- 1School of Civil and Resource Engineering, University of Science and Technology Beijing, Beijing, China
- 2Institute of Geology, Chinese Academy of Geological Sciences, Beijing, China
- 3Postdoctoral Programme, Guosen Securities, Shenzhen, China
Lower crust-derived granitic rocks provide constraints on the crustal reworking process and consequently give hints on the destruction mechanism of the cratons. The North China Craton (NCC) underwent extensive crustal melting in the Mesozoic. This study investigated granitic intrusions in the Dazeshan region of the Jiaodong Peninsula. Whole-rock major and trace element analyses and zircon U-Pb ages coupled with Hf isotopes were used to reveal the crustal reworking processes. Zircons separated from the quartz porphyry, Linglong granite, rhyolite porphyry, and biotite granite showed weighted mean 206Pb-238U ages of 119.2 ± 1.0 Ma, 140.2 ± 1.0 Ma, 120.6 ± 0.5 Ma, and 119.9 ± 0.7 Ma, respectively. The quartz porphyry, rhyolite porphyry, and biotite granite had high silica contents (SiO2 = 74–77 wt.%) but low MgO, Co, and Ni concentrations. The calculated εHf(t) values for the rhyolite porphyry and the biotite granite ranged from −18.3 to −20.0 and −17.8 to −20.2, respectively. These geochemical features imply ancient crust sources. The quartz porphyry showed distinct primitive mantle-normalized rare earth element (REE) patterns and was characterized by lower ΣREE content and lack of pronounced negative Eu anomalies. Whole-rock and zircon Dy/Yb ratios showed no correlation with whole-rock Rb/Sr ratios and zircon Hf contents, reflecting limited effects of crystal fractionation. The Ba/La ratios were also high (>150), but the Sr/Y and La/Yb ratios were low (Sr/Y < 50; La/Yb < 15). These features likely indicate that the quartz porphyry was generated by water-fluxed melting without differentiation. The rhyolite porphyry and biotite granite shared many geochemical similarities, denoting a unified source. The high La/Yb (>30) but low Sr/Y (<20) ratios and apparent negative Eu anomalies indicated plagioclase fractionation. Decreased zircon Dy/Yb with increasing Hf concentrations reflected noticeable amphibole fractionation. These two suites had fairly low Ba/La ratios. These data together point toward an identical source: dehydration melting of a relatively thickened crust. These melts experienced crystal fractionation after extraction. We propose that the intrusions were generated by the underplating of water-rich mafic magma, which provided both fluid and heat and finally induced two kinds of melting.
1 Introduction
Cratons are generally considered the most stable part of the lithosphere because the great thickness of the continental mantle beneath cratons prevents them from being destroyed by the convective mantle. However, decades of research have greatly improved our understanding and we now know that stable cratons can be destroyed (Gao et al., 2004; Xu et al., 2009; Lee et al., 2011; Wu et al., 2019). The NCC has stabilized following the amalgamation between the western and eastern blocks at about 1.9 Ga (Zhao et al., 2005). However, it was reworked during the Mesozoic and the thick lithosphere underwent extensive thinning, particularly at the eastern part of the NCC (Menzies et al., 2007; Chen et al., 2008; Wu et al., 2019). The destruction of NCC was accompanied by crustal reworking and the development of extensional structures and many metamorphic core complexes (like the Gudaoling and Linglong) and sedimentary basins (Charles et al., 2012; Meng et al., 2019; Wu et al., 2019; Zheng and Gao, 2021). Thus, these processes are used as indicators of decratonization in the NCC.
This thinning process of the lithosphere mantle can be traced at the shallow crustal levels by Jurassic-Cretaceous granitic rocks, as they represent thermal or tectonic disturbances underneath the thick crust (Chappell, 2004; Weinberg and Hasalová, 2015; Gao et al., 2016; Zheng and Gao, 2021). Although extensive studies have been conducted on these magmatic rocks to demonstrate the timing and mechanisms of NCC destruction, some divergences exist regarding the timing and duration of the NCC destruction. The onset of NCC destruction remains elusive because the understanding of “destruction” differs (Xu et al., 2009). The northern and southern margins of the NCC were subducted by the Paleo-Asian Oceanic slab and the South China Block during the Carboniferous-Permian and Late Triassic, respectively (Windley et al., 2010). The resultant magmatism in the northern and southern margins indicated changes in lithospheric structure and thermal structure. Following this logic, the NCC destruction initiated in different craton margins in the Carboniferous and Triassic (Xu et al., 2009). Nevertheless, the destruction of NCC is now broadly recognized to have peaked in the Early Cretaceous (Wu et al., 2005a), as indicated by extensive granitic magmatism associated with mantle-crust interactions.
The mechanism of lithosphere thinning also remains under debate. One school of thought argues that the thinning occurs mechanically by delamination (Gao et al., 2004; Ma et al., 2014), while other researchers believe that the thinning proceeds gradually via a thermal-chemical erosion process (Xu et al., 2009; Geng et al., 2019). Hou et al. (2007) inferred that the ∼130 Ma Guojialing suite adakitic granitoids were formed by crustal thickening during the descent into the asthenosphere (delamination). Ma et al. (2014) proposed that the lithosphere mantle was rapidly replaced by the fertile asthenosphere mantle at ca. 120 Ma by delamination based on investigations of the mafic dykes in Jiaodong. These results drive the timing of delamination, if it occurred, towards later times. Therefore, the genesis of these Early Cretaceous magmatic rocks is crucial for understanding the crustal evolution of the NCC. However, how these granitic rocks were generated remains undetermined in the NCC (e.g., fluid fluxed or dehydration melting), particularly those magmatic rocks formed at ca. 120 Ma. This impedes our knowledge of decratonization processes. This study investigated the petrogenesis of the granitic rocks that intruded into the Jurassic Linglong granite in the Dazeshan region in Jiaodong Penisular, to reveal the crustal reworking processes.
2 Geological background
The Jiaodong Penisular is an integral part of the NCC, which has Early Archean crustal remnants (Jahn and Zhang, 1984; Liu et al., 1992). The NCC was stabilized through the amalgamation of the eastern and western blocks during a Paleoproterozoic orogenic event along the Trans-North China Orogen (Figure 1) (Zhao, 2001). Both blocks are dominated by Late Archaean tonalitic–trondhjemitic–granodioritic (TTG) gneiss complexes with minor supracrustal rocks with metamorphic ages of ∼2.5 Ga (Zhao, 2001). The Jiaodong Penisula is located in the southeastern part of the NCC and was displaced to its current location by the approximately 2,400 km Tanlu fault (Zhu et al., 2009). Three main tectonic units constitute the Jiaodong Peninsula: the Jiaobei terrane, the Sulu orogen, and the Jiaolai basin (Figure 1).
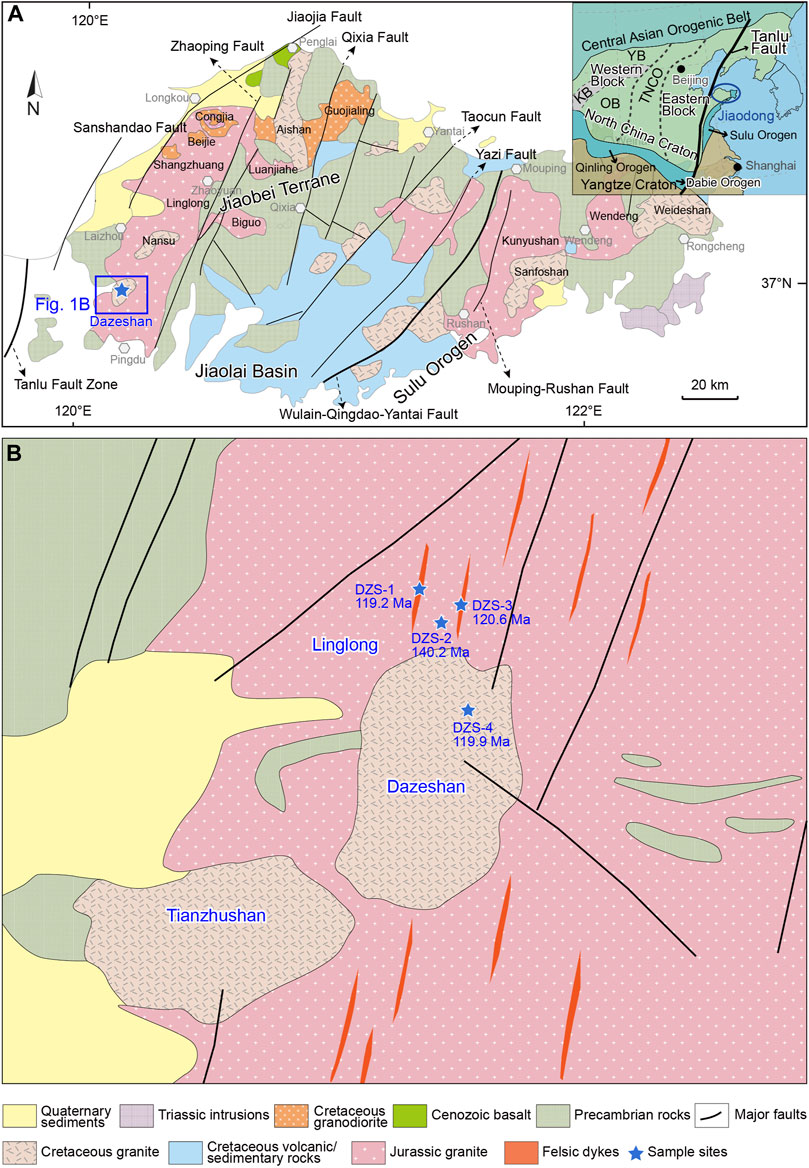
FIGURE 1. (A) Geological map of the Jiaodong Peninsula, showing major structures, lithological units, and study area (Song et al., 2021). (B) The U-Pb ages obtained in this study are noted on the map.
The Jiaobei terrane, an integral part of the NCC, comprises Archean-Paleozoic metamorphic rocks and TTG gneiss (such as biotite gneiss and plagioclase amphibolite). These rock units are mainly located in the Qixia area. The Jurassic Linglong granite, along with the Luanjianhe and Biguo granites, intrudes into the Precambrian rocks and together constitute the composite Linglong pluton (Figure 1). The zircon U-Pb ages of this composite pluton range from 170 to 150 Ma (Yang et al., 2012; Yang et al., 2017). The volumetrically smaller Guojialing, Congjia, Beijie, and Shangzhuang granodiorite were subsequently emplaced during the Early Cretaceous. The zircon U-Pb ages of these plutonic rocks range from 130 to 127 Ma (Yang et al., 2012; Jiang et al., 2016). The Aishan and Nansu porphyry granites, which feature large K-feldspar phenosrysts, are emplaced within the Guojialing granodiorite and the Linglong granite, respectively, and have zircon U-Pb ages of 118–116 Ma (Goss et al., 2010; Li et al., 2012). The Sulu orogen is situated to the east of the Wulian-Qingdao-Yantai fault and is considered the suture zone separating the NCC and South China Block (Wu and Zheng, 2013). Many ultrahigh- to high-pressure metamorphic rocks are preserved in the Sulu orogen. Additionally, the Triassic alkaline complex in the Rongcheng area (Shidao) dates to 225–205 Ma and represents magmatism related to mantle upwelling after continent-continent collision (Chen et al., 2003). The Jiaolai basin comprises volcanic and sedimentary sequences deposited in the Early Cretaceous (Zhang et al., 2008). The main volcanic rocks in the Jiaolai basin are Qingshan group basic to felsic rocks, including rhyolite, andesite, dacite, and tuff. Laiyang and Wangshi group sedimentary rocks (sandstone and mudstone) cover the Qingshan group volcanic rocks. Cenozoic basalt, although rarely exposed, is mainly reported in the Penglai area (Figure 1).
3 Samples and analytical methods
The samples investigated in this study were collected in the Dazeshan region at the Jiaobei terrane (Figure 1B). Four types of magmatic rocks were sampled: quartz porphyry (DZS-1), Linglong granite (DZS-2), rhyolite porphyry (DZS-3), and Dazeshan biotite granite (DZS-4) (Figure 2). From field observations, the quartz porphyry, rhyolite porphyry, and Dazeshan biotite granite all intruded the Linglong granite. The Linglong granite is predominantly composed of plagioclase, K-feldspar, quartz, and biotite (Figures 2A, 3A). The sizes of the plagioclase, K-feldspar, and quartz are similar (2–5 mm). The biotite crystals are smaller and appear to be tabular. The samples from the Linglong granite were fresh. The quartz porphyry is a fine-grained grayish felsic rock that contains quartz and minor K-feldspar phenocrysts (Figures 2B, 3B). The volume fraction of the quartz phenocryst is <5%. The matrix contains micron-scale quartz and feldspar crystals. The K-feldspar in both phenocrysts and matrix was subjected to weathering and formed small amounts of sericite. The rhyolite porphyry sample (DZS-3) contained quartz and K-feldspar phenocrysts (Figures 2C, 3C). The phenocrysts were approximately 2–5 mm in diameter. The Dazeshan biotite granite (DZS-4) is a coarse-grained plutonic rock that intruded into the Linglong granite (Figure 1). The Dazeshan biotite granite had the same mineral assemblages as the Linglong granite but with larger crystal sizes.
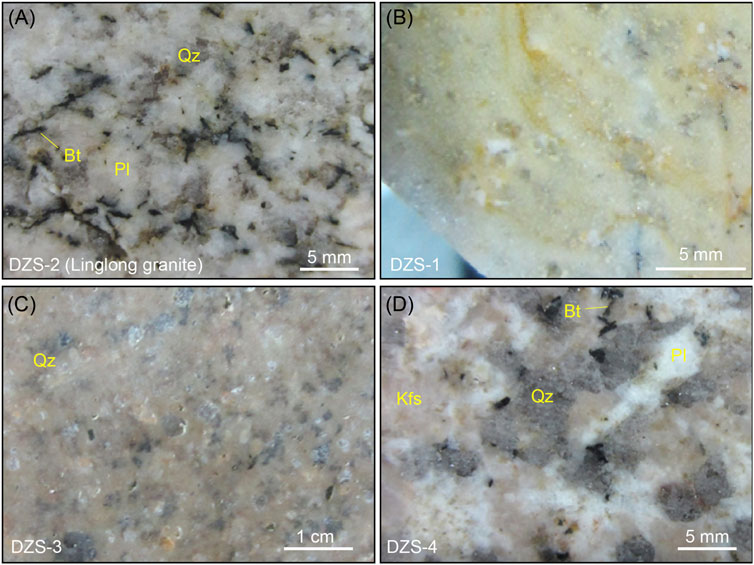
FIGURE 2. (A–D) Photographs of Linglong granite, quartz porphyry, rhyolite porphyry, and Dazeshan biotite granite.
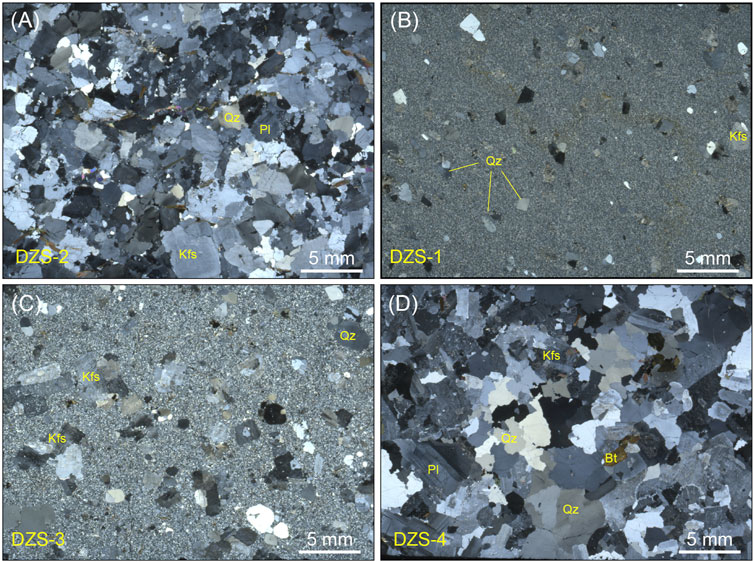
FIGURE 3. Microscopic images of Linglong granite (A), quartz porphyry (B), rhyolite porphyry (C), and Dazeshan biotite granite (D). Abbreviations: Kfs = K-feldspar, Pl = plagioclase, Qz = quartz, Bt = biotite. The abbreviations for the minerals are after Whitney and Evans. (2010).
3.1 Major and trace element analyses
Major element data for whole-rock samples were obtained by X-ray fluorescence (XRF) spectrometry on fused glass discs using a PANalytical AXIOS Minerals instrument at the Institute of Geology and Geophysics, Chinese Academy of Sciences. Glass discs for WD-XRF analyses were prepared by fusing 0.5 g of the standard sample with 5.0 g of lithium tetraborate-metaborate mixture (2:1) using Pt-Au crucibles and moulds on fusion equipment with gas burners. Loss on ignition (LOI) was measured as the weight loss of the samples after 1 h of baking at a constant temperature of 1,000°C in a muffle furnace. The ferrous iron content was determined using the titration method described by Xue et al. (2017). Whole-rock trace element analyses were performed at the National Research Center of Geoanalysis, Chinese Academy of Geological Science by inductively coupled plasma mass spectrometry (ICP-MS) on a TJA PQ-ExCell system. Standards GSR1 and GSR9 were used to monitor the analytical reproducibility. 2σ uncertainties are <5% for elements >10 ppm and <10% for elements <10 ppm.
3.2 Zircon U-Pb dating and trace elements
Zircons were separated using conventional heavy liquid and magnetic separation techniques. They were mounted in epoxy and polished to a surface. Transmitted, reflected light and cathodoluminescence (CL) images were captured to acquire information about the inner structure, surface characteristics, and CL textures of the zircons, respectively. Laser ablation-inductively coupled plasma-mass spectrometry (LA-ICPMS) zircon U-Pb analyses were performed on an Agilent 7900 ICP-MS instrument equipped with a 193 nm laser ablation system at the Institute of Geology, Chinese Academy of Geological Sciences. A laser spot size of 30 μm was used to ionize the samples. The laser was operated at an energy of 2 J/cm2 and a repetition rate of 5 Hz.
3.3 Zircon Lu-Hf isotopes
In situ zircon Lu-Hf isotopic analyses were performed on a Neptune MC-ICPMS coupled with a 193 nm laser ablation system at the National Research Center for Geoanalysis. An ArF excimer laser ablation system was used for the laser ablation analysis. The analyses used a laser repetition rate of 10 Hz at 100 mJ. A spot size of ca. 40 μm was applied depending on the size of the zircon grains. The ionized samples were carried by Ar gas. The detailed analytical procedures are described in (Wu et al., 2006).
4 Analytical results
4.1 Whole-rock major and trace elements
Eleven sample powders from three intrusions (except for the Linglong granite) were analyzed. The whole-rock geochemical data are presented in Figures 4, 5, 6 and Table 1. Overall, the silica contents of the quartz porphyry, rhyolite porphyry, and Dazeshan biotite granite are high (74–77 wt.%). In the total alkali vs. silica diagram (Figure 4A), these samples plot in the granite field. They belong to the high-K calc-alkaline series in the plot of SiO2 vs. K2O diagram (Figure 4B). In the plots of SiO2 vs. CaO, Al2O3, MgO, TFeO, TiO2, and P2O5, the three types of magmatic rocks display distinct major element contents (Figure 5). CaO concentrations in the Dazeshan biotite granite are the highest among the samples, while the quartz porphyry and rhyolite porphyry have CaO contents around 0.4 wt.%. The aluminum content for the quartz porphyry is the highest among the three intrusions (14.01–14.34 wt.%), about 1–2 wt.% higher than the other two suites. The Al2O3 contents in the Dazeshan biotite granite are in the range of 13.22–13.29 wt.%. The MgO concentrations in the intrusions are low (<0.4 wt.%) and show negative correlations with SiO2 (Figure 5C). Total FeO contents are lower in the quartz porphyry and rhyolite porphyry (1.11–1.40 wt.% and 1.07–1.12 wt.%, respectively) and higher in the Dazeshan biotite granite (1.63–1.72 wt.%) (Figure 5D). The concentrations of TiO2 and P2O5 are elevated in the Dazeshan biotite granite compared to those in the quartz and rhyolite porphyries (Figures 5E, F).
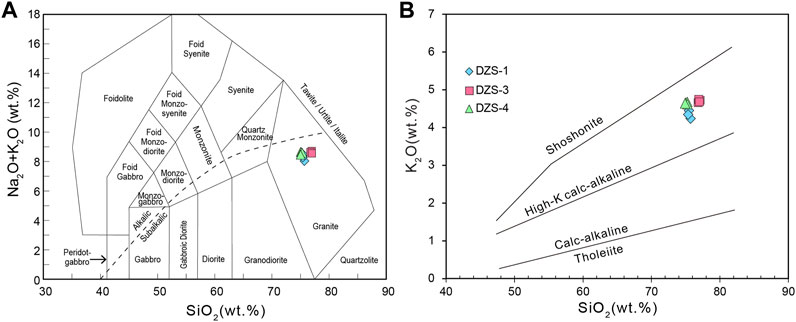
FIGURE 4. Total alkaline vs. SiO2 (A) and SiO2 vs. K2O (B) diagrams (Peccerillo and Taylor, 1976; Le Bas et al., 1986).
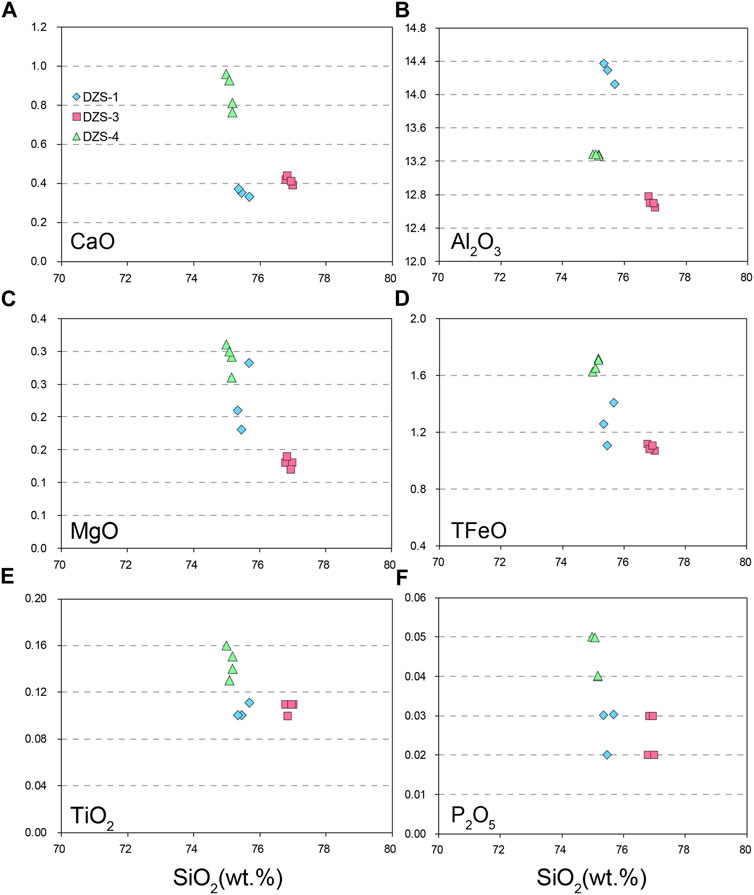
FIGURE 5. (A–F) Harker-type diagrams of whole-rock CaO, Al2O3, MgO, total FeO, TiO2, and P2O5 versus SiO2 contents for the studied intrusions.
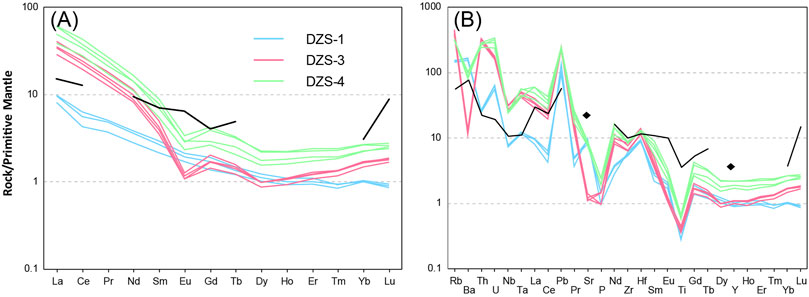
FIGURE 6. Primitive mantle-normalized REE patterns (A) and trace element variation diagrams (B) for the quartz porphyry, rhyolite porphyry, and Dazeshan biotite granite. The normalization values are from McDonough and Sun (1995). Black line: average granulite terrane/xenolith concentrations in the NCC (Jahn and Zhang, 1984; Liu et al., 2001; Zhai et al., 2001).
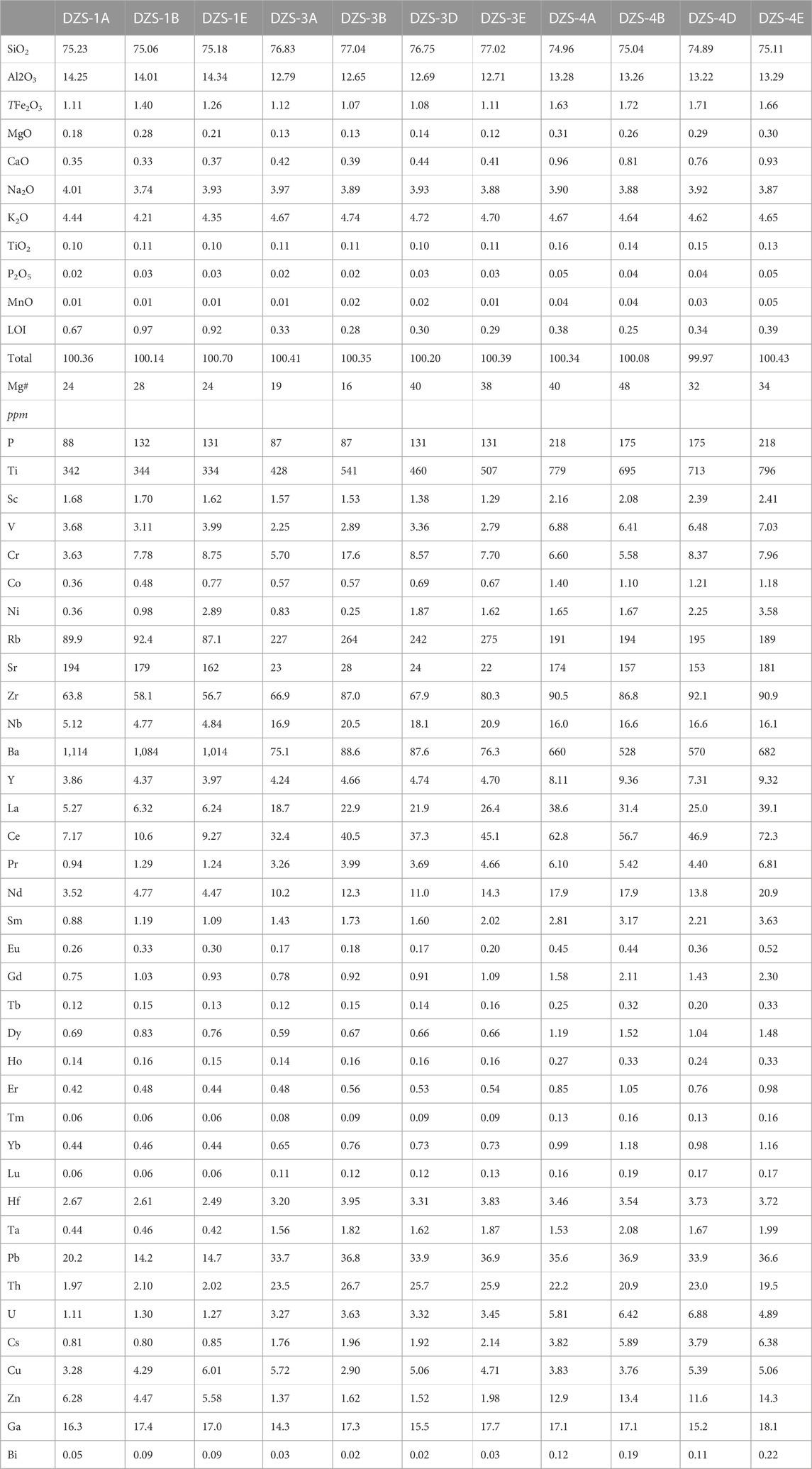
TABLE 1. Major (wt %) and trace (ppm) element concentrations for igneous rocks in the Dazeshan region.
The whole-rock trace element data are normalized to the primitive mantle (PM) in REE and spider diagrams (Figure 6). In the REE diagram, the three intrusions display different enrichment and/or depletion patterns. The samples from quartz porphyry have low contents of total REE (20.7–27.7 ppm). Additionally, the light rare earth elements (La-Eu; LREE) are not strongly fractionated from the heavy rare earth elements (Gd-Lu; HREE) given the LREE/HREE ratios from 6.8 to 7.6. No apparent negative Eu anomaly is observed in the PM-normalized REE pattern. The calculated Eu/Eu* values range from 0.89 to 0.98 for the quartz porphyry samples (Eu/Eu* represents the europium anomaly calculated as EuN/(SmN×GdN)0.5, the subscript “N” denotes the PM normalized value from McDonough and Sun, 1995). In the spider diagram (Figure 6B), high field strength elements (HFSE) like P and Ti in the quartz porphyry show negative anomalies. However, the large ion lithophile elements (LILE) like Rb and Ba, along with fluid mobile element Pb, are strongly enriched.
The REE patterns for the rhyolite porphyry and Dazeshan biotite granite show contrasting features compared to the quartz porphyry. Generally, these two intrusions have elevated REE concentrations compared to the quartz porphyry and display listric-shaped patterns. Y These two porphyries also show obvious negative Eu anomalies. The Eu/Eu* for the rhyolite porphyry and Dazeshan biotite granite are 0.40–0.48 and 0.52–0.66, respectively. The LREE and HREE are strongly fractionated in these two intrusions. The LREE/HREE ratios are 23.7–26.0 for the rhyolite porphyry and 16.8–26.8 for the Dazeshan biotite granite. However, the Dazeshan biotite granite has higher total REE concentrations than that of the rhyolite porphyry (97.6–150.2 ppm vs. 69.7–96.4 ppm). In the spider diagram, the rhyolite porphyry and Dazeshan biotite granite display overall similar patterns except for Ba and Sr. The rhyolite porphyry shows more depletion of these two elements compared to the Dazeshan biotite granite (Figure 6B). Elements from Th to Pb in Figure 6B are elevated compared to the quartz porphyry.
4.2 Zircon U-Pb ages and trace elements
Four sets of zircon U-Pb ages and trace element data were obtained in this study. The analytical results are presented in Figures 7, 8 and Table 2 and Table 3. The LA-ICPMS zircon data from each rock type are briefly described below.
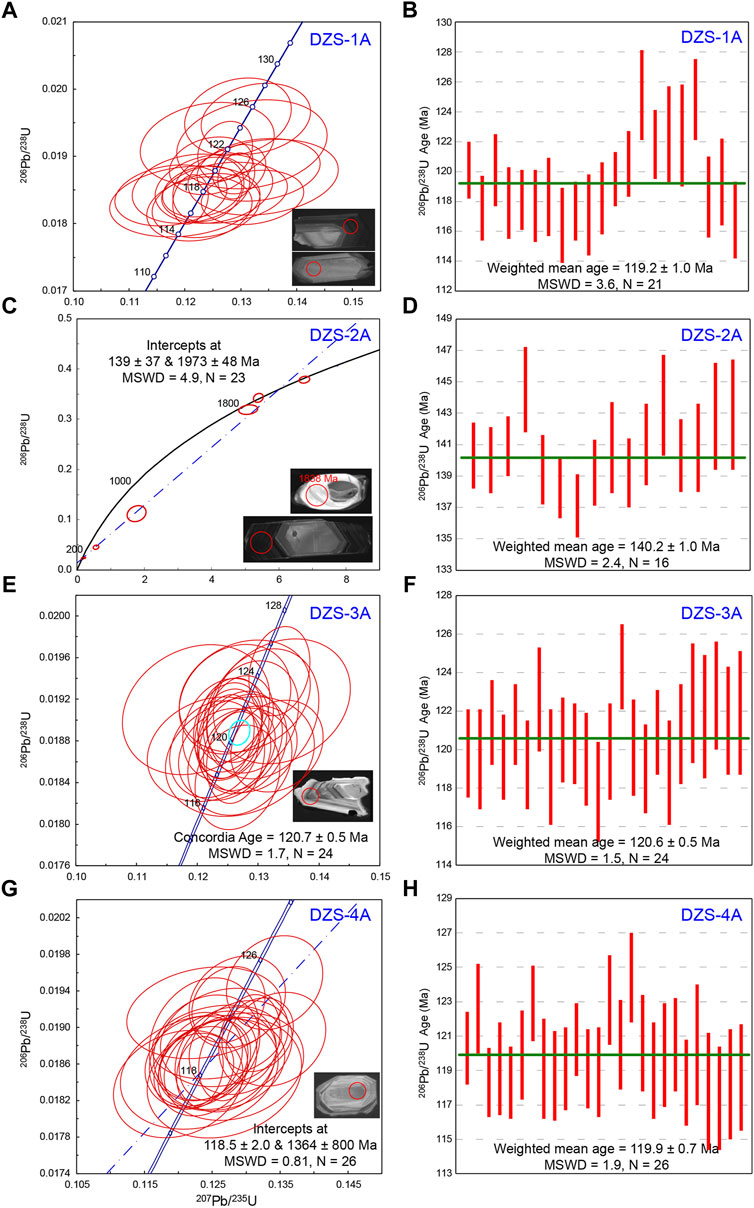
FIGURE 7. Zircon U-Pb concordia and weighted mean 238U/206Pb ages of the studied magmatic rocks. The red circles in the zircon grains are analytical spots and are 30 μm in diameter. (A,B) Quartz porphyry; (C,D) Linglong granite; (E,F) rhyolite porphyry; (G,H) Dazeshan biotite granite.
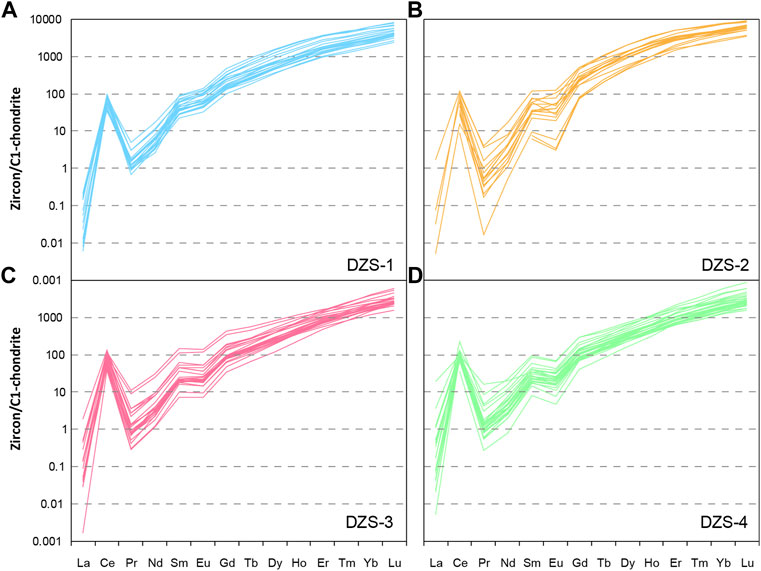
FIGURE 8. Zircon REE concentrations of quartz porphyry, Linglong granite, rhyolite porphyry, and Dazeshan biotite granite, respectively (A–D). The normalization values are from Sun and McDonough (1989).
4.2.1 Quartz porphyry (DZS-1A)
Zircons from this sample are clear by microscopy and contain little mineral inclusions. Additionally, they display oscillatory zoning patterns under CL images. The lengths of these zircons are about 100–200 μm, while the widths are about 40–100 μm. Most of the zircons have a CL brighter grayish core without oscillatory zoning but are overgrown by CL dark rims with oscillatory zonings (Figure 7A). The zircon U-Pb ages in these two different areas are consistent. Twenty-one spot analyses on zircons were conducted. These isotope data cannot construct a well-defined Concordia U-Pb age but give a weighted mean 206Pb-238U age of 119.2 ± 1.0 Ma (Figures 7A, B; MSWD = 3.6, N = 21). The U and Th concentrations in the zircons have wide ranges (201–3,411 ppm and 74–2084 ppm, respectively), whereas the Th/U ratios are relatively restricted between 0.36 and 1.03, with an average value of 0.62 (Table 2).
4.2.2 Linglong granite (DZS-2A)
Twenty-three zircons were analyzed in this sample. Most of these zircons are columnar with lengths of about 100–200 μm, and widths of 50–100 μm (mostly ∼50 μm). Mineral inclusions like apatite or rutile are common in these zircons. The zircons have simple CL textures, with or without an oscillatory zoning rim. The cores of the zircons appear to be dark gray. Inherit zircons, however, are frequently observed and featured by bright CL (Figure 7C inset). Six of the twenty-four zircons underwent Pb loss and fall out of the Concordia line, yet they construct two ages intercepting at 139 ± 37 and 1973 ± 48 Ma (MSWD = 4.9, N = 23). The two oldest zircons have 207Pb-236Pb ages of 2078 ± 30 Ma and 1838 ± 86 Ma, respectively. The young group of zircons constructs a weighted mean 206Pb-238Pb age of 140.2 ± 1.0 Ma (Figure 7D, MSWD = 2.4, N = 16). For the younger group zircons, which are considered the crystallization phase from the magma (see later discussion), the U concentrations range from 348 to 3,048 ppm, while the Th content varies between 58 and 1866 ppm (Table 2). The corresponding Th/U ratios are 0.08–1.29, with an average value of 0.47.
4.2.3 Rhyolite porphyry (DZS-3A)
Twenty-four zircons were separated from the rhyolite porphyry sample. Zircon morphology exhibits tiny differences from the quartz porphyry and Linglong granite samples. These grains are equally sized, with length-to-width ratios of around 1.5–2.0. The lengths of most zircons are around 150 μm. Their CL characters vary. Some zircons have a bright core with CL dark/bright oscillatory zoning rims, while others have CL bright oscillatory zoning in the whole grain. Nevertheless, the zircon ages in these different areas are the same. All the zircons construct a Concordia U-Pb age of 120.7 ± 0.5 Ma (Figure 7E; MSWD = 1.7, N = 24 and a weighted mean 206Pb-238Pb age of 120.6 ± 0.5 Ma (Figure 7F; MSWD = 1.5, N = 24). The zircon U and Th concentrations are 179–1,533 ppm and 191–1,037 ppm, respectively. The average U and Th concentrations are 575 ppm and 360 ppm, respectively. The zircon Th/U ratios are 0.42–1.58.
4.2.4 Dazeshan biotite granite (DZS-4A)
Zircons from the biotite granite are characterized by low length-to-width ratios (1–1.5). Most of these zircons are 100–150 μm in size and have CL bright/dark oscillatory zoning occupying the whole zircon grain. Generally, the CL bright zone is rimmed by a CL dark zone with or without oscillatory zoning patterns. The data of the 26 analyzed zircons yielded ages that intercept at 1,364 ± 800 Ma and 118.5 ± 2.0 Ma (Figure 7G, MSWD = 0.81). The weighted mean 206Pb-238Pb age is 119.9 ± 0.7 Ma (Figure 7H, MSWD = 1.9). The zircon U concentrations are 181–3,275 ppm, while the Th concentrations are 148–2,233 ppm. The Th/U ratios in zircons range from 0.47 to 1.46 (Table 2).
Zircon trace element data of the four samples are presented in Figure 8 and Table 3. All zircons feature HREE enrichment but LREE depletion (except for Ce, which displays a strong positive anomaly) in PM-normalized REE patterns (Figures 8A–D). Europium shows a relatively weak negative anomaly in zircons from the quartz porphyry. However, the negative Eu anomalies are strikingly obvious in the other three zircon samples.
4.3 Zircon Lu-Hf isotopes
Zircon Hf isotopes were analyzed in rhyolite porphyry and the Dazeshan biotite granite. The hafnium isotope data are presented in Figure 9 and Table 4. For the rhyolite porphyry, 176Hf/177Hf values are 0.282133–0.282181. The calculated εHf(t) was −18.3 to −20.0. The two stage model ages are 3.2 to 3.4 Ga. Five spot analyses of zircons from the Dazeshan biotite granite give a176Hf/177Hf values between 0.282130 and 0.282195 (εHf(t) = −17.8 to −20.2).
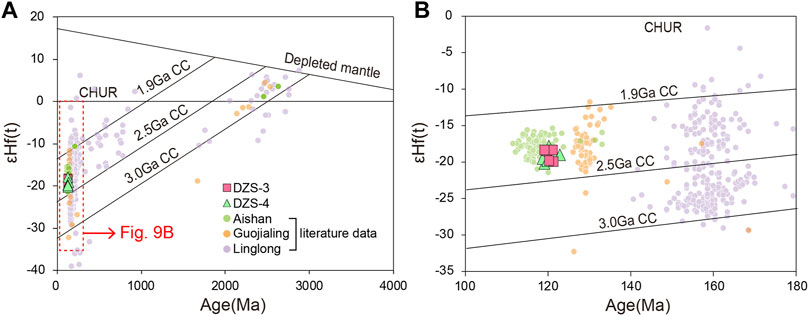
FIGURE 9. Zircon Hf isotopic data from rhyolite porphyry (A) and Dazeshan biotite granite (B). Published zircon isotope data are compiled from Yang et al. (2012; 2017), Ma et al. (2013; 2014), and Li et al. (2019).
5 Discussion
5.1 Genesis of the ∼120 Ma magmatic rocks
Granites are produced predominantly by anatexis of crustal rocks (Brown, 2013; Clemens et al., 2020), although in some cases, the granites are generated by fractional crystallization of mantle-derived melts (Soesoo, 2000; Thompson et al., 2002). For the samples investigated in this study, the SiO2 contents of the three intrusions (except for the Linglong granite) are very high (74–77 wt. %). Meanwhile, no considerable mafic melts occurred along with these granites (Figure 1), eliminating the possibility of fractional crystallization of mantle-derived melts. The Hf isotope compositions from the zircons also provide information on the sources of the melts well (Figure 9). The εHf(t) values are restricted for both the rhyolite porphyry and Dazeshan biotite granite (ca. −18 to −20), comparable to other granitic rocks reported in the Jiaodong Penisula (e.g., Linglong granite and Aishan granodiorite). For example, the Linglong granite was interpreted to be the melting products of the Archean lower crust, with almost no contribution from the mantle (Ma et al., 2013; Li et al., 2019). The two stage model ages calculated via zircon Hf isotope compositions are 3.2–3.4 Ga for the rhyolite porphyry and Dazeshan biotite granite (Table 4), demonstrating their ancient continental crust source. Therefore, these granite rocks are generated by crustal anatexis.
From the perspective of the whole-rock and zircon trace element data, the three intrusions have strikingly different geochemical features (Figures 6, 10). This implies that these intrusions probably experienced distinct melting or differentiation processes. The following section discusses the chemical variations in these magmatic rocks and their magmatic evolution.
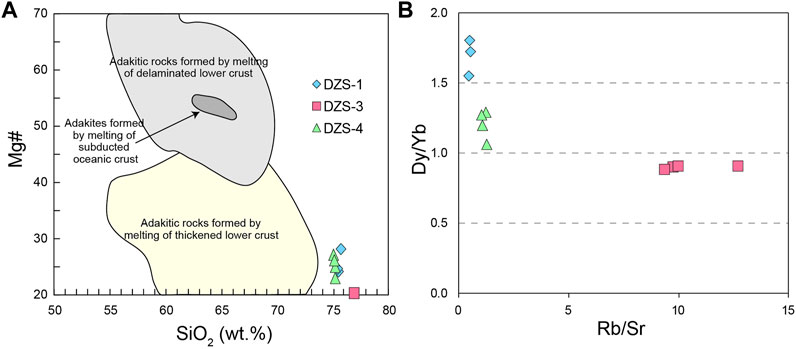
FIGURE 10. Mg# vs. SiO2 (A) and Dy/Yb vs. Rb/Sr (B) diagrams. Adakite fields are adopted from Sen and Dunn (1994), Rapp and Watson (1995), and Rapp et al. (1999) and references therein.
5.1.1 Quartz porphyry (DZS-1)
The quartz porphyry is characterized by low LREE content and a lack of negative Eu anomaly in the REE pattern (Figure 6A). The LREE concentrations in the quartz porphyry here are even lower than the average concentrations of the granulite terranes/xenoliths in the NCC, which were regarded as representative of the lower crust fragments (Liu et al., 1999; Zhai et al., 2001; Liu et al., 2004). Partial melting of the granulite rocks would give rise to higher REE content in the melt considering their incompatibility. The discordant REE patterns between the quartz porphyry and granulite terranes/xenoliths differ from what would be expected if the quartz porphyry is the melting product of lower-crust granulite. Magmatic processes like fractionation crystallization or magma mixing could exert a conspicuous influence on the compositions of the melt (DePaolo, 1981; Yang et al., 2004; Cao et al., 2022).
The whole-rock major and trace elements, trace elements ratios (Th/Nb, La/Sm, and Rb/Sr), and Mg# values vary little among the three samples from the quartz porphyry, indicating that fractionation crystallization of major mineral phases (e.g., amphibole, K-feldspar) probably plays a negligible role in generating such low REE concentrations. This supposition is supported by the fact that Dy/Yb in zircons does not decrease with increasing Hf concentrations (Figure 11A), implying no considerable amphibole fractionation (Davidson et al., 2007). Rather, Dy/Yb increases with Hf enrichment in zircons. This may indicate zircon separation, which reduces the Yb contents of the remnant melt. Eu/Eu* values in zircon are high and are not linearly correlated with Hf concentrations (Figure 11B), eliminating the potential influence of plagioclase fractionation.
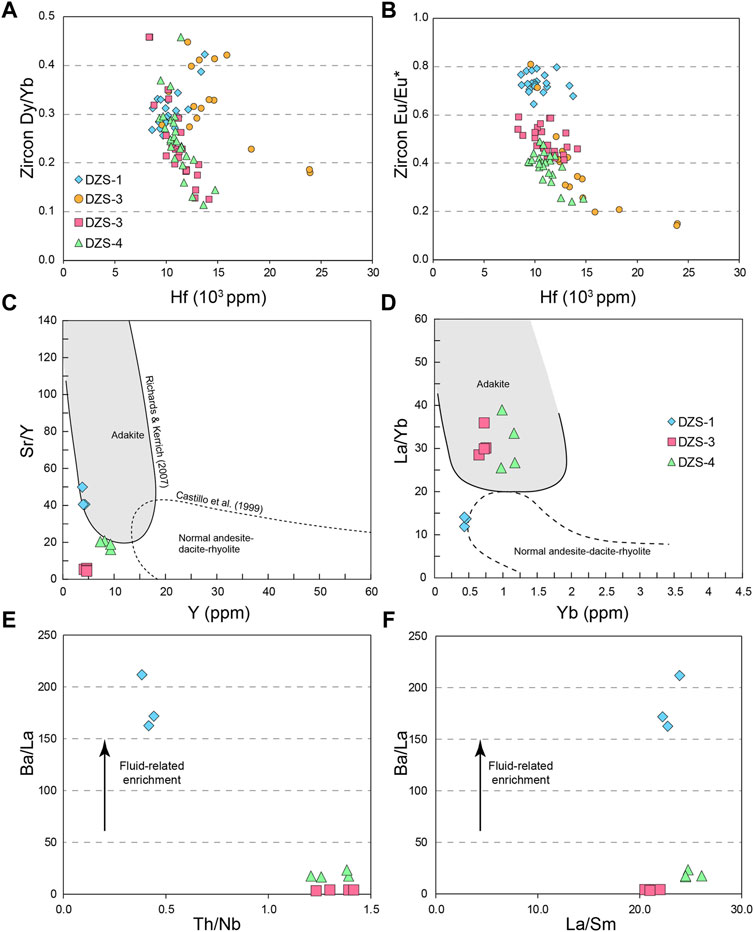
FIGURE 11. Zircon Dy/Yb (A) and Eu/Eu* (B) vs. Hf contents for the studied magmatic rocks. (C) Sy/Y vs. Y plot. The adakite and normal andesite-dacite-rhyolite fields are from Richards and Kerrich (2007) and Castillo et al. (1999), respectively. (D) La/Yb vs. Yb plot. Ba/La vs. Th/Nb (E) and La/Sm (F) plots. See the text for explanation.
The effects of magma mixing, if it occurs, rely on the composition of the mixing end-members. The relatively depleted features of LREE require at least one end-member of REE-depleted melt, most likely the depleted mantle source. However, the low Mg# numbers (<30), together with low Ni and Co concentrations (<2 ppm) imply that the mantle component is not involved in the magma genesis for the quartz porphyry. Therefore, the quartz porphyry was probably generated by the remelting of the REE-depleted lower crust at around 120 Ma.
5.1.2 Rhyolite porphyry (DZS-3)
The rhyolite porphyry also features extremely low Mg# values and low Ni and Co concentrations (Figure 10A; Table 1), ruling out the possibility of the involvement of the mantle component. These rocks show obvious Sr, P, and Ti depletion. Moreover, they are characterized by negative Eu anomalies and listric-shaped REE patterns (Figure 6A). These geochemical features imply that fractionation crystallization plays an important role in generating rhyolite porphyry. As shown in Figure 10B, whole-rock Dy/Yb ratios remain consistent with increasing Rb/Sr ratios, although the Rb/Sr ratios only show slight variation within samples. Zircon trace elements likely reflect more clear trends. The Zircon Dy/Yb ratio decreases from 0.45 to 0.1 when the Hf concentrations in zircon increase from 7,000 to 15,000 ppm (Figure 11A). Eu/Eu* values in zircon also show a decreasing trend with increasing Hf content (Figure 11B). This evidence supports the fractionation crystallization of amphibole and plagioclase. The amphibole incorporates more MREE (e.g., Dy) compared to HREE (e.g., Yb), thus leading to higher Dy/Yb values in the remnant melt (Davidson et al., 2007). Hafnium is an incompatible element during magmatic differentiation and its concentration will increase in the melt with progressive crystallization (Rollinson, 1993), which can be recorded in the co-crystallized zircon. In the current case, Dy/Yb decreases with increasing Hf content in zircon, implying amphibole fractionation. The negative Eu anomaly and decreasing zircon Eu/Eu* trend along with rising Hf concentrations reflect plagioclase fractionation because Eu preferentially partitions into plagioclase and replaces Ca in the lattice site under most geological environments.
Negative zircon Hf isotopes (−18.3 to −20.0) and the Archean two-stage Hf model ages suggest that the rhyolite porphyry is derived from the old crust source, consistent with the ancient nature of the NCC (Liu et al., 1992). Therefore, the rhyolite porphyry was derived from the melting of the ancient lower crust and experienced amphibole and plagioclase fractionation during magmatic evolution.
5.1.3 Dazeshan biotite granite (DZS-4)
The Dazeshan biotite granite shares overall similar geochemical features to those of the rhyolite porphyry in both REE pattern and spider diagram (Figure 6). Zircon trace elements and Hf isotopic compositions were almost identical (Figures 8–9, 11). Therefore, the origin of Dazeshan biotite granite was certainly the same as that of the rhyolite porphyry (crust anatexis with limited mantle contribution). The minor differences in REE and Sr concentrations were likely the result of discrepancies in differentiation processes between these two intrusions. As noted above, the negative Eu anomaly in the PM-normalized REE pattern and low zircon Eu/Eu* values indicated plagioclase fractionation. The listric-shaped REE pattern and decreasing zircon Dy/Yb values with rising Hf concentrations reflect amphibole-dominated fractionation. Therefore, it is reasonable to conclude that the Dazeshan biotite granite is derived from the partial melting of the lower crust with subsequent fractionation crystallization.
5.2 Multiple reworking events of the NCC lower crust
Previous studies have shown that the widely distributed Jurassic Linglong granite in the Jiaodong Penisular has adakite-like geochemical signatures with high Sr/Y and La/Yb ratios, which were considered to be the result of the melting of the thickened lower crust at the garnet stability field (Ma et al., 2013; Yang et al., 2017). The geodynamic model for the crustal thickening is probably related to the subduction of the Paleo-Pacific plate (Wu et al., 2005b; Ma et al., 2013). This left a residue with large portions of garnet in the lower crust, which by inference should be depleted of LILE and LREE due to the prior melt extraction. The crust-mantle interaction was strikingly extensive during the Early Cretaceous (∼130 Ma) given the widespread granitic and/or mafic magmatism and extensional deformation-related sedimentary basins (Wu et al., 2005b; Cai et al., 2013; Yang et al., 2018a, b; Meng et al., 2019). Furthermore, the ∼130 Ma granitic rocks in Jiaodong (e.g., Guojialing pluton), which also show adakite-like characters, were interpreted to be derived either from the melting of the delaminated eclogitic crust (Hou et al., 2007) or multi-stage magma mixing between Archean lower crust derived felsic magma and mafic lower crust-derived dioritic magma (Jiang et al., 2016).
The three intrusions are emplaced at around 120 Ma based on the zircon U-Pb ages obtained in the present study (Figure 7). These magmatic rocks were derived from the melting of the ancient lower crust of the NCC, as discussed in the previous section. The strontium concentrations for these samples (<200 ppm) are not as high as those in the Linglong and Guojialing magmatic rocks reported by Yang et al. (2012) (>300 ppm). Consequently, the Sr/Y values of the quartz porphyry, rhyolite porphyry, and the Dazeshan biotite granite are low (Figure 11C), unlike the Jurassic and Early Cretaceous granitoids in Jiaodong. However, the La/Yb ratios are high and plot within the “adakite field” (Figure 11D) (Condie, 2005; Castillo, 2006). This antinomy can be explained by the dominant crystal fractionation of amphibole and plagioclase during the magmatic evolution. As mentioned above, limited crystal fractionation occurred in the parent magma of the quartz porphyry. Therefore, the Sr/Y and La/Yb ratios probably represent its original character in these magmatic rocks. The low Sr/Y and La/Yb ratios (<50 and <15, respectively; Figures 11C, D), imply that little or no garnet remained as a residue phase in the source. Thus, the quartz porphyry may have been derived from a shallow crustal level rather than a thickened lower crust (>50 km) as in Linglong granite. Fluid flux played an important role in inducing the melting reaction because the Ba/La ratios for these samples are elevated (Figures 11E, F). Barium is a fluid mobile element, while the La is less so (Kessel et al., 2005). Therefore, the subduction-related fluid carried more Ba than La and generated high Ba/La ratios in the resultant magma.
The rhyolite porphyry and Dazeshan biotite granite, however, experienced amphibole + plagioclase fractionation, as evidenced by the geochemical data. Both suites have low Sr/Y but high La/Yb ratios. We propose that the plagioclase fractionation depleted the Sr concentration in the remnant melt but did not affect the La or Yb contents of the magma. Garnet, which retains most of the Y and Yb in the source, should have been stable when the parent magma of the rhyolite porphyry and the Dazeshan biotite granite formed. Under such a scenario, these two suites were probably generated by melting of a thicker crust compared to the quartz porphyry. These two suites were most likely formed by dehydration melting considering the low Ba/La but high Th/Nb and La/Sm ratios in these samples. Thorium is more incompatible than Nb while La is more incompatible than Sm; thus, Th/Nb and La/Sm ratios would be promoted during dehydration melting when no external element is introduced into the system.
Therefore, the melting may have occurred at different crustal levels at around 120 Ma, with distinct triggers. The fluid responsible for the fluxed melting very likely originated from the upwelling mafic magma, which was ultimately sourced from the dehydration of the subducted Paleo-Pacific plate (Windley et al., 2010; Geng et al., 2019). The dehydration melting in the case of rhyolite porphyry and the Dazeshan biotite granite may have been induced by heat loss from the underplated mafic magma. Regardless of fluid fluxed or dehydration melting, this change required a deeper source of heat and fluid. This required water-rich mafic magma, among which lithosphere mantle-derived magma is a possible candidate (Geng et al., 2019).
6 Conclusion
The results of this study reveal that the magmatic rocks in the Dazeshan region have zircon ages of ∼120 Ma and were generated by crustal melting; however, they experienced different melting/differentiation processes. The quartz porphyry was formed by fluid-mediated melting without apparent crystal crystallization. The rhyolite porphyry and the Dazeshan biotite granite were produced by dehydration melting and underwent amphibole and plagioclase fractionation, as indicated by whole-rock and zircon trace element data. Our results imply that, in the Early Cretaceous, the lower crust of the NCC was reworked multiple times in different ways. The driving force for the reworking probably invoked the underplating of water-rich mafic magma under an extensional regime (Li et al., 2012), which not only provided fluid to generate the quartz porphyry but also released heat to promote the dehydration melting of the lower crust to form the rhyolite porphyry and biotite granite.
Data availability statement
The original contributions presented in the study are included in the article/Supplementary Material. Further inquiries can be directed to the corresponding author.
Author contributions
LD collected samples, performed whole-rock and zircon geochemical analyses, and wrote the manuscript. XB drew the maps and figures. CD performed the data processing. ZY reviewed and edited the manuscript.
Funding
This study was financially supported by the National Natural Science Foundation of China-Shandong Joint Fund Program (U2006201), the National Key Research and Development Project of China (2016YFC0600305), and Fundamental Research Funds for the Central Universities (FRF-TP-20-042A1).
Acknowledgments
We express our sincere thanks to Li Huawei, Yu Chao, and Zhou Limin for their help with the LA-(MC)-ICPMS zircon U-Pb dating and Hf isotope analyses, and to Zhou Yiwei and Liu Yanhong for their help with the whole-rock major element analyses.
Conflict of interest
CD was employed in the Postdoctoral Programme of the Guosen Securities company.
The remaining authors declare that the research was conducted in the absence of any commercial or financial relationships that could be construed as a potential conflict of interest.
Publisher’s note
All claims expressed in this article are solely those of the authors and do not necessarily represent those of their affiliated organizations, or those of the publisher, the editors, and the reviewers. Any product that may be evaluated in this article, or claim that may be made by its manufacturer, is not guaranteed or endorsed by the publisher.
References
Brown, M. (2013). Granite: From Genesis to emplacement. Geol. Soc. Am. Bull. 125, 1079–1113. doi:10.1130/b30877.1
Cai, Y. C., Fan, H. R., Santosh, M., Liu, X., Hu, F. F., Yang, K. F., et al. (2013). Evolution of the lithospheric mantle beneath the southeastern North China Craton: Constraints from mafic dikes in the Jiaobei terrain. Gondwana Res. 24, 601–621. doi:10.1016/j.gr.2012.11.013
Cao, K., Yang, Z. M., White, N. C., and Hou, Z. Q. (2022). Generation of the giant porphyry Cu-Au deposit by repeated recharge of mafic magmas at Pulang in eastern Tibet. Econ. Geol. 117, 57–90. doi:10.5382/econgeo.4860
Castillo, P. R. (2006). An overview of adakite petrogenesis. Chin. Sci. Bull. 51, 257–268. doi:10.1007/s11434-006-0257-7
Castillo, P. R., Janney, P. E., and Solidum, R. U. (1999). Petrology and geochemistry of Camiguin Island, southern Philippines: Insights to the source of adakites and other lavas in a complex arc setting. Contributions mineralogy petrology 134, 33–51. doi:10.1007/s004100050467
Chappell, B. (2004). Towards a unified model for granite Genesis. Earth Environ. Sci. Trans. R. Soc. Edinb. 95, 1–10. doi:10.1017/s0263593300000870
Charles, N., Gumiaux, C., Augier, R., Chen, Y., Faure, M., Lin, W., et al. (2012). Metamorphic core complex dynamics and structural development: Field evidences from the Liaodong Peninsula (China, East Asia). Tectonophysics 560-561, 22–50. doi:10.1016/j.tecto.2012.06.019
Chen, J. F., Xie, Z., Zhang, X. D., Zhou, T. X., Park, Y. S., Ahn, K. S., et al. (2003). U-Pb zircon ages for a collision-related K-rich complex at Shidao in the Sulu ultrahigh pressure terrane, China. Geochem. J. 37, 35–46. doi:10.2343/geochemj.37.35
Chen, L., Tao, W., Zhao, L., and Zheng, T. (2008). Distinct lateral variation of lithospheric thickness in the Northeastern North China Craton. Earth Planet. Sci. Lett. 267, 56–68. doi:10.1016/j.epsl.2007.11.024
Clemens, J. D., Stevens, G., and Bryan, S. E. (2020). Conditions during the formation of granitic magmas by crustal melting-hot or cold; drenched, damp or dry? Earth-Science Rev. 200, 102982. doi:10.1016/j.earscirev.2019.102982
Condie, K. C. (2005). TTGs and adakites: Are they both slab melts? Lithos 80, 33–44. doi:10.1016/j.lithos.2003.11.001
Davidson, J., Turner, S., Handley, H., Macpherson, C., and Dosseto, A. (2007). Amphibole “sponge” in arc crust? Geol. 35, 787–790. doi:10.1130/g23637a.1
DePaolo, D. J. (1981). Trace element and isotopic effects of combined wallrock assimilation and fractional crystallization. Earth Planet. Sci. Lett. 53, 189–202. doi:10.1016/0012-821x(81)90153-9
Gao, P., Zheng, Y. F., and Zhao, Z. F. (2016). Experimental melts from crustal rocks: A lithochemical constraint on granite petrogenesis. Lithos 266–267, 133–157. doi:10.1016/j.lithos.2016.10.005
Gao, S., Rudnick, R. L., Yuan, H. L., Liu, X. M., Liu, Y. S., Xu, W. L., et al. (2004). Recycling lower continental crust in the North China craton. Nature 432, 892–897. doi:10.1038/nature03162
Geng, X., Foley, S. F., Liu, Y., Wang, Z., Hu, Z., and Zhou, L. (2019). Thermal-chemical conditions of the North China Mesozoic lithospheric mantle and implication for the lithospheric thinning of cratons. Earth Planet. Sci. Lett. 516, 1–11. doi:10.1016/j.epsl.2019.03.012
Goss, S. C., Wilde, S. A., Wu, F., and Yang, J. (2010). The age, isotopic signature and significance of the youngest mesozoic granitoids in the Jiaodong terrane, Shandong province, north China craton. Lithos 120, 309–326. doi:10.1016/j.lithos.2010.08.019
Hou, M. L., Jiang, Y. H., Jiang, S. Y., Ling, H. F., and Zhao, K. D. (2007). Contrasting origins of late mesozoic adakitic granitoids from the northwestern Jiaodong Peninsula, east China: Implications for crustal thickening to delamination. Geol. Mag. 144, 619–631. doi:10.1017/s0016756807003494
Jahn, B. M., and Zhang, Z. Q. (1984). Archean granulite gneisses from eastern Hebei province, China: Rare Earth geochemistry and tectonic implications. Contr. Mineral. Pet. 85, 224–243. doi:10.1007/bf00378102
Jiang, P., Yang, K. F., Fan, H. R., Liu, X., Cai, Y. C., and Yang, Y. H. (2016). Titanite-scale insights into multi-stage magma mixing in early cretaceous of NW Jiaodong terrane, north China craton. Lithos 258–259, 197–214. doi:10.1016/j.lithos.2016.04.028
Kessel, R., Schmidt, M. W., Ulmer, P., and Pettke, T. (2005). Trace element signature of subduction-zone fluids, melts and supercritical liquids at 120–180 km depth. Nature 437, 724–727. doi:10.1038/nature03971
Le Bas, M. J., Le Maitre, R. W., Streckeisen, A., and Zanettin, B. (1986). A chemical classification of volcanic rocks based on the total alkali – silica diagram. J. Petrology 27, 745–750. doi:10.1093/petrology/27.3.745
Lee, C. T. A., Luffi, P., and Chin, E. J. (2011). Building and destroying continental mantle. Annu. Rev. Earth Planet. Sci. 39, 59–90. doi:10.1146/annurev-earth-040610-133505
Li, X. C., Fan, H. R., Santosh, M., Hu, F. F., Yang, K. F., Lan, T. G., et al. (2012). An evolving magma chamber within extending lithosphere: An integrated geochemical, isotopic and zircon U-Pb geochronological study of the Gushan granite, eastern North China Craton. J. Asian Earth Sci. 50, 27–43. doi:10.1016/j.jseaes.2012.01.016
Li, X. H., Fan, H. R., Hu, F. F., Hollings, P., Yang, K. F., and Liu, X. (2019). Linking lithospheric thinning and magmatic evolution of late Jurassic to early cretaceous granitoids in the Jiaobei Terrane, southeastern North China Craton. Lithos 324–325, 280–296. doi:10.1016/j.lithos.2018.11.022
Liu, D. Y., Nutman, A. P., Compston, W., Wu, J. S., and Shen, Q. H. (1992). Remnants of ≥3800 Ma crust in the Chinese part of the Sino-Korean craton. Geol. 20, 339–342. doi:10.1130/0091-7613(1992)020<0339:romcit>2.3.co;2
Liu, Y., Gao, S., and Tingchuan, L. (1999). Geochemistry of granulites in north China craton: Implications for the composition of archean lower crus. Geology-Geochemistry 27, 40–46.
Liu, Y., Gao, S., Yuan, H., Zhou, L., Liu, X., Wang, X., et al. (2004). U-Pb zircon ages and Nd, Sr, and Pb isotopes of lower crustal xenoliths from north China craton: Insights on evolution of lower continental crust. Chem. Geol. 211, 87–109. doi:10.1016/j.chemgeo.2004.06.023
Liu, Y. S., Gao, S., Jin, S. Y., Hu, S. H., Sun, M., Zhao, Z. B., et al. (2001). Geochemistry of lower crustal xenoliths from neogene hannuoba basalt, north China craton: Implications for petrogenesis and lower crustal composition. Geochimica Cosmochimica Acta 65, 2589–2604. doi:10.1016/s0016-7037(01)00609-3
Ma, L., Jiang, S. Y., Dai, B. Z., Jiang, Y. H., Hou, M. L., Pu, W., et al. (2013). Multiple sources for the origin of Late Jurassic Linglong adakitic granite in the Shandong Peninsula, eastern China: Zircon U-Pb geochronological, geochemical and Sr–Nd–Hf isotopic evidence. Lithos 162–163, 251–263. doi:10.1016/j.lithos.2013.01.009
Ma, L., Jiang, S. Y., Hofmann, A. W., Dai, B. Z., Hou, M. L., Zhao, K. D., et al. (2014). Lithospheric and asthenospheric sources of lamprophyres in the Jiaodong Peninsula: A consequence of rapid lithospheric thinning beneath the north China craton? Geochimica Cosmochimica Acta 124, 250–271. doi:10.1016/j.gca.2013.09.035
McDonough, W. F., and Sun, S. S. (1995). The composition of the Earth. Chem. Geol. 120, 223–253. doi:10.1016/0009-2541(94)00140-4
Meng, Q. R., Wu, G. L., Fan, L. G., and Wei, H. H. (2019). Tectonic evolution of early Mesozoic sedimentary basins in the North China block. Earth-Science Rev. 190, 416–438. doi:10.1016/j.earscirev.2018.12.003
Menzies, M., Xu, Y., Zhang, H., and Fan, W. (2007). Integration of geology, geophysics and geochemistry: A key to understanding the north China craton. Lithos 96, 1–21. doi:10.1016/j.lithos.2006.09.008
Peccerillo, A., and Taylor, S. R. (1976). Geochemistry of eocene calc-alkaline volcanic rocks from the Kastamonu area, Northern Turkey. Contr. Mineral. Pet. 58, 63–81. doi:10.1007/bf00384745
Rapp, R. P., and Watson, E. B. (1995). Dehydration melting of metabasalt at 8–32 kbar: Implications for continental growth and crust-mantle recycling. J. Petrology 36, 891–931. doi:10.1093/petrology/36.4.891
Rapp, R., Shimizu, N., Norman, M., and Applegate, G. (1999). Reaction between slab-derived melts and peridotite in the mantle wedge: Experimental constraints at 3.8 GPa. Chem. Geol. 160, 335–356. doi:10.1016/s0009-2541(99)00106-0
Richards, J. P., and Kerrich, R. (2007). Special paper: Adakite-like rocks: Their diverse origins and questionable role in metallogenesis. Econ. Geol. 102, 537–576. doi:10.2113/gsecongeo.102.4.537
Rollinson, H. R. (1993). Using geochemical data: Evaluation, presentation, interpretation. Singapore: Longman Singapore Publishers (Pte) Ltd.
Sen, C., and Dunn, T. (1994). Dehydration melting of a basaltic composition amphibolite at 1.5 and 2.0 GPa: Implications for the origin of adakites. Contr. Mineral. Pet. 117, 394–409. doi:10.1007/bf00307273
Soesoo, A. (2000). Fractional crystallization of mantle-derived melts as a mechanism for some I-type granite petrogenesis: An example from lachlan fold belt, Australia. J. Geol. Soc. Lond. 157, 135–149. doi:10.1144/jgs.157.1.135
Song, M.-C., Li, J., Yu, X.-F., Song, Y.-X., Ding, Z.-J., and Li, S.-Y. (2021). Metallogenic characteristics and tectonic setting of the Jiaodong gold deposit, China. Solid Earth Sci. 6 (4), 385–405. doi:10.1016/j.sesci.2021.07.002
Sun, S.-s., and McDonough, W. F. (1989). “Chemical and isotopic systematics of oceanic basalts: Implications for mantle composition and processes,” in Magmatism in the ocean basins. Editors D. Saunders, and M. J. Norry (London: Geological Society), 313–345.
Thompson, A. B., Matile, L., and Ulmer, P. (2002). Some thermal constraints on crustal assimilation during fractionation of hydrous, mantle-derived magmas with examples from central alpine batholiths. J. Petrology 43, 403–422. doi:10.1093/petrology/43.3.403
Weinberg, R. F., and Hasalová, P. (2015). Water-fluxed melting of the continental crust: A review. Lithos 212–215, 158–188. doi:10.1016/j.lithos.2014.08.021
Whitney, D. L., and Evans, B. W. (2010). Abbreviations for names of rock-forming minerals. Am. mineralogist 95, 185–187. doi:10.2138/am.2010.3371
Windley, B. F., Maruyama, S., and Xiao, W. J. (2010). Delamination/thinning of sub-continental lithospheric mantle under Eastern China: The role of water and multiple subduction. Am. J. Sci. 310, 1250–1293. doi:10.2475/10.2010.03
Wu, F., Yang, J., Xu, Y., Wilde, S. A., and Walker, R. J. (2019). Destruction of the north China craton in the mesozoic. Annu. Rev. Earth Planet. Sci. 47, 173–195. doi:10.1146/annurev-earth-053018-060342
Wu, F. Y., Lin, J.-Q., Wilde, S. A., Zhang, X. O., and Yang, J. H. (2005a). Nature and significance of the Early Cretaceous giant igneous event in eastern China. Earth Planet. Sci. Lett. 233, 103–119. doi:10.1016/j.epsl.2005.02.019
Wu, F. Y., Yang, J. H., Wilde, S. A., and Zhang, X. O. (2005b). Geochronology, petrogenesis and tectonic implications of Jurassic granites in the Liaodong Peninsula, NE China. Chem. Geol. 221, 127–156. doi:10.1016/j.chemgeo.2005.04.010
Wu, F. Y., Yang, Y. H., Xie, L. W., Yang, J. H., and Ping, X. (2006). Hf isotopic compositions of the standard zircons and baddeleyites used in U–Pb geochronology. Chem. Geol. 234, 105–126. doi:10.1016/j.chemgeo.2006.05.003
Wu, Y., and Zheng, Y. (2013). Tectonic evolution of a composite collision orogen: An overview on the Qinling-Tongbai-Hong'an-Dabie-Sulu orogenic belt in central China. Gondwana Res. 23, 1402–1428. doi:10.1016/j.gr.2012.09.007
Xu, Y., Li, H., Pang, C., and He, B. (2009). On the timing and duration of the destruction of the North China Craton. Sci. Bull. (Beijing). 54, 3379–3396. doi:10.1007/s11434-009-0346-5
Xue, D., Wang, H., Liu, Y., Xie, L., and Shen, P. (2017). An improved procedure for the determination of ferrous iron mass fraction in silicate rocks using a schlenk line-based digestion apparatus to exclude oxygen. Geostand. Geoanal. Res. 41, 411–425. doi:10.1111/ggr.12164
Yang, F., Santosh, M., and Kim, S. W. (2018a). Mesozoic magmatism in the eastern North China Craton: Insights on tectonic cycles associated with progressive craton destruction. Gondwana Res. 60, 153–178. doi:10.1016/j.gr.2018.04.003
Yang, F., Santosh, M., and Tang, L. (2018b). Extensive crustal melting during craton destruction: Evidence from the Mesozoic magmatic suite of Junan, eastern North China Craton. J. Asian Earth Sci. 157, 119–140. doi:10.1016/j.jseaes.2017.07.010
Yang, J., Wu, F., Chung, S., Wilde, S. A., and Chu, M. (2004). Multiple sources for the origin of granites: Geochemical and Nd/Sr isotopic evidence from the gudaoling granite and its mafic enclaves, northeast China. Geochimica Cosmochimica Acta 68, 4469–4483. doi:10.1016/j.gca.2004.04.015
Yang, K. F., Fan, H. R., Santosh, M., Hu, F. F., Wilde, S. A., Lan, T. G., et al. (2012). Reactivation of the Archean lower crust: Implications for zircon geochronology, elemental and Sr–Nd–Hf isotopic geochemistry of late Mesozoic granitoids from northwestern Jiaodong Terrane, the North China Craton. Lithos 146–147, 112–127. doi:10.1016/j.lithos.2012.04.035
Yang, L. Q., Dilek, Y., Wang, Z. L., Weinberg, R. F., and Liu, Y. (2017). Late Jurassic, high Ba–Sr Linglong granites in the Jiaodong Peninsula, east China: Lower crustal melting products in the eastern north China craton. Geol. Mag. 155, 1040–1062. doi:10.1017/s0016756816001230
Zhai, M., Guo, J., and Liu, W. (2001). An exposed cross-section of early Precambrian continental lower crust in North China craton. Phys. Chem. Earth Part A Solid Earth Geodesy 26, 781–792. doi:10.1016/s1464-1895(01)00127-2
Zhang, Y., Li, J., Zhang, T., Dong, S., and Yuan, J. (2008). Cretaceous to Paleocene Tectono-Sedimentary Evolution of the Jiaolai basin and the Contiguous Areas of the Shandong Peninsula(North China) and Its Geodynamic Implications. Acta Geol. Sin. 82, 1229–1257.
Zhao, G. (2001). Palaeoproterozoic assembly of the North China craton. Geol. Mag. 138, S0016756801005040–S0016756801005091. doi:10.1017/s0016756801005040
Zhao, G., Sun, M., Wilde, S. A., and Li, S. (2005). Late Archean to Paleoproterozoic evolution of the North China Craton: key issues revisited. Precambrian Res. 136, 177–202. doi:10.1016/j.precamres.2004.10.002
Zheng, Y. F., and Gao, P. (2021). The production of granitic magmas through crustal anatexis at convergent plate boundaries. Lithos 402–403, 106232. doi:10.1016/j.lithos.2021.106232
Keywords: Jiaodong, North China Craton, granite, petrogenesis, crustal reworking
Citation: Dong L, Yang Z, Bai X and Deng C (2023) Generation of the Early Cretaceous granitoid in the Dazeshan region, Jiaodong Peninsula: Implications for the crustal reworking in the North China Craton. Front. Earth Sci. 10:1083608. doi: 10.3389/feart.2022.1083608
Received: 29 October 2022; Accepted: 05 December 2022;
Published: 30 January 2023.
Edited by:
Fei Xue, Hohai University, ChinaReviewed by:
Sam Uthup, National Taiwan Normal University, TaiwanJian Li, Shandong University of Technology, China
Chao Zhao, Chang’an University, China
Copyright © 2023 Dong, Yang, Bai and Deng. This is an open-access article distributed under the terms of the Creative Commons Attribution License (CC BY). The use, distribution or reproduction in other forums is permitted, provided the original author(s) and the copyright owner(s) are credited and that the original publication in this journal is cited, in accordance with accepted academic practice. No use, distribution or reproduction is permitted which does not comply with these terms.
*Correspondence: Leilei Dong, bGVpbGVpZG9uZ0B1c3RiLmVkdS5jbg==