- Department of Agricultural and Forestry Science and Technology, Chongqing Three Gorges Vocational College, Chongqing, China
Decadally cycling soil carbon (dSOC) is the main component of the terrestrial soil carbon (C) pool. The response of dSOC to warming largely determines the feedback between climate warming and the C cycle. However, there is a lack of investigations about the effect of warming on the relative change in turnover rate (RCT) of dSOC and annually cycling SOC (aSOC) in dissolved organic carbon (DOC), microbial biomass carbon (MBC) and CO2. We clarified this issue by incubating two C3-C4 vegetation switch soils (23 years switch, HA soil and 55 years switch, GG soil) at 20°C and 30°C in the recently improved continuous airflow CO2 trapping system for 1 year. Warming increased the contribution of dSOC (C3-C) by 21℅ (soil HA) and 8℅ (soil GG) in MBC, and 38℅ (soil HA) and 15℅ (soil GG) in CO2, while only 2%–3℅ increase in DOC at the final stage of the incubation. Furthermore, warming increased the RCT in MBC and CO2 by 5.3- and 4.1-fold, respectively, but had no significant influence on the RCT in DOC, indicating that soil microbes may be an important engine to accelerate dSOC-derived CO2 emission in a warming world.
Introduction
Soil is the largest organic carbon (C) pool in terrestrial ecosystems, and thus even a small variation in soil C stock can significantly change atmospheric CO2 concentration (Batjes, 1996; Kirschbaum, 2006). The increase in CO2 can cause a series of global climate and ecosystem changes, including global warming (Hausfather et al., 2022) and plant photosynthesis increases (Keenan et al., 2021; Kumar et al., 2021). Most studies presume that climate warming will accelerate soil organic carbon (SOC) mineralization and increase CO2 efflux and thus further exacerbate climate warming, forming positive feedback (Melillo et al., 2002; Giardina et al., 2014). However, the intensity of this feedback may largely depend on the response of soil C pools with different turnover rates to warming.
According to the turnover rate, the soil C stocks can be conveniently divided into annually cycling carbon (aSOC), decadally cycling carbon (dSOC), and millennially cycling carbon components (mSOC) (Davidson and Janssens, 2006). The aSOC is relatively labile and has a much small size (only 0%–5%). The mSOC would be beyond our concerns with the turnover time of a few centuries and much stronger stability (Dungait et al., 2012). The dSOC with turnover times of 10–100 years is the main component of SOC stocks (60%–85%), and its response to global change will significantly alter the global C cycle (Jones et al., 2005). Therefore, it is crucial to better understand the response of dSOC to warming (Conant et al., 2011).
The response of soil C turnover to warming might depend on the chemical recalcitrance and physical accessibility of microbial substrates, and the change of soil microbial community activity/composition and extracellular enzymes (Schimel and Schaeffer, 2012; Xu et al., 2018). Relevant observations have shown that 14 years-warming caused shifts in soil microbial community activity/composition and thus accelerated the turnover of labile, but not recalcitrant organic C in a tallgrass prairie soil (Stuble et al., 2019). Microbial community influences on SOC turnover in mineral soils are based on physical access to the occluded or sorbed substrates, and how organisms allocate the C they take up (Xu et al., 2018). Soil C pools containing less labile material have a longer turnover time with higher activation energy, and thus are more temperature-sensitive as projected by a three-pool model (Knorr et al., 2005). However, the underlying mechanisms driving this process are still unclear.
There are two main methods to assess the response of soil C pools to climate warming. Firstly, based on soil respiration rates from laboratory incubation and modeling (Paul et al., 2001; Paterson et al., 2009; Jagadamma et al., 2014; Jiang et al., 2018). Secondly, according to 13C isotopic tracer (Martin et al., 1990; Roscoe et al., 2001; Blagodatskaya et al., 2011) or radiocarbon (14C) dating measurement and modeling (O’Brien et al., 2013; Han et al., 2017; Hall et al., 2018).
In this study, we determined the effect of warming on SOC decomposition via the assumed pathway of SOC → dissolved organic carbon (DOC) → microbial biomass carbon (MBC) → CO2 by using the natural 13C isotopic tracing method. The relative change in turnover rate (RCT) of dSOC increased while aSOC decreased in DOC, MBC and CO2 were assessed in a 1-year incubation experiment using two C3-C4 vegetation switch soils at 20°C and 30°C. In this study, we hypothesized that 1) warming increased the contribution of dSOC to DOC, MBC and CO2; and 2) warming increased the RCT in MBC and CO2.
Materials and methods
Soil used
Two C3-C4 vegetation switch soils were sampled from the plow layer (0–20 cm). One soil was obtained from the experimental station of Heilongjiang Academy of Agricultural Sciences, Harbin, in the northeast of China (HA soil), where C4-maize (δ13C = −13.9‰) was planted for 23 years after mixed C3 grass causing a shift in the δ13C of SOC from −25.6‰ to −20.4‰ in HA soil [Corg = 17.0 g kg−1; Ntotal = 1.4 g kg−1; pH (CaCl2) = 6.7]. The other soil was obtained from the agricultural region of Guigang in southwest China (GG soil), where C4- sugarcane (δ13C= −13.0‰) was grown for 55 years on a C3 paddy field shifting the δ13C of SOC from −27.9‰ to −15.4‰ in GG soil [Corg = 22.6 g kg−1; Ntotal = 2.0 g kg−1; pH (CaCl2) = 6.9]. These differences in δ13C were used to distinguish dSOC (C3 signal) and aSOC (C4 signal).
Experimental design and soil incubation
The 1-year laboratory incubation experiment on air-dried, root-free soils was executed. The procedures in detail are given in (Lin et al., 2015). Specifically, each soil was screened by 2 mm, mixed evenly, air-dried in the field, and brought back to the laboratory. The plant roots and visible gravel were carefully removed before incubation. 300 g soil was put into a polypropylene column with a diameter of 5 cm and a height of 25 cm. The bottom of the column was plugged with a silicone plug with a hose for ventilation, and the top was covered with a parafilm film with a small hole for water retention and ventilation. Each column was placed in an incubator with an accuracy of ±0.2°C (SHELLAB LI20-2, United States) at 20°C and 30°C. Before the experiment, soil moisture was adjusted to 60% of the maximum soil moisture holding capacity (WHC) by adding deionized water. Soil moisture was kept constant by weighing during the 360-day incubation period. To avoid anaerobic conditions, each column was vented for 1 h each day with CO2-free air.
Soil respiration and δ13C-CO2
An improved continuous air-flow CO2 trapping system was used for soil respiration rate and δ13C-CO2 measurement (Virginia, 1993; Lin et al., 2015). Soil respiration and δ13C-CO2 were measured at 14, 45, 60, 120, 180, 240, 320, and 360 d. Soil respiration was measured by using an infrared CO2 analyzer (LiCOR 6262, Lincoln, NB, United Stat) coupled with a digital mass flow meter (GFM17, Aalborg Instruments and Control Inc., New York, United States). The δ13C-CO2 was measured by analyzing NaOH solution by Cavity Ring-Down Spectroscopy (CRDS) with Automate Module (Picarro G2131-i Analyzer, United States) after CO2 trapping. Blanks without soil were included as a reference to correct handling errors.
DOC, MBC, SOC, and δ13C
The content and δ13C of DOC and MBC were measured at 14, 45, 60, 120, 180, 240, 320, and 360 d in HA soil and at 14, 45, 320, and 360 d in GG soil, respectively. The content and δ13C of SOC were measured at 14, 320, and 360 d in soil HA and soil GG. DOC from 50 g soil was extracted with 0.5 M K2SO4 in a 1:2 ratio. MBC from another 50 g of soil was fumigated by chloroform and then extracted in the same way. The conversion factor of Kc is 0.38 for MBC (Vance et al., 1987). The extracts were determined by a TOC/TN analyzer (Multi N/C 3100, Analytik Jena, Germany). An aliquot of 20 ml K2SO4 extract was measured for the δ13C by Picarro iTOC-CRDS analyzer (Picarro Inc., Santa Clara, CA, United States). In brief, inorganic C in the extracts was removed by 5% phosphoric acid at 70°C, and then organic C in the extract was converted into CO2 by 10% Na2S2O8 at 98°C. SOC was analyzed by dry combustion using an Elementar analyzer (Vario ELⅢ, elementar, Germany). The δ13C of SOC was determined by WS-CRDS (Picarro G2131-i Analyzer, Picarro Inc., Santa Clara, CA, United States).
Calculations and statistical analysis
In order to calculate the respiration rate of the soil samples, we used the formula (Tian et al., 2014; Lin et al., 2015):
where R is soil CO2 efflux (µg CO2-C g−1 dry soil day−1), C is the recorded CO2 concentration (µmol CO2 mol−1) in the sample jar, C0 is the recorded CO2 concentration in the blank jar, v is the recorded CO2 flow rate by digital mass flow meter (ml min−1), 22.4 is the molar volume of gas under standard conditions (L/mol), and W is gram dry weight of the soil sample.
The portion (F) of aSOC (maize or sugar cane-derived C) in DOC, MBC, SOC, and CO2 was estimated by the following equation (Amelung et al., 2008):
where δ13Ct is the δ13C value of the C pools (SOC, DOC, MBC) and CO2-C under maize or sugar cane; δ13C3 is the δ13C value of SOC in reference soil with continuous C3 vegetation (Lin et al., 2015). The δ13C of DOC, MBC and CO2-C in reference soil were calculated according to the δ13C shift between SOC and the C pools (Blagodatskaya et al., 2011). The δ13C4 was calculated based on the δ13C value of maize or sugar cane (mean of leaves, stems and roots) and corrected by subtracting the difference between δ13C of SOC in C3 reference soil and δ13C of corresponding C3 plant (i.e., rice, wheat or fescue) by assuming similar isotopic fractionation from C3 and C4 plants in humification processes (Schneckenberger and Kuzyakov, 2007).
where Rs is soil respiration rate; MBC is microbial biomass C; Y (microbial substrate utilization efficiency) is 0.45; Rm (soil microbial maintenance respiration rate) is 0.08% of the biomass day−1.
Based on the 13C shift, the relative change in the turnover rate (RCT) of dSOC increased while aSOC decreased in DOC, MBC and CO2 was calculated as follows (Blagodatskaya et al., 2011):
where C3-C is C3-derived C in DOC, MBC or CO2; C4-C is C4-derived C in DOC, MBC or CO2. “Final” represents the stage of the last 45 d during 1-year incubation. “Initial” represents the stage of the first 45d from the beginning of 1-year incubation.
Statistical analyses for all data were performed using SPSS Statistics 20. The Fischer LSD test was used for mean comparisons at p<0.05. Curve fitting was performed using SigmaPlot12.5.
Results
Dynamics of δ13C in CO2-C, MBC and DOC
The δ13CCO2 continuously depleted with time prolonged and warming, suggesting the C source for microbial respiration increasingly transformed from aSOC to dSOC (Figure 1). The δ13CMBC firstly increased at the initial stage, and then continuously decreased and was stable at the final stage for both soils at 20°C and 30°C, indicating that a relative contribution switched from aSOC to dSOC for microbial assimilation with the incubation time prolonged. On the contrary, the δ13CDOC and δ13CSOC were almost constant throughout the whole incubation and warming, indicating an equal contribution of dSOC and aSOC to DOC.
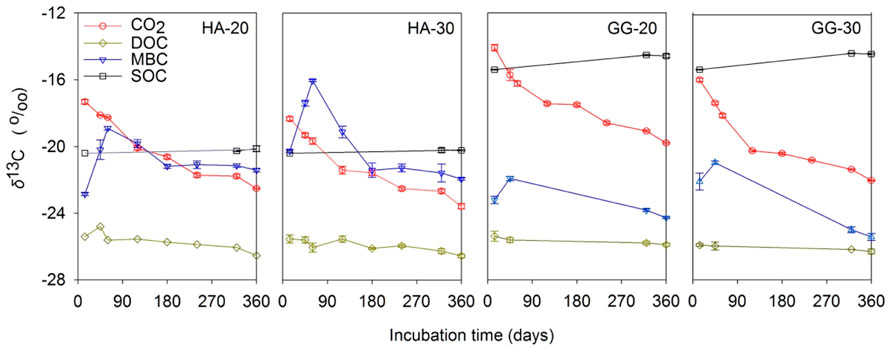
FIGURE 1. The effect of temperature on the δ13C dynamics in C pools during incubation of the soil after C3-C4 vegetation change. Bars indicate standard errors (SE, n = 3).
Contribution of dSOC to CO2-C, MBC and DOC under warming
At the initial stage (first 45 d) of the incubation, warming increased the C3/Ctotal by 3℅ (soil HA) and 7℅ (soil GG) in MBC, and 9℅ (soil HA) and 12℅ (soil GG) in CO2, while had a 1%–3℅ increase on C3/Ctotal in DOC. Furthermore, warming increased the C3/Ctotal by 21℅ (soil HA) and 8℅ (soil GG) in MBC, and 38℅ (soil HA) and 15℅ (soil GG) in CO2, while having a slight increase in C3/Ctotal in DOC by 2%–3℅ at the final stage of the incubation (Table 1).
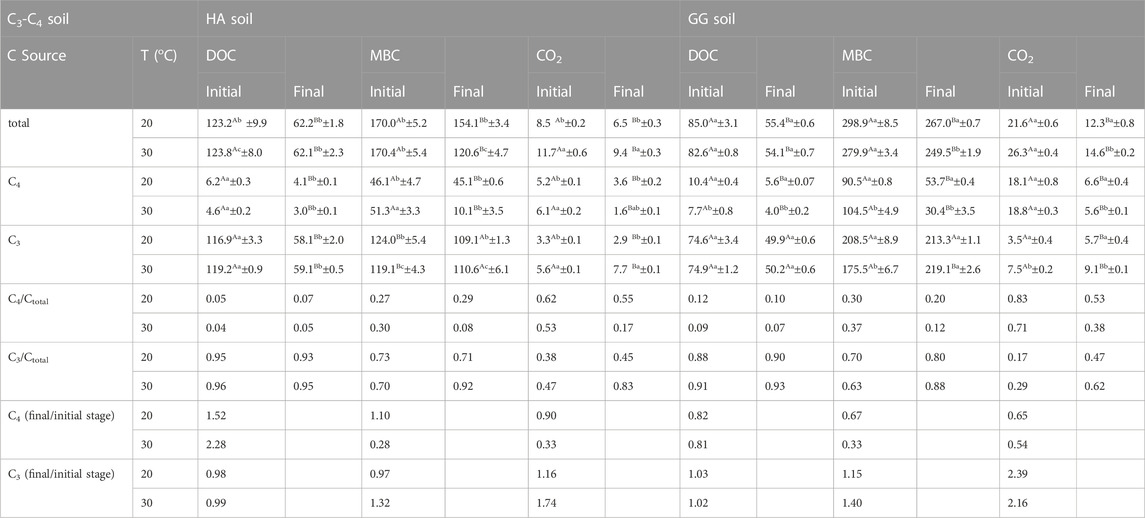
TABLE 1. The contribution of aSOC (C4) and dSOC (C3) to CO2-C (μg CO2-C g−1 soil d−1), MBC (μg C g−1 soil) and DOC (μg C g−1 soil) at the initial 45d and the final 45d stages of incubation. Values are means ± standard errors (n = 3). Values with different lowercase letters in each column and capital letters in each row are significantly different (p < 0.05). T: incubation temperature (oC).
Relative change in turnover rate
Relative change in turnover rate (RCT) in MBC and CO2 significantly increased with temperature increasing for both soils. However, the RCT in DOC was not significantly different between 20°C and 30°C in soil HA and GG, respectively (Figure 2).
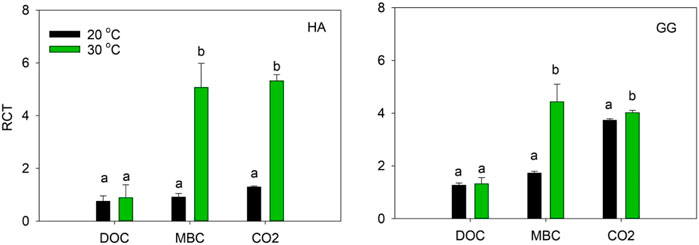
FIGURE 2. RCT variation after 1-year incubation at 20 C and 30 C. RCT is an indicator as the relative change of contribution of C3-C in DOC, MBC, and CO2-C increased while that of C4-C decreased during the incubation. Different lowercase letters denote significant differences at p < 0.05 level between 20°C and 30 C.
δ13C shift in the direction of SOC→DOC →MBC→CO2
Warming simultaneously depleted the δ13C of SOC→DOC and MBC→CO2 for soil HA and GG at the initial and final stages (Figure 3). However, warming enriched the δ13C of DOC→MBC at the initial stage, while depleting the δ13C of DOC→MBC at the final stage for soil HA and GG. The change of the δ13C in DOC→MBC further verified that the microbial substrate source transforms from aSOC to dSOC with warming and incubation time.
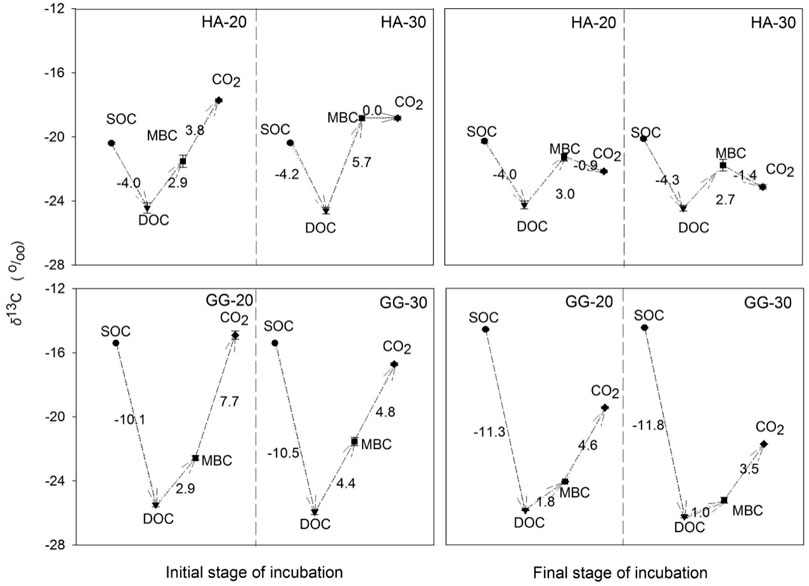
FIGURE 3. Changes in the δ13C signature of C pools during the 1-year incubation. Values are presented based on δ13C of C pools in the soil after C3-C4 vegetation change. We assumed that the transformations go in the following direction: SOC→DOC→MBC→CO2. Error bars show ±SE (n = 3).
Discussion
Microbial substrates gradually switched from aSOC to dSOC with warming and prolonged time. Under a sufficient supply of available substrates, aSOC-derived substrates were immediately preferentially utilized and decomposed by microorganisms metabolically for CO2 release (Esperschütz et al., 2009; Vain et al., 2021). Under the warming, aSOC was further utilized by microorganisms for soil respiration and microbial assimilation. The δ13C was different in soil C pools and their metabolites (Figure 1). The δ13C pathway of DOC→MBC was prolonged, and the absolute δ13C values were enriched by 1.5%–2.6‰ across soil HA and soil GG after warming at the initial stage (left scheme on Figure 3), which further verified that microorganisms selectively utilize more available aSOC that mainly originated from C4-C, i.e., recent C from C3-C4 vegetation switch, such as root exudate fractions from DOC at the initial warming stage. On the contrary, compared with the initial stage, the δ13C pathway of DOC→MBC shortened with warming and incubation time at the final stage, and the absolute values of δ13C were depleted by 0.3%–0.8‰ across the two soils (right scheme Figure 3). This indicated a decreased microbial uptake of aSOC from DOC, so that more dSOC was assigned to microbial growth and respiration, which enhanced the contribution of dSOC to MBC and CO2 with incubation prolonged and warming (Table 1).
The RCT is an indicator of the relative change in the turnover rate of dSOC (C3-C) increased when aSOC (C4-C) decreased, which is more indicative here as the relative contribution of dSOC in MBC or CO2 increased while those of aSOC decreased during the 360-d incubation (Blagodatskaya et al., 2011). The RCT in MBC and CO2 were much higher at the higher temperature in Figure 2, which confirmed that warming accelerates the relative change in the turnover rate of dSOC-derived C in MBC and CO2. Nutrient availability impacts the response of SOC pools to warming by altering substrate stabilization, microbial community composition and extracellular enzyme activity (Doetterl et al., 2018). SOC turnover mostly involves several steps with distinct inherent kinetics, such as aggregate disruption and then exoenzymes breaking up the polymers (Liang and Balser, 2011; Poeplau et al., 2017). Microbial biomass is a small size and relatively labile C pool, and thus it turnovers much quicker relative to SOC. Microbial growth may primarily utilize the dead cells and dSOC under a shift of C substrate types with incubation time. Warming might intensify the decomposition of the dSOC by deactivating aggregate-binding (Poeplau et al., 2017). Once dSOC loses the physi-chemical protection of soil aggregates, it will be exposed to microorganisms. Thus, microorganisms can quickly access dSOC and further improve the turnover of SOC at the final stage of the incubation. A meta-analysis showed that C-degradation-related enzyme activities differentially respond to warming. Ligninase activity and turnover of recalcitrant C pools were gradually enhanced with experiment duration and warming (Chen et al., 2018). Another relevant study found that a 14-year warming-induced change in the shift of soil microbial community activity and composition accelerated the turnover of labile, but not recalcitrant C pools (Stuble et al., 2019). Similarly, warming-induced losses of unprotected SOC were detected from 4 to 9 years of whole-ecosystem warming experiments in a grassland (Phillips et al., 2016).
The average microbial turnover time (MTT) was estimated according to the amount of CO2-C respired compared to the amount of MBC by assuming a steady state at the final stage of incubation (Eq. 3). It was observed that warming accelerated the turnover of microbial biomass by 1.6–2.6 and 1.5–2.0 times in dSOC- and aSOC-derived MBC, respectively (Figure 4). The MTT was estimated as 17.7–47.2 d and 6.6–15.4 d in dSOC- and aSOC-derived MBC across two soils and two temperatures in this study (Figure 4), which is lower than that of 64.5 d in dSOC and 20.7 d in aSOC reported in Blagodatskaya et al. (2011). Obviously, the sensitivity of dSOC- and aSOC-derived MBC turnover to warming is inconsistent. Furthermore, Li et al. (2019) found that warming increases microbial biomass turnover using a probabilistic inversion approach by integrating a microbial-enzyme model with two decades of soil warming measurement. However, the difference in temperature sensitivity of microbial turnover in soil C pools with different turnover rates (such as aSOC and dSOC) is not considered in the model predictions. It is crucial to accurately evaluate the prediction of soil C budget caused by warming. Thus, it should be focused on in the following research.
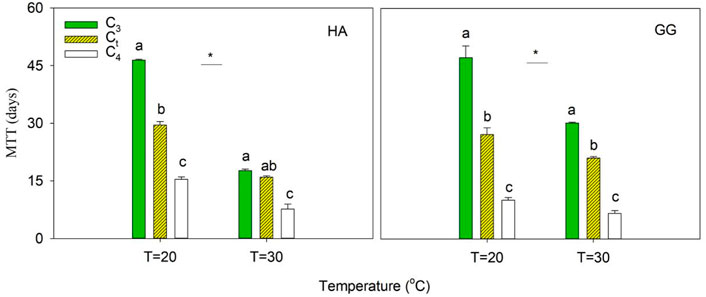
FIGURE 4. The microbial turnover time (MTT) of C3-derived, C4-derived, and total C (Ct). Error bars show ±SE (n = 3). Different lowercase letters denote significant differences at p < 0.05 level among the MTT of C3, C4, and Ct. Asterisk indicates a significant difference of C3, C4, and Ct at p<0.05 between T = 20 C and T = 30°C, respectively.
Conclusion
Based on a natural 13C isotopic tracer, the effect of warming on RCT of dSOC in DOC, MBC and CO2 was investigated by incubating two C3-C4 vegetation switch soils for 1 year. Warming increased the contribution of dSOC to total SOC by 8%–21℅ in MBC, and 15%–38℅ in CO2, while only 2%–3℅ in DOC. The RCT in MBC and CO2 increased by 5.3- and 4.1-fold due to warming, respectively, but had no significant variation in DOC, indicating that soil microbes may be an important engine to accelerate dSOC-derived CO2 emission in a warming world. The following research should focus on microbial regulation in the dSOC transformation process and temperature sensitivity of soil C pools with distinct turnover rates in MBC and CO2 for correctly projecting the feedback between climate warming and soil C storage.
Data availability statement
The raw data supporting the conclusion of this article will be made available by the authors, without undue reservation.
Author contributions
QN and DL designed the experiment; CX and DL performed the experiments; DL and WZ analyzed the data and wrote the manuscript; All authors revised the manuscript.
Funding
This work was supported by the Science and Technology Research Program of Chongqing Municipal Education Commission (KJZD-K202003501, KJZD-K202001203), the Program of Chongqing Science and Technology Commission (cstc2020jcyj-msxmX0095), and the Chongqing University Innovation Research Group Project (CXQTP19037).
Conflict of interest
The authors declare that the research was conducted in the absence of any commercial or financial relationships that could be construed as a potential conflict of interest.
Publisher’s note
All claims expressed in this article are solely those of the authors and do not necessarily represent those of their affiliated organizations, or those of the publisher, the editors and the reviewers. Any product that may be evaluated in this article, or claim that may be made by its manufacturer, is not guaranteed or endorsed by the publisher.
Abbreviations
aSOC, annually cycling soil organic carbon; C, carbon; DOC, dissolved organic carbon; dSOC, decadally cycling soil organic carbon; GG, soil obtained from Guigang after 55 years of C3-C4 vegetation switch; HA, soil obtained from Harbin after 23 years of C3-C4 vegetation switch; MBC, microbial biomass carbon; mSOC, millennially cycling carbon; MTT, microbial turnover time; RCT, relative change in turnover rate; SOC, soil organic carbon.
References
Amelung, W., Brodowski, S., Sandhage-Hofmann, A., and Bol, R. (2008). Advances in agronomy. Cambridge, Massachusetts, United States: Academic Press, 155–250.
Batjes, N. H. (1996). Total carbon and nitrogen in the soils of the world. Eur. J. Soil Sci. 47 (2), 151–163. doi:10.1111/j.1365-2389.1996.tb01386.x
Blagodatskaya, E., Yuyukina, T., Blagodatsky, S., and Kuzyakov, Y. (2011). Turnover of soil organic matter and of microbial biomass under C3–C4 vegetation change: Consideration of 13C fractionation and preferential substrate utilization. Soil Biol. Biochem. 43 (1), 159–166. doi:10.1016/j.soilbio.2010.09.028
Chen, J., Luo, Y., García-Palacios, P., Cao, J., Dacal, M., Zhou, X., et al. (2018). Differential responses of carbon-degrading enzyme activities to warming: Implications for soil respiration. Glob. Chang. Biol. 24 (10), 4816–4826. doi:10.1111/gcb.14394
Conant, R. T., Ryan, M. G., gren, G. I., Birge, H. E., Davidson, E. A., Eliasson, P. E., et al. (2011). Temperature and soil organic matter decomposition rates–synthesis of current knowledge and a way forward. Glob. Chang. Biol. 17 (11), 3392–3404. doi:10.1111/j.1365-2486.2011.02496.x
Doetterl, S., Berhe, A. A., Arnold, C., Bodé, S., Fiener, P., Finke, P., et al. (2018). Links among warming, carbon and microbial dynamics mediated by soil mineral weathering. Nat. Geosci. 11 (8), 589–593. doi:10.1038/s41561-018-0168-7
Dungait, J. A., Hopkins, D. W., Gregory, A. S., and Whitmore, A. P. (2012). Soil organic matter turnover is governed by accessibility not recalcitrance. Glob. Chang. Biol. 18 (6), 1781–1796. doi:10.1111/j.1365-2486.2012.02665.x
Esperschütz, J., Buegger, F., Winkler, J., Munch, J., Schloter, M., and Gattinger, A. (2009). Microbial response to exudates in the rhizosphere of young beech trees (Fagus sylvatica L.) after dormancy. Soil Biol. Biochem. 41 (9), 1976–1985. doi:10.1016/j.soilbio.2009.07.002
Giardina, C. P., Litton, C. M., Crow, S. E., and Asner, G. P. (2014). Warming-related increases in soil CO2 efflux are explained by increased below-ground carbon flux. Nat. Clim. Chang. 4 (9), 822–827. doi:10.1038/nclimate2322
Hall, S. J., Berhe, A. A., and Thompson, A. (2018). Order from disorder: Do soil organic matter composition and turnover co-vary with iron phase crystallinity? Biogeochemistry 140 (1), 93–110. doi:10.1007/s10533-018-0476-4
Han, C., Wang, Z., Si, G., Lei, T., Yuan, Y., and Zhang, G. (2017). Increased precipitation accelerates soil organic matter turnover associated with microbial community composition in topsoil of alpine grassland on the eastern Tibetan Plateau. Can. J. Microbiol. 63 (10), 811–821. doi:10.1139/cjm-2017-0157
Hausfather, Z., Marvel, K., Schmidt, G. A., Nielsen-Gammon, J. W., and Zelinka, M. (2022). Climate simulations: Recognize the ‘hot model’problem. Nature 605, 26–29. doi:10.1038/d41586-022-01192-2
Jagadamma, S., Steinweg, J. M., Mayes, M. A., Wang, G., and Post, W. M. (2014). Decomposition of added and native organic carbon from physically separated fractions of diverse soils. Biol. Fertil. Soils 50 (4), 613–621. doi:10.1007/s00374-013-0879-2
Jiang, Y., Qian, H., Wang, X., Chen, L., Liu, M., Li, H., et al. (2018). Nematodes and microbial community affect the sizes and turnover rates of organic carbon pools in soil aggregates. Soil Biol. Biochem. 119, 22–31. doi:10.1016/j.soilbio.2018.01.001
Jones, C., Mcconnell, C., Coleman, K., Cox, P., Falloon, P., Jenkinson, D., et al. (2005). Global climate change and soil carbon stocks; predictions from two contrasting models for the turnover of organic carbon in soil. Glob. Chang. Biol. 11 (1), 154–166. doi:10.1111/j.1365-2486.2004.00885.x
Keenan, T., Luo, X., De Kauwe, M., Medlyn, B., Prentice, I., Stocker, B., et al. (2021). A constraint on historic growth in global photosynthesis due to increasing CO2. Nature 600 (7888), 253–258. doi:10.1038/s41586-021-04096-9
Kirschbaum, M. U. F. (2006). The temperature dependence of organic-matter decomposition—Still a topic of debate. Soil Biol. Biochem. 38 (9), 2510–2518. doi:10.1016/j.soilbio.2006.01.030
Knorr, W., Prentice, I. C., House, J., and Holland, E. (2005). Long-term sensitivity of soil carbon turnover to warming. Nature 433 (7023), 298–301. doi:10.1038/nature03226
Kumar, A., Kumar, M., Pandey, R., Zhiguo, Y., and Cabral-Pinto, M. (2021). Forest soil nutrient stocks along altitudinal range of uttarakhand himalayas: An aid to nature based climate solutions. Catena 207, 105667. doi:10.1016/j.catena.2021.105667
Li, J., Wang, G., Mayes, M. A., Allison, S. D., Frey, S. D., Shi, Z., et al. (2019). Reduced carbon use efficiency and increased microbial turnover with soil warming. Glob. Chang. Biol. 25 (3), 900–910. doi:10.1111/gcb.14517
Liang, C., and Balser, T. C. (2011). Microbial production of recalcitrant organic matter in global soils: Implications for productivity and climate policy. Nat. Rev. Microbiol. 9 (1), 75. doi:10.1038/nrmicro2386-c1
Lin, J., Zhu, B., and Cheng, W. (2015). Decadally cycling soil carbon is more sensitive to warming than faster-cycling soil carbon. Glob. Chang. Biol. 21 (12), 4602–4612. doi:10.1111/gcb.13071
Martin, A., Mariotti, A., Lavelle, P., and Vuattoux, R. (1990). Estimate of organic matter turnover rate in a savanna soil by 13C natural abundance measurements. Soil Biol. Biochem. 22 (4), 517–523. doi:10.1016/0038-0717(90)90188-6
Melillo, J., Steudler, P., Aber, J., Newkirk, K., Lux, H., Bowles, F., et al. (2002). Soil warming and carbon-cycle feedbacks to the climate system. Science 298 (5601), 2173–2176. doi:10.1126/science.1074153
O’brien, S. L., Jastrow, J. D., Mcfarlane, K. J., Guilderson, T. P., and Gonzalez-Meler, M. A. (2013). Decadal cycling within long-lived carbon pools revealed by dual isotopic analysis of mineral-associated soil organic matter. Biogeochemistry 112 (1-3), 111–125. doi:10.1007/s10533-011-9673-0
Paterson, E., Midwood, A. J., and Millard, P. (2009). Through the eye of the needle: A review of isotope approaches to quantify microbial processes mediating soil carbon balance. New Phytol. 184 (1), 19–33. doi:10.1111/j.1469-8137.2009.03001.x
Paul, E., Morris, S., and Bohm, S. (2001). “The determination of soil C pool sizes and turnover rates: Biophysical fractionation and tracers,” in Assessment Methods for Soil Carbon. 1st Edn. Editors M. John, Kimble, F. Ronald, B. A. Follett, and Stewart (CRC Press), 14. https://sc.panda321.com/extdomains/books.google.com/books?hl=zh-CN&lr=&id=ijFRDwAAQBAJ&oi=fnd&pg=PA193&ots=E4ypVaIu1Y&sig=RpRk6aCa_JGFhKI7NJA23IZ3YTo.
Phillips, C. L., Murphey, V., Lajtha, K., and Gregg, J. W. (2016). Asymmetric and symmetric warming increases turnover of litter and unprotected soil C in grassland mesocosms. Biogeochemistry 128 (1-2), 217–231. doi:10.1007/s10533-016-0204-x
Poeplau, C., Kätterer, T., Leblans, N. I., and Sigurdsson, B. D. (2017). Sensitivity of soil carbon fractions and their specific stabilization mechanisms to extreme soil warming in a subarctic grassland. Glob. Chang. Biol. 23 (3), 1316–1327. doi:10.1111/gcb.13491
Roscoe, R., Buurman, P., Velthorst, E., and Vasconcellos, C. (2001). Soil organic matter dynamics in density and particle size fractions as revealed by the 13C/12C isotopic ratio in a Cerrado's oxisol. Geoderma 104 (3-4), 185–202. doi:10.1016/s0016-7061(01)00080-5
Schimel, J. P., and Schaeffer, S. M. (2012). Microbial control over carbon cycling in soil. Front. Microbiol. 3, 348. doi:10.3389/fmicb.2012.00348
Schneckenberger, K., and Kuzyakov, Y. (2007). Carbon sequestration under Miscanthus in sandy and loamy soils estimated by natural 13C abundance. Z. Pflanzenernahr. Bodenk. 170 (4), 538–542. doi:10.1002/jpln.200625111
Stuble, K. L., Ma, S., Liang, J., Luo, Y., Classen, A. T., and Souza, L. (2019). Long-term impacts of warming drive decomposition and accelerate the turnover of labile, not recalcitrant, carbon. Ecosphere 10 (5), e02715. doi:10.1002/ecs2.2715
Tian, Q., He, H., Cheng, W., and Zhang, X. (2014). Pulse-dynamic and monotonic decline patterns of soil respiration in long term laboratory microcosms. Soil Biol. Biochem. 68, 329–336. doi:10.1016/j.soilbio.2013.10.015
Vain, A-C., Rakotondrazafy, N., Razanamalala, K., Trap, J., Marsden, C., Blanchart, E., et al. (2021). The fate of primed soil carbon between biomass immobilization and respiration is controlled by nutrient availability. Eur. J. Soil Biol. 105, 103332. doi:10.1016/j.ejsobi.2021.103332
Vance, E. D., Brookes, P. C., and Jenkinson, D. S. (1987). An extraction method for measuring soil microbial biomass C. Soil Biol. Biochem. 19 (6), 703–707. doi:10.1016/0038-0717(87)90052-6
Virginia, R. A. (1993). Measurement of microbial biomass in arctic tundra soils using fumigation-extraction and substrate-induced respiration procedures. Soil Biol. Biochem. 25 (1), 135–141. doi:10.1016/0038-0717(93)90251-6
Keywords: warming, decadally cycling soil organic carbon, 13 C natural abundance, microbial biomass, terrestrial ecosystems
Citation: Liu D, Zhang W, Xiong C and Nie Q (2023) Warming increases the relative change in the turnover rate of decadally cycling soil carbon in microbial biomass carbon and soil respiration. Front. Earth Sci. 10:1089544. doi: 10.3389/feart.2022.1089544
Received: 04 November 2022; Accepted: 29 November 2022;
Published: 19 January 2023.
Edited by:
Dafeng Hui, Tennessee State University, United StatesReviewed by:
Wenjuan Huang, Iowa State University, United StatesCancan Zhao, Henan University, China
Copyright © 2023 Liu, Zhang, Xiong and Nie. This is an open-access article distributed under the terms of the Creative Commons Attribution License (CC BY). The use, distribution or reproduction in other forums is permitted, provided the original author(s) and the copyright owner(s) are credited and that the original publication in this journal is cited, in accordance with accepted academic practice. No use, distribution or reproduction is permitted which does not comply with these terms.
*Correspondence: Qingyu Nie, bnF5MzE4QDE2My5jb20=