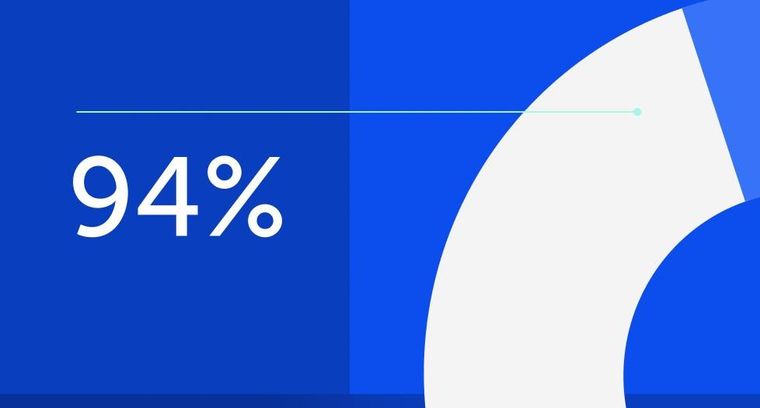
94% of researchers rate our articles as excellent or good
Learn more about the work of our research integrity team to safeguard the quality of each article we publish.
Find out more
PERSPECTIVE article
Front. Earth Sci., 28 November 2022
Sec. Structural Geology and Tectonics
Volume 10 - 2022 | https://doi.org/10.3389/feart.2022.1046652
This article is part of the Research TopicWomen in Science: Structural Geology and Tectonics 2022View all 6 articles
The outer shells of solid planetary bodies have been all subject to deformation during their history. The nature and style of deformation strictly depends on the evolution of each planetary body. Earth’s lithospheric style of deformation is related to plate tectonics, but it turns out to be a unique case in our Solar System. How can we study the tectonics of other planetary bodies? How and why do planetary lithospheres deform, and what are the implications of these processes? These are the driving questions for investigations of modern planetary structural geology. The improvement in technology, machine learning analyses, and quality and quantity of planetary space mission data give us the chance to deepen our grasp in planetary surface deformation. The lack of micro-to-local scale information that is so crucial in Earth structural geology studies has driven planetary structural geology to advance pioneering methods to study crustal deformation. Conversely, the study of old deformed surfaces such as those of Mercury, the Moon and Mars will deepen our grasp on the early evolution of Earth tectonics.
Deformation can be recorded on any solid body, rock or ice, where stresses exceed the strength of the lithosphere or cryosphere, respectively. The study of deformation and resultant landforms on other planets (and technically Earth) is termed ‘planetary tectonics’. Although we tend to use Earth’s structures as a framework for understanding how these landforms develop, this does not imply other planets deform due to the translation of multiple tectonic plates across their surfaces. However, it does mean that geologic processes on some scale must be or have been active and translating stresses to the outer shells (Stern et al., 2018) of those planets at some point present or past. Our goal is largely to reconstruct the timing, extent, and magnitudes of those geologic processes.
Many tectonic landforms that would be useful are destroyed or heavily modified by asteroid impacts; however, preserved features are subjected to minimal erosion (Figure 1). Practicing planetary structural geology is challenging because so much of its focus is on understanding deformation that occurred when planets were more geologically active, in some cases, billions of years ago with data at lower resolutions than Earth and no ability to access one’s field area. Still, data resolutions are improving, and toolsets developed for Earth are being adapted for planetary use. Thus, our field has exciting opportunities for future research.
FIGURE 1. Imagery and simple maps of normal (A), reverse (B), and strike slip (C) planetary structures. The Rima Hyginus lunar graben system in (A) demonstrates the connection between pit volcanism and extension, with pit and fault traces in pink and black linework (Lunar Orbiter Laser Altimeter topography atop Lunar Reconnaissance Orbiter Camera Basemap near 5.8° E, 8.4° N). (B) Carnegie Rupes, a large thrust on Mercury, exemplifies how faults and craters can interact (USGS topography atop global monochrome mosaic near 68.9° W, 58.2° N). (C) Strike slip faulting on Europa illustrates the tectonics of icey bodies (Galileo Orbiter imagery in the Northern Hemisphere).
Planetary structural geology has been constantly evolving as a field since the 1960s and 1970s when orbiters of the Mariner program returned the first images from the terrestrial planets. Initial observations aimed to explore tectonics for tectonics’ sake. Basic characterization like styles and amounts of deformation (Golombek and McGill, 1983; Banerdt et al., 1992; Watters, 2004), orientations of landforms (Cordell and Strom, 1977; Melosh, 1980; Watters and Robinson, 1999), and geological connection between tectonism and other processes were investigated (Strom et al., 1975; Dzurisin, 1978; Solomon, 1978; Wise et al., 1979; Melosh and McKinnon, 1988). These observational studies encouraged detailed descriptions of tectonism-especially as data resolution and diversity increased with new missions. Today, planetary structural geologists consider three central questions: 1) How do lids deform? 2) Why do lids deform? and 3) What are the implications of deformation? While the first question dominated previous studies and is still necessary today, the latter questions motivate new work.
Studies of fault geometry are prerequisite for understanding magnitudes of lid deformation. While normal faults largely reveal their planar geometries at the surface, thrust fault breaks are not always clear, with blind thrusts remaining hidden. Positive, linear relief associated with thrust fault-related landforms (Figure 1B) has been used to constrain modeling investigations that compare fault geometries and slip magnitudes. Stresses and strains associated with planetary structures have been estimated using Coulomb Matlab software (Schultz and Watters, 2001; Watters, 2004; Toda et al., 2011), but a push toward 3D structural modeling can improve our ability to visualize the subsurface. The open-source tools GemPy, Loop, and Visual Karsys may allow for improved structural analysis. Some recent work has used Move Structural Geology Modeling Software, a package aimed at industry, to investigate landform geometries and in ways that explicitly consider deformational style and folding (e.g., fault bend folding, trishear, fault propagation folding Klimczak et al., 2018a; Herrero-Gil et al., 2020; Crane, 2020).
Move software dramatically enhances our ability to investigate deformation (Figure 2). Landforms can be undeformed or unfolded to track strain accumulation. Stresses can be tracked at the surface and at depth. Many Move modules have yet to be applied to planetary landforms. The Fault Analysis and 3D Kinematic Modeling tools could be used to study normal and strike-slip faults (Figure 1A and Figure 1C) while the Fracture Modeling tool could analyze fracture networks that produce polygonal impact craters (crater cut in Figure 1B). Kinematic Modeling tools have already been used to reconstruct subsurface thrust faults such as the example shown in Figure 2, helping planetary geologists address the longstanding question of the relative importance of thick- and thin-skinned deformation (Anguita et al., 2001; Bethell et al., 2022). As with any package developed for Earth, rock properties and projections should be altered to reflect the surface properties of the body of interest and software documentation explored to understand the underlying assumptions of model computations.
FIGURE 2. Thrust fault-related landform on Mars displayed in Move software. Context camera imagery was draped over Mars Orbiter Laser Altimeter topography and fault surface breaks were mapped and fault planes generated. The transition from simple, single planar fault in the north (upper right) to complex, shallow multi-fault system in the south (lower left) demonstrates how morphological variability can be interpreted through subsurface modeling.
We infer mechanical properties from fault geometries. For example, thrust faults inferred to shallow into decollements (Crane and Klimczak, 2019a) and normal faults that imply listric geometries (Balbi et al., 2022) both represent fault geometries that suggest strength contrasts in layered materials at depth. Rheological layering may also be implied when an entire fault population shares topographic characteristics (Golombek, 1985) or when fault planes are assumed to root into the Brittle Ductile Transition (Schultz and Watters, 2001; Egea-Gonzalez et al., 2012; Watters et al., 2015). For example, trough systems on Asteroid 4 Vesta, interpreted as opening mode fractures, reveal that Vesta’s upper ∼55 km of lithosphere does not favor faulting (Cheng and Klimczak, 2022). Finite element models are often used to derive these depths, but displacement-length (D-L) scaling has also been a popular tool.
Fault planes grow elliptically, so as faults lengthen, they accumulate displacement from their centers (maximum) to their tips (minimum). Displacement results in topographic relief as faults propagate toward the surface, and since relief can be measured in planetary topographic datasets, displacement studies have been carried out on Mars, the Moon, Mercury, and Earth. The shape of D-L profiles (plotting displacement over the length of the fault) can be diagnostic of barriers to fault propagation at depth and linkage (Schultz et al., 2006; Polit et al., 2009). Faults rarely occur in isolation. Recognizing their arrangements as systems permits interpretations of how they grow (Massironi et al., 2015). Often, D-L profiles are used to infer growth mechanisms and interaction between multiple faults. Earth analogue field studies have also been important for understanding linkage-particularly because the order and spatial extent of linkage can point to reasons for deformation (Klimczak et al., 2018a; Martin and Watters, 2022). Rheological changes can also occur laterally across a planet’s surface. Patterns in fault vergence (Roggon et al., 2017; Galluzzi et al., 2019) and geometry (Byrne et al., 2014) highlight those differences.
We may be interested in how lids deform, but with high resolution imagery and topography and geodatabases capable of holding and processing large amounts of data, many planetary structural geologists have turned our attention towards why lids deform.
Geologic histories of planets are not simple series of isolated events. Systematic approaches to understanding timing and extent of events are challenging. Volcanism, mantle upwelling and downwelling, initiating (and failing) plate tectonics, ice and water modifications, impacts, basin loading, orbital parameter changes, and polar wander are just a few of the processes which may affect the distribution of faults. Personally, both authors have found trying to parse specific geologic events to be relatively infuriating, but each may have some kind of fingerprint-a distinct way that faults may be affected-that would allow processes to be identified.
Locations of faults can be analyzed to differentiate whole body processes from local or regional processes, and fault trace orientation has been used in conjunction with fault location to provide additional constraints to formational mechanism hypotheses (Dombard and Hauck, 2008; King, 2008). For example, early imagery of Mercury showed a global population of thrust fault-related landforms (Cordell, 1977; Strom, 1979). This observation supported the hypothesis that global contraction was the primary culprit for global tectonics. More recent imagery mapped using Geographical Information Systems (GIS) has been used to construct rose diagrams showing regional trends—proof that other processes also affected the tectonic landscape of Mercury (Matsuyama and Nimmo, 2009; Watters et al., 2015; Crane and Klimczak, 2019b; Galluzzi et al., 2019). Rose diagrams also highlight patterns in landform orientation on the Moon and Mars.
Impacts often cut faults, so by isolating and analyzing only faults crosscut by impact craters from certain time periods, one might be able to observe trends in their locations, orientations, and magnitudes. This type of study would struggle with two limitations-chance and preservation. An old fault should be heavily crosscut, but it could have avoided the fate of being struck, appearing young. Alternatively, an old fault could be completely obliterated by impact cratering-not preserved at all. Either way, a study of ancient faulting would miss information conveyed through that fault. This is why it is critical that any faulting study looking to identify specific processes over any specific period of time conduct global investigations to construct a robust dataset. For example, it is crucial to regionally identify systems of faults rather than studying isolated fault segments in order to get reliable statistics on the relative age of deformation (e.g., Galluzzi et al., 2019; Giacomini et al., 2020).
On the other way round, faults can also crosscut older impacts (Figure 1B) providing hints on fault geometry, kinematics, and timing for both rocky (e.g., Galluzzi et al., 2015; Crane and Klimczak, 2017) and icy bodies (Pappalardo and Collins, 2005). Although planetary surfaces often lack this kind of kinematic indicator, when present, they can help with the assessment of regional stress fields to be compared to the global tectonic models (Galluzzi et al., 2019).
Machine learning may provide future avenues for identifying unique populations of tectonic landforms, including those that the human eye may have missed or mis-mapped during mapping (e.g. Schaaf and Bond, 2019; Donn et al., 2020). Studies might include identifying faults that are active via observed recent landslides (e.g., Katta et al., 2021), recognition of polygonal craters with rims reflecting underlying tectonic fabrics (Beddingfield and Cartwright, 2020), and quantification of populations based on morphological differences. As groups are differentiated, it may become possible to parse the underlying causes for regional and global tectonism. One such mechanism may be plate tectonics.
On Earth, plate collision results in thrusts linked by transforms. Plate collision boundaries are often reused as spreading centers when plates diverge due to strength contrasts between the thickened zone of collision juxtaposed against thin, undeformed lithosphere (Thomas, 2006; Blakey and Ranney, 2017; Clark et al., 2017; Copeland et al., 2017). Thus, elongated, long-lived fault zones separating terrains of different strength may indicate places where mantle convection maintained one principal direction of flow and where convecting mantle was rheologically tied to the upper lithosphere. We may be able to visually identify such zones (e.g., the Thaumasia region of Mars, the high-magnesium region’s north-eastern boundary on Mercury) but the more dispersed the process, the less likely visual assessment is to identify such fingerprints.
Tectonic patterns arising from the initiation and cessation of plate tectonics may be hidden within tectonic fabrics associated with later processes-especially if plate tectonics was confined to the early periods of planetary history. Crustal strength or density differences above underlying mantle are the only real prerequisite for subduction. Thus, one could imagine scenarios where Mars, a planet with a crustal dichotomy, developed a shell broken into two or more plates. Plate arrangements could explain topography and shape the Thaumasia Highlands or the magnetic patterns indicative of seafloor spreading discovered by Mars Global Surveyor in the 1990s.
Like intercontinental mountain ranges on Earth, any other planetary subduction zones (if they ever existed) are likely overprinted by later deformation, but to date, it remains unknown what that overprinting would look like and if the remnant signature of plate collision would be recognizable. New studies using ASPECT mantle convection modeling and supercomputing (Van Der Bogert et al., 2018) could pave the way for recognizing Martian mantle scars. While written for Earth applications, this code has many cookbooks (prepared base codes) that can be adapted for other planetary bodies and may allow us to investigate mountain building, subduction, and rifting on other worlds.
Models in ASPECT can quantify stresses and strains associated with processes, but we can also use the landforms themselves to estimate total strains. If each landform represents a certain amount of strain, then accounting for each landform can result in whole body estimates. One of the best ways to understand the true shortening, extension, or shearing associated with landforms is to measure such properties at Earth analogue sites.
Earth analogues are also useful when testing formation hypotheses for planetary structures. By locating features on Earth that look and share lithological characteristics with those features on other planets, the processes behind the formation of the landforms can be inferred. For example, Medicine Lake, California silicate domes may inform the mechanism behind the formation of silicate domes on the Moon (Fink and Anderson, 2017). In Steens Mountain, Oregon, table mountains appear similar in arrangement and morphology to mesas in the chaos regions of Mars (Bohanon and Crane, 2022). In Oregon, these features root within a weak layer, structurally connected to the presence of a quiet caldera, with active faulting and hot springs close by. Could these same processes have operated in the chaos terrains?
Because of our long history of plate tectonics, Earth’s crust is critically fractured (Zoback, 2010). These fractures provide conduits for volcanic material to migrate and critically link the processes volcanism and tectonism. Even introductory geology courses often ask students to notice that the majority of volcanoes are located at plate boundaries, which are simply glorified large, connected fault systems. On planets without functioning plate tectonics, can we make similar connections?
Mercury, the Moon, and Mars all have evidence of large effusive deposits likely emplaced by dikes, maybe multiple generations of dikes which can be thought of as filled, reactivated, vertical fractures. Dikes on Earth are sometimes observed to be sill-fed (e.g., Ferrar Large Igneous Province of Antartctica and the Chief Joseph Dike Swarm of the Columbia River Basalts (Muirhead et al., 2014; Morriss et al., 2020)). Thus, dikes and their propagation can help us explore regional stresses, rheological layering, and thermal history, even when a lithosphere is in a relative state of horizontal compression (Crane and Bohanon, 2021). Orientations and density of fracture networks can be explored through volcanic observations such vent size, location, and alignment (Klimczak et al., 2018b), lava tube injection direction (Torrese et al., 2021), and pit chain orientation and size (Figure 1A). However, more work should be done to understand intraplate volcanism as processes like thermal erosion, delamination, and underplating could all be important processes for Mars (Li et al., 2021) and other bodies. A casual observation at the brown cryovolcanoes in Figure 1C curiously shows that these features on the icy body of Europa are located atop tiny plates between (and not on) strike slip boundaries. Earth analogues are likely an important source for furthering our understanding of volcano-tectonic interplay (Sauro et al., 2019).
On Earth, many have hypothesized that the evolution of life is tied to the initiation and continuation of plate tectonics (Stern, 2016; Johnson and Van Kranendonk, 2019). Without these processes, life may exist in simpler forms in the subsurface of other bodies. Organisms may take hold in fractures and faults at depth, so understanding how these organisms thrive and how to identify their presence is an exciting new pathway for planetary structural research. These endoliths or chasmoendoliths, organisms that occupy rocks and rock fractures, respectively, when living or fossilized will only be observable at scales visible to rovers, landers, UAS, and future submersibles and human explorers. Current rovers are equipped with UV and infrared spectrometers which could detect organic signatures, but planetary structural geologists should work with biogeochemists and astrobiologists to take analogue tools in the field to characterize relationships between chemical signatures, appearance, and abundance of endoliths.
Humans will almost certainly use such toolsets to study sites for natural hazards potential, astrobiological implications, and to quantify the potential for habitability. Afterall, the aquifers of Mars will almost certainly be fracture-connected, not sedimentary aquifers and fracture connectivity quantification will be critical for describing the potential for gaining access to a usable water supply.
Observations of morphologically crisp tectonic landforms show that all terrestrial planets in our Solar System have been tectonically active in the geologically recent past (Watters et al., 2015; Stern et al., 2018), but decoding that tectonic activity requires deep understanding of what deformation the landforms represent. Planetary structural geologists aim to understand when, where, and why these planets are tectonically active and if their lids ever participated in more Earth-like tectonics. Fracture systems may also be our path to investigating life on other bodies as chasmoendoliths on Earth have been observed in extreme environmental settings. Nonetheless, Earth’s plate tectonics triggering mechanisms are still under investigation and understanding our planet’s early evolution is challenging because of the lack of information (especially from ancient uncontaminated outcrops). Thus, studying the tectonics of other planetary bodies (that often preserve their ancient surface) and investigating their structural frameworks is also crucial to reconstruct putative scenarios of Earth’s early evolution.
While this work excites the authors, we recognize how important it is to diversify our field with unique perspectives. We reject the idea of creative scarcity and welcome these underheard voices to our field. Our hope is that through developing more inclusive, collaborative research teams, planetary structural geology will advance in ways we cannot yet predict.
The original contributions presented in the study are included in the article/Supplementary Material, further inquiries can be directed to the corresponding author.
KC crafted the initial draft of the manuscript which was meaningfully enhanced and revised by VG.
The authors declare that the research was conducted in the absence of any commercial or financial relationships that could be construed as a potential conflict of interest.
All claims expressed in this article are solely those of the authors and do not necessarily represent those of their affiliated organizations, or those of the publisher, the editors and the reviewers. Any product that may be evaluated in this article, or claim that may be made by its manufacturer, is not guaranteed or endorsed by the publisher.
Anguita, F., Farelo, A. F., López, V., Mas, C., Muñoz-Espadas, M. J., Márquez, Á., et al. (2001). Tharsis dome, Mars: New evidence for Noachian-Hesperian thick-skin and Amazonian thin-skin tectonics. J. Geophys. Res. 106 (4), 7577–7589. doi:10.1029/2000JE001246
Balbi, E., Cianfarra, P., Ferretti, G., Crispini, L., and Tosi, S. (2022). Modelling the extensional tectonic setting of the Claritas Fossae. Vienna, Austria: EGU General Assembly. doi:10.5194/egusphere-egu22-7683
Banerdt, W. B., Golombek, M. P., and Tanaka, K. L. (1992). Stress and tectonics on Mars. Italy: Mars, 249–297.
Beddingfield, C. B., and Cartwright, R. J. (2020). Hidden tectonism on Miranda's Elsinore Corona revealed by polygonal impact craters. Icarus 343, 113687. doi:10.1016/j.icarus.2020.113687
Bethell, E. M., Ernst, R. E., and Samson, C. (2022). Analysis of venusian wrinkle ridge morphometry using stereo-derived topography: A case study from southern eistla regio. JGR. Planets 127 (5), e2021JE006879. doi:10.1029/2021JE006879
Blakey, R. C., and Ranney, W. D. (2017). Ancient landscapes of western north America: A geologic history with paleogeographic maps. Berlin, Germany: Springer.
Bohanon, A., and Crane, K. (2022). Structural and biological analysis of faults in basalts in Sheepshead Mountains, Oregon as an Earth analogue to Mars. Icarus 385, 115121. doi:10.1016/j.icarus.2022.115121
Byrne, P. K., Klimczak, C., Celâl Şengör, A. M., Solomon, S. C., Watters, T. R., Hauck, I. I., et al. (2014). Mercury’s global contraction much greater than earlier estimates. Nat. Geosci. 7 (4), 301–307. doi:10.1038/ngeo2097
Cheng, H. C. J., and Klimczak, C. (2022). Structural relationships in and around the Rheasilvia basin on Vesta. J. Struct. Geol. 161, 104677. doi:10.1016/j.jsg.2022.104677
Clark, J. D., Hurtado, J. M., Hiesinger, H., van der Bogert, C. H., and Bernhardt, H. (2017). Investigation of newly discovered lobate scarps: Implications for the tectonic and thermal evolution of the Moon. Icarus 298, 78–88. doi:10.1016/j.icarus.2017.08.017
Copeland, P., Currie, C. A., Lawton, T. F., and Murphy, M. A. (2017). Location, location, location: The variable lifespan of the Laramide orogeny. Geology 45 (3), 223–226. doi:10.1130/G38810.1
Cordell, B. M., and Strom, R. G. (1977). Global tectonics of Mercury and the moon. Phys. Earth Planet. Interiors 15 (2-3), 146–155. doi:10.1016/0031-9201(77)90027-9
Cordell, B. M. (1977). Tectonism and the interior of Mercury. United States: The University of Arizona.
Crane, K., and Bohanon, A. (2021). Dike propagation during global contraction: Making sense of conflicting stress histories on Mercury. Front. Earth Sci. (Lausanne). 9, 752864. doi:10.3389/feart.2021.752864
Crane, K. (2020). Structural interpretation of thrust fault-related landforms on Mercury using Earth analogue fault models. Geomorphology 369, 107366. doi:10.1016/j.geomorph.2020.107366
Crane, K. T., and Klimczak, C. (2019a). A 3-D structural model of the saddle mountains, yakima fold Province, Washington, USA: Implications for late tertiary tectonic evolution of the Columbia River flood basalt Province. Tectonophysics 766, 1–13. doi:10.1016/j.tecto.2019.05.015
Crane, K. T., and Klimczak, C. (2019b). Tectonic patterns of shortening landforms in Mercury's northern smooth plains. Icarus 317, 66–80. doi:10.1016/j.icarus.2018.05.034
Crane, K. T., and Klimczak, C. (2017). Timing and rate of global contraction on Mercury. Geophys. Res. Lett. 44 (7), 3082–3089. doi:10.1002/2017GL072711
Dombard, A. J., and Hauck, S. A. (2008). Despinning plus global contraction and the orientation of lobate scarps on Mercury: Predictions for MESSENGER. Icarus 198 (1), 274–276. doi:10.1016/j.icarus.2008.06.008
Donn, L., Beach, T., Schank, C., Inomatat, T., and Ortiz, A. (2020). Machine learning applications for landscape management and conservation: Using digital elevation data to model locations of natural and cultural features. Washington, D.C., United States: American Geophysical Union.
Dzurisin, D. (1978). The tectonic and volcanic history of Mercury as inferred from studies of scarps, ridges, troughs, and other lineaments. J. Geophys. Res. 83 (10), 4883–4906. doi:10.1029/JB083iB10p04883
Egea-González, I., Ruiz, J., Fernández, C., Williams, J. P., Márquez, Á., and Lara, L. M. (2012). Depth of faulting and ancient heat flows in the Kuiper region of Mercury from lobate scarp topography. Planet. Space Sci. 60 (1), 193–198. doi:10.1016/j.pss.2011.08.003
Fink, J. H., and Anderson, S. W. (2017). Emplacement of holocene silicic lava flows and domes at newberry, south sister, and Medicine Lake volcanoes, California and Oregon. United States: US Geological Survey. doi:10.3133/sir20175022I
Galluzzi, V., Di Achille, G., Ferranti, L., Popa, C., and Palumbo, P. (2015). Faulted craters as indicators for thrust motions on Mercury. Geol. Soc. Lond. Spec. Publ. 401 (1), 313–325. doi:10.1144/SP401.17
Galluzzi, V., Ferranti, L., Massironi, M., Giacomini, L., Guzzetta, L., and Palumbo, P. (2019). Structural analysis of the Victoria quadrangle fault systems on Mercury: Timing, geometries, kinematics, and relationship with the high-Mg region. J. Geophys. Res. Planets 124 (10), 2543–2562. doi:10.1029/2019JE005953
Giacomini, L., Massironi, M., Galluzzi, V., Ferrari, S., and Palumbo, P. (2020). Dating long thrust systems on Mercury: New clues on the thermal evolution of the planet. Geosci. Front. 11 (3), 855–870. doi:10.1016/j.gsf.2019.09.005
Golombek, M. P. (1985). Fault type predictions from stress distributions on planetary surfaces: Importance of fault initiation depth. J. Geophys. Res. 90 (4), 3065–3074. doi:10.1029/JB090iB04p03065
Golombek, M. P., and McGill, G. E. (1983). Grabens, basin tectonics, and the maximum total expansion of the Moon. J. Geophys. Res. 88 (4), 3563–3578. doi:10.1029/JB088iB04p03563
Herrero-Gil, A., Ruiz, J., and Romeo, I. (2020). 3D modeling of planetary lobate scarps: The case of Ogygis Rupes, Mars. Earth Planet. Sci. Lett. 532, 116004. doi:10.1016/j.epsl.2019.116004
Johnson, C. M., and Van Kranendonk, M. J. (2019). Ancient life and plate tectonics Handbook of astrobiology. Boca Raton, Florida, United States: CRC Press. doi:10.1201/b22230-16
Katta, S., Paheding, S., Oommen, T., Rajaneesh, A., and Sajinkumar, K. S. (2021). Categorization of martian landslides from satellite imagery using vision transformer. Chicago: AGU Fall Meeting.
King, S. D. (2008). Pattern of lobate scarps on Mercury’s surface reproduced by a model of mantle convection. Nat. Geosci. 1 (4), 229–232. doi:10.1038/ngeo152
Klimczak, C., Crane, K. T., Habermann, M. A., and Byrne, P. K. (2018b). The spatial distribution of Mercury's pyroclastic activity and the relation to lithospheric weaknesses. Icarus 315, 115–123. doi:10.1016/j.icarus.2018.06.020
Klimczak, C., Kling, C. L., and Byrne, P. K. (2018a). Topographic expressions of large thrust faults on Mars. JGR. Planets 123 (8), 1973–1995. doi:10.1029/2017JE005448
Li, Y., Weng, A., Xu, W., Zou, Z., Tang, Y., Zhou, Z., et al. (2021). Translithospheric magma plumbing system of intraplate volcanoes as revealed by electrical resistivity imaging. Geology 49 (11), 1337–1342. doi:10.1130/G49032.1
Martin, E. S., and Watters, T. R. (2022). Displacement-length scaling relations of nearside graben: Evidence of restricted normal faults on the moon. Icarus 388, 115215. doi:10.1016/j.icarus.2022.115215
Massironi, M., Di Achille, G., Rothery, D. A., Galluzzi, V., Giacomini, L., Ferrari, S., et al. (2015). Lateral ramps and strike-slip kinematics on Mercury. Geol. Soc. Lond. Spec. Publ. 401 (1), 269–290. doi:10.1144/SP401.16
Matsuyama, I., and Nimmo, F. (2009). Gravity and tectonic patterns of Mercury: Effect of tidal deformation, spin-orbit resonance, nonzero eccentricity, despinning, and reorientation. J. Geophys. Res. 114 (1), E01010. doi:10.1029/2008JE003252
Melosh, H. J., and McKinnon, W. B. (1988). The tectonics of Mercury. Tucson, Arizona, United States: University of Arizona Press, 374–400.
Melosh, H. J. (1980). Tectonic patterns on a tidally distorted planet. Icarus 43 (3), 334–337. doi:10.1016/0019-1035(80)90178-5
Morriss, M. C., Karlstrom, L., Nasholds, M. W., and Wolff, J. A. (2020). The Chief Joseph dike swarm of the Columbia River flood basalts, and the legacy data set of William H. Taubeneck. Geosph. (Boulder). 16 (4), 1082–1106. doi:10.1130/GES02173.1
Muirhead, J. D., Airoldi, G., White, J. D., and Rowland, J. V. (2014). Cracking the lid: Sill-fed dikes are the likely feeders of flood basalt eruptions. Earth Planet. Sci. Lett. 406, 187–197. doi:10.1016/j.epsl.2014.08.036
Pappalardo, R. T., and Collins, G. C. (2005). Strained craters on ganymede. J. Struct. Geol. 27 (5), 827–838. doi:10.1016/j.jsg.2004.11.010
Polit, A. T., Schultz, R. A., and Soliva, R. (2009). Geometry, displacement–length scaling, and extensional strain of normal faults on Mars with inferences on mechanical stratigraphy of the Martian crust. J. Struct. Geol. 31 (7), 662–673. doi:10.1016/j.jsg.2009.03.016
Roggon, L., Hetzel, R., Hiesinger, H., Clark, J. D., Hampel, A., and van der Bogert, C. H. (2017). Length-displacement scaling of thrust faults on the Moon and the formation of uphill-facing scarps. Icarus 292, 111–124. doi:10.1016/j.icarus.2016.12.034
Sauro, F., Pozzobon, R., Santagata, T., Tomasi, I., Tonello, M., Martínez-Frías, J., et al. (2019). “Volcanic caves of lanzarote: A natural laboratory for understanding volcano-speleogenetic processes and planetary caves,” in Lanzarote and chinijo islands geopark: From Earth to space (Berlin, Germany: Springer), 125–142.
Schaaf, A., and Bond, C. E. (2019). Quantification of uncertainty in 3-D seismic interpretation: Implications for deterministic and stochastic geomodeling and machine learning. Solid earth. 10, 1049–1061. doi:10.5194/se-10-1049-2019
Schultz, R. A., Okubo, C. H., and Wilkins, S. J. (2006). Displacement-length scaling relations for faults on the terrestrial planets. J. Struct. Geol. 28 (12), 2182–2193. doi:10.1016/j.jsg.2006.03.034
Schultz, R. A., and Watters, T. R. (2001). Forward mechanical modeling of the Amenthes Rupes thrust fault on Mars. Geophys. Res. Lett. 28 (24), 4659–4662. doi:10.1029/2001GL013468
Solomon, S. C. (1978). On volcanism and thermal tectonics on one-plate planets. Geophys. Res. Lett. 5 (6), 461–464. doi:10.1029/GL005i006p00461
Stern, R., Gerya, T., and Tackley, P. (2018). Stagnant lid tectonics: Perspectives from silicate planets, dwarf planets, large moons, and large asteroids. Geosci. Front. 9, 103–119. doi:10.1016/j.gsf.2017.06.004
Stern, R. J. (2016). Is plate tectonics needed to evolve technological species on exoplanets? Geosci. Front. 7 (4), 573–580. doi:10.1016/j.gsf.2015.12.002
Strom, R. G. (1979). Mercury: A post-mariner 10 assessment. Space Sci. Rev. 24 (1), 3–70. doi:10.1007/bf00221842
Strom, R. G., Trask, N. J., and Guest, J. E. (1975). Tectonism and volcanism on Mercury. J. Geophys. Res. 80 (17), 2478–2507. doi:10.1029/JB080i017p02478
Thomas, W. A. (2006). Tectonic inheritance at a continental margin. GSA today 16 (2), 4–11. doi:10.1130/1052-5173(2006)016[4:tiaacm]2.0.co;2
Toda, S., Stein, R. S., Sevilgen, V., and Lin, J. (2011). Coulomb 3.3 graphic-rich deformation and stress-change software for earthquake, tectonic, and volcano research and teaching—user guide: U.S. Geological survey open-file report 2011–1060. Available at: https://pubs.usgs.gov/of/2011/1060/.
Torrese, P., Pozzobon, R., Rossi, A. P., Unnithan, V., Sauro, F., Borrmann, D., et al. (2021). Detection, imaging and analysis of lava tubes for planetary analogue studies using electric methods (ERT). Icarus 357, 114244. doi:10.1016/j.icarus.2020.114244
Van Der Bogert, C. H., Clark, J. D., Hiesinger, H., Banks, M. E., Watters, T. R., and Robinson, M. S. (2018). How old are lunar lobate scarps? 1. Seismic resetting of crater size-frequency distributions. Icarus 306, 225–242. doi:10.1016/j.icarus.2018.01.019
Watters, T. R. (2004). Elastic dislocation modeling of wrinkle ridges on Mars. Icarus 171 (2), 284–294. doi:10.1016/j.icarus.2004.05.024
Watters, T. R., Robinson, M. S., Collins, G. C., Banks, M. E., Daud, K., Williams, N. R., et al. (2015). Global thrust faulting on the Moon and the influence of tidal stresses. Geology 43 (10), 851–854. doi:10.1130/G37120.1
Watters, T. R., and Robinson, M. S. (1999). Lobate scarps and the Martian crustal dichotomy. J. Geophys. Res. 104 (8), 18981–18990. doi:10.1029/1998JE001007
Wise, D. U., Golombek, M. P., and McGill, G. E. (1979). Tectonic evolution of Mars. J. Geophys. Res. 84 (14), 7934–7939. doi:10.1029/JB084iB14p07934
Keywords: structural geology, tectonics, Mars, Mercury, Moon, planetary geology
Citation: Crane K and Galluzzi V (2022) Exciting opportunities in planetary structural geology and tectonics: An early career perspective. Front. Earth Sci. 10:1046652. doi: 10.3389/feart.2022.1046652
Received: 16 September 2022; Accepted: 18 November 2022;
Published: 28 November 2022.
Edited by:
Andrea Billi, National Research Council (CNR), ItalyReviewed by:
Ken McCaffrey, Durham University, United KingdomCopyright © 2022 Crane and Galluzzi. This is an open-access article distributed under the terms of the Creative Commons Attribution License (CC BY). The use, distribution or reproduction in other forums is permitted, provided the original author(s) and the copyright owner(s) are credited and that the original publication in this journal is cited, in accordance with accepted academic practice. No use, distribution or reproduction is permitted which does not comply with these terms.
*Correspondence: Kelsey Crane, a2Vsc2V5Y3JhbmVAZ2Vvc2NpLm1zc3RhdGUuZWR1
Disclaimer: All claims expressed in this article are solely those of the authors and do not necessarily represent those of their affiliated organizations, or those of the publisher, the editors and the reviewers. Any product that may be evaluated in this article or claim that may be made by its manufacturer is not guaranteed or endorsed by the publisher.
Research integrity at Frontiers
Learn more about the work of our research integrity team to safeguard the quality of each article we publish.