influence of catastrophic climatic events and human waste on Vibrio distribution in the Karnaphuli estuary, Bangladesh. Ecohealth 6, 279–286. doi:10.1007/s10393-009-0257-6
Lehnertz, K. (2006). “Epilepsy: Extreme events in the human brain,” in Extreme events in nature and society. Editors S. Albeverio, V. Jentsch, and H. Kantz (Berlin, Heidelberg: Springer Berlin Heidelberg), 123–143. doi:10.1007/3-540-28611-X_6
Lenton, T. M., Held, H., Kriegler, E., Hall, J. W., Lucht, W., Rahmstorf, S., et al. (2008). Tipping elements in the Earth’s climate system. Proc. Natl. Acad. Sci. U. S. A. 105, 1786–1793. doi:10.1073/pnas.0705414105
Li, B., Zhu, C., Wu, L., Li, F., Sun, W., Wang, X., et al. (2013). Relationship between environmental change and human activities in the period of the Shijiahe culture, Tanjialing site, Jianghan Plain, China. Quat. Int. 309, 45–52. doi:10.1016/j.quaint.2013.05.041
Li, P., Yin, W., Li, P., Li, X., Zhang, C., Stagnitti, F., et al. (2010). Distribution and migration of nitrobenzene in water following a simulated spill. J. Hazard. Mat. 182, 787–791. doi:10.1016/j.jhazmat.2010.06.105
Li, T., Horton, R. M., Bader, D. A., Zhou, M., Liang, X., Ban, J., et al. (2016). Aging will amplify the heat-related mortality risk under a changing climate: Projection for the elderly in beijing, China. Sci. Rep. 6, 28161. doi:10.1038/srep28161
Longin, F. M. (2000). From value at risk to stress testing: The extreme value approach. J. Bank. Finance 24, 1097–1130. doi:10.1016/S0378-4266(99)00077-1
Lu, X., Wrathall, D. J., Sundsøy, P. R., NadiruzzamanWetter, E., and Iqbal, A. (2016). Unveiling hidden migration and mobility patterns in climate stressed regions: A longitudinal study of six million anonymous mobile phone users in Bangladesh. Glob. Environ. Change 38, 1–7. doi:10.1016/j.gloenvcha.2016.02.002
Madsen, D. B., Chen, F.-H., and Gao, X. (2007). Changing views of Late Quaternary human adaptation in arid China. Dev. Quat. Sci. 9, 227–232. doi:10.1016/S1571-0866(07)09014-8
Margolis, H. G. (2014). “Heat waves and rising temperatures: Human health impacts and the determinants of vulnerability,” in Global climate change and public health. Editors K. E. Pinkerton, and W. N. Rom (New York, NY: Humana), 85–120. doi:10.1007/978-1-4614-8417-210.1007/978-1-4614-8417-2_6
Marotzke, J. (2000). Abrupt climate change and thermohaline circulation: Mechanisms and predictability. Proc. Natl. Acad. Sci. U. S. A. 97, 1347–1350. doi:10.1073/pnas.97.4.1347
Marriner, N., Flaux, C., Morhange, C., and Stanley, J.-D. (2013). Tracking nile delta vulnerability to Holocene change. PLoS One 8, e69195. doi:10.1371/journal.pone.0069195
IPCC (2021). Climate change 2021: The physical science basis. Contribution of working group I to the sixth assessment report of the intergovernmental panel on climate change. V. Masson-Delmotte, P. Zhai, A. Pirani, S. L. Connors, C. Péan, and S. Berger Editors (Cambridge: Cambridge University Press).
Matthewman, S. (2016). Disasters, risks and revelation: Making sense of our times. Hampshire: Palgrave Macmillan.
Matthews, T. K. R., Wilby, R. L., and Murphy, C. (2017). Communicating the deadly consequences of global warming for human heat stress. Proc. Natl. Acad. Sci. U. S. A. 114, 3861–3866. doi:10.1073/pnas.1617526114
Mayewski, P. A., Sneed, S. B., Birkel, S. D., Kurbatov, A. V., and Maasch, K. A. (2014). Holocene warming marked by abrupt onset of longer summers and reduced storm frequency around Greenland. J. Quat. Sci. 29, 99–104. doi:10.1002/jqs.2684
McPhillips, L. E., Chang, H., Chester, M. V., Depietri, Y., Friedman, E., Grimm, N. B., et al. (2018). Defining extreme events: A cross‐disciplinary review. Earths Future 6, 441–455. doi:10.1002/2017ef000686
Messerli, B., Grosjean, M., Hofer, T., Núñez, L., and Pfister, C. (2000). From nature-dominated to human-dominated environmental changes. Quat. Sci. Rev. 19, 459–479. doi:10.1016/S0277-3791(99)00075-X
Miao, Y., Zhang, D., Cai, X., Li, F., Jin, H., Wang, Y., et al. (2017). Holocene fire on the northeast Tibetan Plateau in relation to climate change and human activity. Quat. Int. 443, 124–131. doi:10.1016/j.quaint.2016.05.029
Middleton, N., Tozer, P., and Tozer, B. (2019). Sand and dust storms: Underrated natural hazards. Disasters 43, 390–409. doi:10.1111/disa.12320
Modarres, R., Ghadami, M., Naderi, S., and Naderi, M. (2018). Future heat stress arising from climate change on Iran’s population health. Int. J. Biometeorol. 62, 1275–1281. doi:10.1007/s00484-018-1532-4
Modarres, R. (2007). Streamflow drought time series forecasting. Stoch. Environ. Res. Risk Assess. 21, 223–233. doi:10.1007/s00477-006-0058-1
Moher, D., Liberati, A., Tetzlaff, J., and Altman, D. G.PRISMA Group (2009). Preferred reporting items for systematic reviews and meta-analyses: The PRISMA statement. PLoS Med. 6, e1000097. doi:10.1371/journal.pmed.1000097
Moreno, P. I., Simi, E., Villa-Martínez, R. P., and Vilanova, I. (2019). Early arboreal colonization, postglacial resilience of deciduous Nothofagus forests, and the Southern Westerly Wind influence in central-east Andean Patagonia. Quat. Sci. Rev. 218, 61–74. doi:10.1016/j.quascirev.2019.06.004
Nel, J. L., Le Maitre, D. C., Nel, D. C., Reyers, B., Archibald, S., van Wilgen, B. W., et al. (2014). Natural hazards in a changing world: A case for ecosystem-based management. PLoS One 9, e95942. doi:10.1371/journal.pone.0095942
Nicholson, T. E., Mayer, K. A., Staedler, M. M., and Johnson, A. B. (2007). Effects of rearing methods on survival of released free-ranging juvenile southern sea otters. Biol. Conserv. 138, 313–320. doi:10.1016/j.biocon.2007.04.026
Oliveira, J. P., Sousa-Pinto, I., Weber, G. M., and Bertocci, I. (2014). Interplay of experimental harvesting and climate-related disturbance on benthic assemblages of rocky seashores. Mar. Ecol. Prog. Ser. 495, 131–142. doi:10.3354/meps10574
Pagan, A. R., and Sossounov, K. A. (2003). A simple framework for analysing bull and bear markets. J. Appl. Econ. 18, 23–46. doi:10.1002/jae.664
Panin, A. V., Fuzeina, J. N., and Belyaev, V. R. (2009). Long-term development of Holocene and Pleistocene gullies in the protva river basin, central Russia. Geomorphology 108, 71–91. doi:10.1016/j.geomorph.2008.06.017
Parnell, A. C., Sweeney, J., Doan, T. K., Salter-Townshend, M., Allen, J. R. M., Huntley, B., et al. (2015). Bayesian inference for palaeoclimate with time uncertainty and stochastic volatility. J. R. Stat. Soc. Ser. C Appl. Stat. 64, 115–138. doi:10.1111/rssc.12065
Paulette, T. (2012). “Domination and resilience in bronze age mesopotamia,” in Surviving sudden environmental change. Editors J. Cooper, and P. Sheets (Boulder: University Press of Colorado), 167–196.
Pawson, E. (2011). Environmental hazards and natural disasters. N. Z. Geog. 67, 143–147. doi:10.1111/j.1745-7939.2011.01207.x
Pedersen, D., Tremblay, J., Errázuriz, C., and Gamarra, J. (2008). The sequelae of political violence: Assessing trauma, suffering and dislocation in the Peruvian highlands. Soc. Sci. Med. 67, 205–217. doi:10.1016/j.socscimed.2008.03.040
Pettay, D. T., Wham, D. C., Smith, R. T., Iglesias-Prieto, R., and LaJeunesse, T. C. (2015). Microbial invasion of the Caribbean by an Indo-Pacific coral zooxanthella. Proc. Natl. Acad. Sci. U. S. A. 112, 7513–7518. doi:10.1073/pnas.1502283112
Pistocchi, A., Calzolari, C., Malucelli, F., and Ungaro, F. (2015). Soil sealing and flood risks in the plains of Emilia-Romagna, Italy. J. Hydrology Regional Stud. 4, 398–409. doi:10.1016/j.ejrh.2015.06.021
Plumlee, G. S., Alpers, C. N., Morman, S. A., and Juan, C. S. (2016). Anticipating environmental and environmental-health implications of extreme storms: ARkStorm scenario. Nat. Hazards Rev. 17, A4015003. doi:10.1061/(asce)nh.1527-6996.0000188
IPCC (2022). in Climate change 2022: Impacts, adaptation, and vulnerability. Contribution of working group II to the sixth assessment report of the intergovernmental panel on climate change. H.-O. Pörtner, D. C. Roberts, M. Tignor, E. S. Poloczanska, K. Mintenbeck, and A. Alegría. Editors (Cambridge: Cambridge University Press).
R Core Team (2013). R: A language and environment for statistical computing. Vienna: R Foundation for Statistical Comuting. Available at: https://www.R-project.org/
Räsänen, T. A., Lindgren, V., Guillaume, J. H. A., Buckley, B. M., and Kummu, M. (2016). On the spatial and temporal variability of ENSO precipitation and drought teleconnection in mainland Southeast Asia. Clim. Past. 12, 1889. doi:10.5194/cp-12-1889-2016
Raup, D. M., and Sepkoski, J. J. (1982). Mass extinctions in the marine fossil record. Science 215, 1501–1503. doi:10.1126/science.215.4539.1501
Raymond, C., Horton, R. M., Zscheischler, J., Martius, O., AghaKouchak, A., Balch, J., et al. (2020). Understanding and managing connected extreme events. Nat. Clim. Chang. 10, 611–621. doi:10.1038/s41558-020-0790-4
Riede, F. (2014). Towards a science of past disasters. Nat. Hazards 71, 335–362. doi:10.1007/s11069-013-0913-6
Rohli, R. V., Bushra, N., Lam, N. S. N., Zou, L., Mihunov, V., Reams, M. A., et al. (2016). Drought indices as drought predictors in the south-central USA. Nat. Hazards 83, 1567–1582. doi:10.1007/s11069-016-2376-z
Sarewitz, D., and Pielke, R. (2001). Extreme events: A research and policy framework for disasters in context. Int. Geol. Rev. 43, 406–418. doi:10.1080/00206810109465022
Scheffer, M. (2020). Critical transitions in nature and society. Princeton: Princeton University Press.
Schneider, S. H., and Root, T. L. (1996). Ecological implications of climate change will include surprises. Biodivers. Conservation 5, 1109–1119. doi:10.1007/BF00052720
Sena, A., Barcellos, C., Freitas, C., and Corvalan, C. (2014). Managing the health impacts of drought in Brazil. Int. J. Environ. Res. Public Health 11, 10737–10751. doi:10.3390/ijerph111010737
Shepherd, T. G. (2016). A common framework for approaches to extreme event attribution. Curr. Clim. Change Rep. 2, 28–38. doi:10.1007/s40641-016-0033-y
Singh, D., Ghosh, S., Roxy, M. K., and McDermid, S. (2019). Indian summer monsoon: Extreme events, historical changes, and role of anthropogenic forcings. Wiley Interdiscip. Rev. Clim. Change 10, e571. doi:10.1002/wcc.571
Smid, M., Russo, S., Costa, A. C., Granell, C., and Pebesma, E. (2019). Ranking European capitals by exposure to heat waves and cold waves. Urban Clim. 27, 388–402. doi:10.1016/j.uclim.2018.12.010
Smith, E. T., and Sheridan, S. C. (2019). The influence of extreme cold events on mortality in the United States. Sci. Total Environ. 647, 342–351. doi:10.1016/j.scitotenv.2018.07.466
Smith, M. D. (2011). An ecological perspective on extreme climatic events: A synthetic definition and framework to guide future research. J. Ecol. 99, 656–663. doi:10.1111/j.1365-2745.2011.01798.x
Smith, M. E. (2021). Archaeology, relevance and science. Antiquity 95, 1085–1087. doi:10.15184/aqy.2021.91
Smith, W., and Dressler, W. (2019). Governing vulnerability: The biopolitics of conservation and climate in upland Southeast Asia. Polit. Geogr. 72, 76–86. doi:10.1016/j.polgeo.2019.04.004
Stott, P. A., Christidis, N., Otto, F. E. L., Sun, Y., Vanderlinden, J.-P., van Oldenborgh, G. J., et al. (2016). Attribution of extreme weather and climate-related events. Wiley Interdiscip. Rev. Clim. Change 7, 23–41. doi:10.1002/wcc.380
Stott, P. A., Stone, D. A., and Allen, M. R. (2004). Human contribution to the European heatwave of 2003. Nature 432, 610–614. doi:10.1038/nature03089
Strogatz, S. H. (2018). Nonlinear dynamics and chaos: With applications to physics, biology, chemistry, and engineering. Boca Raton: CRC Press.
Sun, Q., Miao, C., Hanel, M., Borthwick, A. G. L., Duan, Q., Ji, D., et al. (2019). Global heat stress on health, wildfires, and agricultural crops under different levels of climate warming. Environ. Int. 128, 125–136. doi:10.1016/j.envint.2019.04.025
Sung, T.-I., Wu, P.-C., Lung, S.-C., Lin, C.-Y., Chen, M.-J., and Su, H.-J. (2013). Relationship between heat index and mortality of 6 major cities in Taiwan. Sci. Total Environ. 442, 275–281. doi:10.1016/j.scitotenv.2012.09.068
Tessler, Z. D., Vörösmarty, C. J., and Grossberg, M. (2015). Profiling risk and sustainability in coastal deltas of the world. Science 349, 638–643. doi:10.1126/science.aab3574
Thieken, A. H., Bessel, T., Kienzler, S., Kreibich, H., Müller, M., Pisi, S., et al. (2016). The flood of june 2013 in Germany: How much do we know about its impacts? Nat. Hazards Earth Syst. Sci. 16, 1519–1540. doi:10.5194/nhess-16-1519-2016
van Oldenborgh, G. J., van der Wiel, K., Kew, S., Philip, S., Otto, F., Vautard, R., et al. (2021). Pathways and pitfalls in extreme event attribution. Clim. Change 166, 13. doi:10.1007/s10584-021-03071-7
Vanos, J. K., Cakmak, S., Kalkstein, L. S., and Yagouti, A. (2015). Association of weather and air pollution interactions on daily mortality in 12 Canadian cities. Air Qual. Atmos. Health 8, 307–320. doi:10.1007/s11869-014-0266-7
Viglizzo, E. F., and Frank, F. C. (2006). Ecological interactions, feedbacks, thresholds and collapses in the Argentine Pampas in response to climate and farming during the last century. Quat. Int. 158, 122–126. doi:10.1016/j.quaint.2006.05.022
Vött, A., Bruins, H., Goodman Tchernov, B., De Martini, P. M., Kelletat, D., Mastronuzzi, G., et al. (2019). Publicity waves based on manipulated geoscientific data suggesting climatic trigger for majority of tsunami findings in the mediterranean—response to ‘tsunamis in the geological record: Making waves with a cautionary tale from the mediterranean’ by marriner et al. Z. für Geomorphol. 62, 7–45. doi:10.1127/zfg_suppl/2018/0547
Wang, A.-J., Kawser, A., Xu, Y.-H., Ye, X., Rani, S., and Chen, K.-L. (2016). Heavy metal accumulation during the last 30 years in the Karnaphuli River estuary, Chittagong, Bangladesh. Springerplus 5, 2079. doi:10.1186/s40064-016-3749-1
Wang, J., Kuffer, M., Sliuzas, R., and Kohli, D. (2019). The exposure of slums to high temperature: Morphology-based local scale thermal patterns. Sci. Total Environ. 650, 1805–1817. doi:10.1016/j.scitotenv.2018.09.324
Wang, Q., and Su, M. (2020). A preliminary assessment of the impact of COVID-19 on environment - a case study of China. Sci. Total Environ. 728, 138915. doi:10.1016/j.scitotenv.2020.138915
Wang, X., Ding, Z., and Peng, P. (2012). Changes in fire regimes on the Chinese Loess Plateau since the last glacial maximum and implications for linkages to paleoclimate and past human activity. Palaeogeogr. Palaeoclimatol. Palaeoecol. 315–316, 61–74. doi:10.1016/j.palaeo.2011.11.008
Warnes, G. R., Bolker, B., Bonebakker, L., Gentleman, R., Huber, W., Liaw, A., et al. (2009). gplots: Various R programming tools for plotting data. R. package version 2, 1.
Wickham, H., Averick, M., Bryan, J., Chang, W., McGowan, L., François, R., et al. (2019). Welcome to the tidyverse. J. Open Source Softw. 4, 1686. doi:10.21105/joss.01686
Wiedemann, G., and Niekler, A. (2017). “Hands-on: A five day text mining course for humanists and social scientists in R,” in Proceedings of the 1st workshop teaching NLP for digital humanities (Berlin: Natural Language Processing Group). Available at: http://ceur-ws.org/Vol-1918/wiedemann.pdf.
Wu, Y., Bake, B., Zhang, J., and Rasulov, H. (2015). Spatio-temporal patterns of drought in North Xinjiang, China, 1961–2012 based on meteorological drought index. J. Arid. Land 7, 527–543. doi:10.1007/s40333-015-0125-x
Yamano, H., Kayanne, H., Yamaguchi, T., Kuwahara, Y., Yokoki, H., Shimazaki, H., et al. (2007). Atoll island vulnerability to flooding and inundation revealed by historical reconstruction: Fongafale Islet, Funafuti Atoll, Tuvalu. Glob. Planet. Change 57, 407–416. doi:10.1016/j.gloplacha.2007.02.007
Yeakel, J. D., Pires, M. M., Rudolf, L., Dominy, N. J., Koch, P. L., Guimarães, P. R., et al. (2014). Collapse of an ecological network in Ancient Egypt. Proc. Natl. Acad. Sci. U. S. A. 111, 14472–14477. doi:10.1073/pnas.1408471111
Keywords: climate change, natural hazard, human health, prehistory, vulnerability, risk, resilience, abrupt
Citation: Stewart M, Carleton WC and Groucutt HS (2022) Extreme events in biological, societal, and earth sciences: A systematic review of the literature. Front. Earth Sci. 10:786829. doi: 10.3389/feart.2022.786829
Received: 30 September 2021; Accepted: 25 July 2022;
Published: 24 August 2022.
Edited by:
Carmen De Jong, Université de Strasbourg, FranceReviewed by:
Michelle Simoes Reboita, Federal University of Itajubá, BrazilMichael Evans, University of Maryland, College Park, United States
Copyright © 2022 Stewart, Carleton and Groucutt. This is an open-access article distributed under the terms of the Creative Commons Attribution License (CC BY). The use, distribution or reproduction in other forums is permitted, provided the original author(s) and the copyright owner(s) are credited and that the original publication in this journal is cited, in accordance with accepted academic practice. No use, distribution or reproduction is permitted which does not comply with these terms.
*Correspondence: Mathew Stewart, bXN0ZXdhcnRAaWNlLm1wZy5kZQ==
†These authors have contributed equally to this work