- 1Shaanxi Earthquake Agency, Xi’an, China
- 2United Laboratory of High-Pressure Physics and Earthquake Science, Institute of Earthquake Forecasting, CEA, Beijing, China
Significant anomalous hydrogeochemical changes in hot spring water are detected during strong seismic cycles. It is now necessary to clarify the relationship between tectonic movements, earthquakes and the evolution of hot springs. In this paper, laboratory analyses of major, trace elements, δD, δ18O and 87Sr/86Sr values of 28 hot spring waters in the Jinshajiang fault zone (JSJFZ) in the northwestern boundary of the Sichuan-Yunnan block were conducted. The results showed that the primary source of water for JSJFZ hot springs was atmospheric precipitation. The geothermal reservoir temperature variation based on the silicon enthalpy mixing model ranged from 73 to 272°C. And the circulation depth range was 1.2–5.4 km. The segmentation characteristics of the 87Sr/86Sr values were related to the influence of source rocks on groundwater cycle processes. A conceptual model of the hydrologic cycle of hot springs explained the spatial distribution of earthquakes associated with tectonic movements. The Batang segment had the strongest water-rock reaction, the highest reservoir temperature and the deepest circulation depth; meanwhile, it was also an earthquake prone area. The fluid circulation of the JSJFZ corresponds well with the seismicity, which indicates that the hydrological characteristics of the hot spring water in a fracture zone play a crucial role in receiving information on seismic activity.
1 Introduction
Underground water geochemistry has played an important role in inferring geotectonic activity. Hydrological effects occurring during strong earthquake cycles have been observed both recently and historically (Scholz et al., 1973; King, 1986; Linde et al., 1988; Thomas, 1988; Kitagawa et al., 1996; Wang et al., 2001; Jonsson et al., 2003; Yang et al., 2011; Li et al., 2014; Skelton, 2014; Yan et al., 2014; Yuce et al., 2014; Manga and Wang, 2015; Rosen et al., 2018; Martinelli and Tamburello, 2020; Nakagawa et al., 2020; Fu et al., 2021; Zhang et al., 2021; Zhou et al., 2021). Large, localized, and sustained fluid pressures could account for weakness of deep-seated fault (Fulton and Saffer, 2009). In particular, geothermal hot springs are important conduits for the discharge of deep source fluids to the surface. The hot springs in the deep-seated fault zone have deep circulation. Due to high temperature and strong water-rock interaction in deep-cycle process, they can dissolve different kinds of minerals, resulting in different geochemical characteristics (Bo et al., 2015; Guo et al., 2014; Tian et al., 2021). Such changes are always considered to be beneficial for fault tectonic and seismic studies.
The Jinshajiang fault zone (JSJFZ) is located in the southeast of the Tibetan Plateau. It has unique advantages in studying the relationship between earthquake and hydrochemistry of hot springs. Firstly, its major faults cut deeply into the crust and even through the asthenosphere (Wang et al., 2018). Secondly, it is an earthquake-prone area that has been hit by several Ms ≥ 6 earthquakes since records began. Among them, the largest earthquake was the Batang M7¼ earthquake in 1870 (Li et al., 2014). Since then, several earthquakes swarms with Ms ≥ 6 had occurred in this region. Recently, the small and intermediate seismic activities in Batang have been more active. More importantly, the Batang geothermal field is a significant part of the eastern Tibetan Plateau geothermal belt (Tang et al., 2017). It is the geothermal field with the highest reservoir temperature (200°C–225°C) along the Sanjiang Orogenic belt, and it has experienced a deep cycle (Yi et al., 2021).
Previous studies have focused on the source of heat and chemical characteristics of some springs in this area (Shi and wang, 2017; Tang et al., 2017; Zhang, 2017; Hou et al., 2018; Tian et al., 2018, 2019; Zhou et al., 2020a; Yi et al., 2021; Liu et al., 2022). However, limited work has been reported on the relationship between the hydro-chemical characteristics of hot springs and fault activity. Such studies are important for evaluating the geothermal energy potential along the fracture zone. In this study, we focus on the hydrochemical properties and origin of 28 hot springs located in the JSJFZ. Major and trace elements, 87Sr/86Sr values, δD and δ18O values are discussed to character the hydrochemical properties of these hot springs. Various methods were employed to calculate the reservoir temperature and depth of water circulation of these hot springs, so as to reveal their possible hydrochemical evolution processes. An attempt has been made to discuss the relationship between hot spring evolution and seismic activity through a conceptual model of hot spring hydrology cycle, and prospect its implications for future monitoring.
2 Geological setting
The JSJFZ is located in the southeastern Tibetan Plateau, which has long been controlled by the NS compression due to the collision between the Indian and Eurasian continents (Yin and Harrison, 2003). Since the late Cenozoic, because of the rapid northward growth and episodic eastward extrusion of the Tibetan Plateau, the Sichuan-Yunnan diamond-shaped block (SYDSB) has become the main channel for the escape of plateau materials to the east and southeast (Zhu et al., 2017). The JSJFZ is part of the northwestern margin of the SYDSB (Figure 1A). It is a multi-stage active suture, and the faults in this area are mainly right-lateral strike-slip and thrust faults (Wang et al., 2018). Its general trend is NW-SE oriented distribution, with a total length of 1,200 km and a width of 50–70 km (Xia and Zhu, 2020). In particular, its major faults cut deeply into the crust and even through the asthenosphere. Tectonically, the JSJFZ is cut into two segments by the NNE Batang fault (BTF) (Figure 1B), namely the Northern Jingshajiang fault (NJSJF) and the Southern Jinshajiang fault (SJSJF). The BTF is a dextral fault that strikes N30°E and dips to the northwest at a sharp angle, with a total length of about 200 km. High-temperature hot springs are distributed along the JSJF and BTF. Particularly, hot springs with temperature higher than 80°C have been found in the Batang geothermal field.
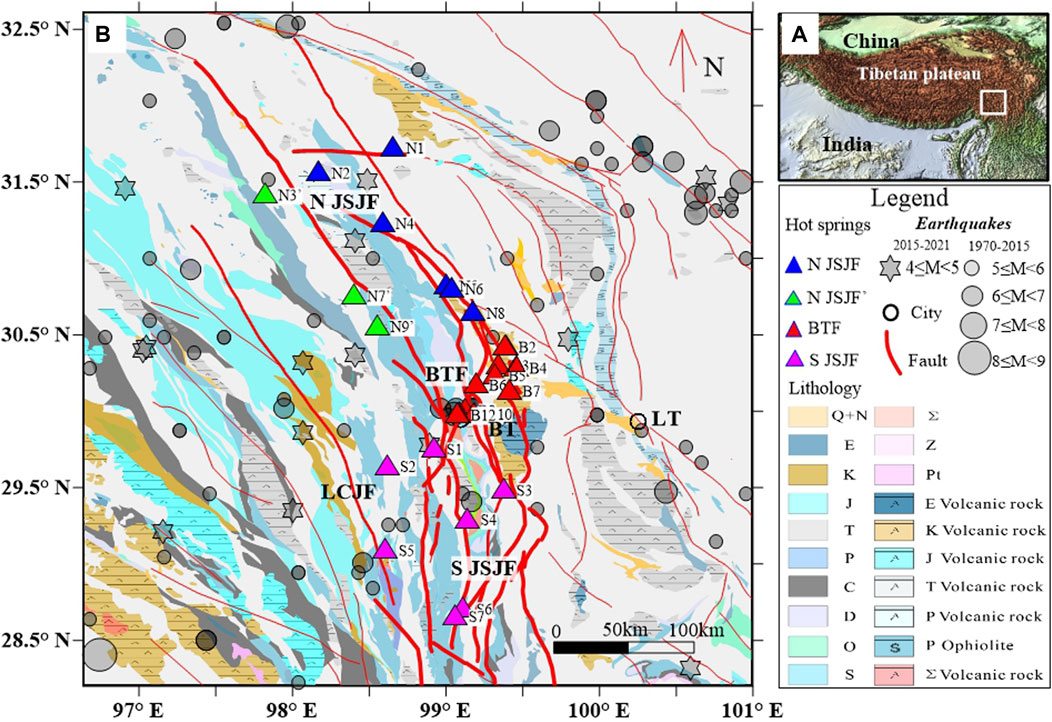
FIGURE 1. The plot of sampling site distribution. (A) Geological map showing the location of the study area. (B) Tectonic and tectonic and lithological features of the JSJFZ. Notes: The statistical earthquakes were divided into two parts, one is between 1970 and 2015 (grey circle), and the other is between 2015 and 2021 (grey polygon). The hot springs in the JSJFZ are divided into four parts (colored triangle). The background represents the lithology of the study area. The red line represents the fault.
The area is covered by widespread Triassic limestone with multi-stage magmatic activity. It owns a well-developed fracture system and frequent earthquakes. Since 780 B.C., two large earthquakes with magnitude >7.0 have occurred in this region, and minor to intermediate seismic events are frequent (Figure 1B). For the SJSJF, the dextral strike-slip rate is 4.9 mma−1 during the last 20 years, with a locking depth of approximately 20 km, while the slip rate of the NJSJF is not significant (Xu et al., 2020). For the BTF, the dextral strike-slip rate was 10.8 ± 2.3 mma−1 during 1999–2007 (Li et al., 2014), and its slip rate is consistent with that of the SJSJF in recent years (Xu et al., 2020).
3 Sampling and analysis
A total of 28 hot spring water samples were collected from the JSJFZ in 2018–2019. Based on the geological structure, the 28 hot springs were divided into three sections: N1-N9 in the NJSJF, B1-B12 in the BTF and S1-S7 in the SJSJF, respectively. Among them, hot springs N3, N7, and N9 were located in the secondary fault of NJSJF (Figure 1B). The main sampling information is listed in Table 1.
All water samples were collected in new, colorless, polyethylene terephthalate (PET) bottles that had been rinsed with the water samples. Specific conductivity, pH, dissolved oxygen and temperature of the spring water samples were measured in situ by placing multi-parameter probes into the spring vents. Reagent-quality HNO3 was added to each sample to lower down the pH below 1. The concentrations of cations and anions were measured by a Dionex ICS-900 ion chromatograph and an AS40 automatic sampler at the Earthquake Forecasting Key Lab of China Earthquake Administration (https://www.ief.ac.cn/sysbygypt/), with the reproducibility within ±2% and detection limits 0.01 mg/L. For SiO2 analysis, the geothermal water samples were diluted ten-fold using deionized water to prevent precipitation of SiO2 in water. Trace elements were analyzed at the Test Center of the Research Institute of Uranium Geology (http://www.albriug.cn/) by Element XR ICP-MS (Thermo Fisher, Bremen, Germany). The hydrogen and oxygen isotopes were measured using a Finnigan MAT253 mass spectrometer, via the TC/EA method. Results were expressed as parts per thousand deviations from the Vienna Standard Mean Ocean Water (V-SMOW). Precisions of ±0.2% (2S.D.) and ±1% (2S.D.) were obtained for δ18O and δD in a standard water sample, respectively (Wang et al., 2010).
4 Results and discussion
4.1 General hydrochemistry and origin of major ions
The 28 geothermal water samples in the study area were mainly divided into three categories, N1–N9, B1–B12, and S1–S7, respectively (Table 2). The Piper diagram in Figure 2 shows the distribution of the main cations (Ca2+, Mg2+, Na+, K+) and anions (HCO3−, Cl− and SO42−) of all water samples, which can be used to classify the hydrochemical characteristics of the water samples. It shows that Ca2+, Na+, and HCO3− are the main chemical components for most of the samples.
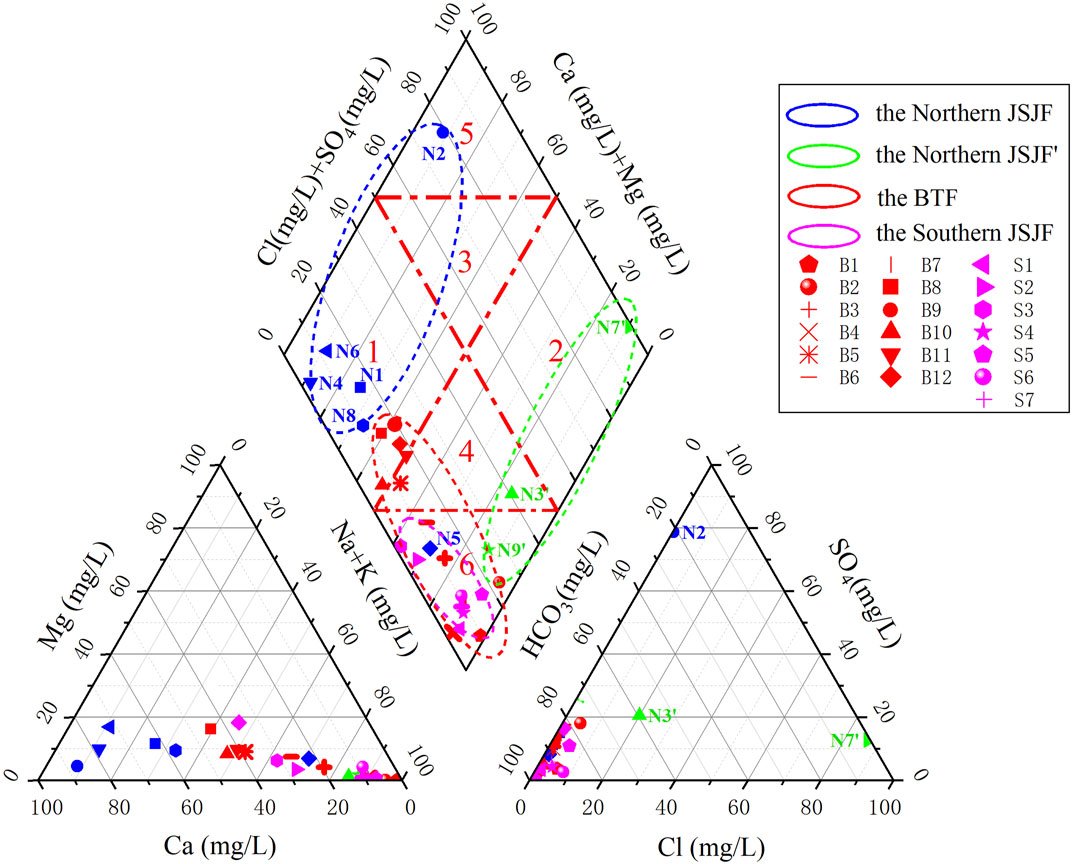
FIGURE 2. Piper diagram showing major ion chemistry of the sampled points. Notes: The hot springs in the blue and green sashed circular area are located in the Northern JSJF, and they are divided into two parts. The hot springs in the red sashed circular area are located in the BTF. The hot springs in the purple sashed circular area are located in the Southern JSJF.
4.1.1 Hot springs from the NJSJF (N1-N9)
N1 - N9 are located in the major and secondary faults of the NJSJF. All these samples can be divided into two groups, N1, N2, N4, N5, N6, N8 in the major fault and N3′, N7′, N9′ in the secondary fault of the NJSJF. Except for N2 (Ca-SO4•HCO3) and N7’ (Na-Cl), the hydrochemical types of these hot spring water samples are Na-HCO3 and Ca•Na-HCO3 in the major and secondary faults of NJSJF. The temperatures of these hot springs are in the range of 10.57°C to 73.8°C, and the pH values vary between 6.57 and 8.25. Most of the spring water samples have low total dissolved solids (TDS) values ranging from 254.83 mg/L to 1757.77 mg/L, except for N7’, which has a TDS value of 43,542.98 mg/L.
In the major fault of the NJSJF, as shown in Figure 2 (blue dots), most of hot spring water samples are distributed in “Zone 1”, where the hardness of carbonic acid exceeds 50% and the chemical properties of groundwater are mainly alkaline earth metals and weak acids. Their aquifers are located in the Triassic limestone. As for sample N5, it locates in “Zone 6”, where the carbonic alkali metal proportion is more than 50%. Na+ in the spring water is mainly from the alternating dissolution and cation adsorption of albite, potassium feldspar, anorthite and other minerals. HCO3− mainly come from the dissolution of carbonate rocks such as limestone and dolomite (Yi et al., 2021). As for sample N2, it locates in “Zone 5”, where non-carbonic acid hardness exceeds 50%. Generally, as the depth of groundwater infiltration depth increases, the leaching effect is enhanced and the concentration of SO42- in the spring water increases. Sample N2 is located in Triassic sandstone, mudstone, volcanic strata and limestone. Oxidation of pyrite in aqueous medium can accelerate the dissolution of limestone, so as to enrich the SO42- in spring water.
In the secondary fault of the NJSJF, as shown in Figure 2 (green dots), the concentrations of Cl− (25,238.17 mg/L), SO42- (3708.77 mg/L), Na+ (12,979.57 mg/L) and Ca2+ (1258.70 mg/L) in N7′ are much higher than those in N3′, N9’. The TDS value of N7’ (43,542.98 mg/L) is the highest one among the samples in the NJSJF. And N7′ is located near the top of the right side of “Zone 2”, where non-carbonated alkali metal content is more than 50% and the groundwater chemistry is mainly alkali metal and strong acid. It may have originated from ancient seawater. During the water cycle, it was concentrated and finally became a deep brine with high salinity (Yan et al., 2021). Meanwhile, N9′ is located in “Zone 6”, where the proportion of carbonic alkali metals exceeds 50%. Their origin is similar to that of N5.
4.1.2 Hot springs from the BTF (B1-B12)
B1–B12 are located in the BTF (Figure 2, red dots). The hydro-chemical types of these hot spring water samples are mainly Na-HCO3, Ca•Na-HCO3 and Na•Ca-HCO3. The temperature of these hot springs varies between 31.50°C and 86.1°C, and the pH value varies between 7.12 and 8.89. The TDS values of these spring water samples are relatively low, ranging from 378.96 mg/L ∼ 1254.35 mg/L. Compared to the samples collected in the NSJSF, the hydro-chemical types of hot spring water samples in the BTF do not change significantly, i.e., Na-HCO3, Ca•Na-HCO3 and Na•Ca-HCO3. Only the hydro-chemical type of B9 is Ca•Mg-HCO3. The Na-HCO3 type waters are located in “Zone 6”, while other samples are mainly located in the transition region of “Zone 1” and “Zone 4”. It indicates that their source rocks are mainly limestone, dolomite, calcareous sandstone and siltstone.
4.1.3 Hot springs from the SJSJF (S1-S7)
S1–S7 are located in the SJSJF (Figure 2, purple dots). The hydro-chemical types of these hot spring water samples are mainly Na-HCO3 and Ca•Na-HCO3. The temperature of these hot springs varies between 17.60°C and 73.50°C, and the pH value varies between 6.73 and 8.21. The TDS values of these spring water samples are relatively low, ranging from 315.32 mg/L∼1313.54 mg/L. Minimal change of the hydro-chemical types of hot spring water samples is found in the SJSJF. All these spring waters are located in “Zone 6”, which is the same with some of the samples in the BTF. It indicates that their source rocks are much more similar, mainly limestone, dolomite.
To sum up, all these spring water samples in the JSJFZ have the following characteristics. Congruent and incongruent dissolution of aquifer rocks, hydrothermal conditions, hydrodynamic power, together with cation exchange reactions may strongly influence the ionic concentration and facies types of the groundwater in the aquifer along the JSJFZ. Carbonate rocks are mainly developed in the study area, and HCO3− in the hot springs mainly comes from carbonate rocks, while Ca2+ and Mg2+ mainly come from soluble limestone (CaCO3) and dolomite (MgCO3) dissolved in the groundwater. In addition to dolomites, limestone, and carbonate rocks, sandstones and conglomerates are also developed in the study area, mainly including quartz, mica, feldspar and other aluminosilicate minerals, etc. Under high temperature and pressure, the recycled water reacts with aluminosilicate and carbonate rocks, causing a large amount of Na+, silicic acid, and carbonate in the surrounding rocks to dissolve in water (Zhang et al., 2003).
4.2 Origin of trace elements hot spring waters
Water-rock reactions during the deep groundwater circulation are responsible for the variation in trace element content. Therefore, the degree of water-rock reaction can be inferred to some extent by analyzing the trace element content characteristics. In this study, the trace elements measurements of 26 samples in the JSJFZ are shown in Supplementary Table S1. Twenty-four kinds of trace elements were determined, including Ti, V, Cr, Fe, Co, Ni, Cu, Zn, Ag, Cd, Sn, Sb, Pb, Li, Be, B, Al, Sr, Mo, Ba, Tl, Th, U, and Mn. The enrichment factor (EFi) is the degree of enrichment of an element in a given geological body, from which the source of trace elements in a hot spring can be determined qualitatively (Ji et al., 2017). The EFi is calculated as follows:
Where CR is the selected reference elemental content, Ci is the elemental content in the sample, w and r is the elemental content in the water sample and the rock, respectively. Titanium (Ti) was chosen as the reference element, and the average content of trace elements in typical granites was used as the reference values from Ji et al. (2017).
As shown in Figure 3, the EFi of Li, B and Sr are relatively higher than the other elements. The mobile chalcophile elements may originate from external sources, such as sulfide-rich altered rocks. The content of alkali metal elements such as Li is generally lower than the major elements, but due to their active chemical properties, strong mobility, they are mainly enriched in acidic rocks with strong migration ability, and the content of some springs is close to or even exceeds the abundance of the surrounding rocks. The activity of Li may be a signature of deep fluid upwelling during deep fracture activity. Li-silicate minerals, such as lithium mica and chert, are usually formed in volcanic and magmatic rocks, and Li can enter water in a dissolved state under hydrolysis (Zhang et al., 2003). However, the Li concentration in the hot springs of the JSJFZ is not as high as that in the Kangding area, indicating a lack of magmatic source in the JSJFZ (Yi et al., 2021).
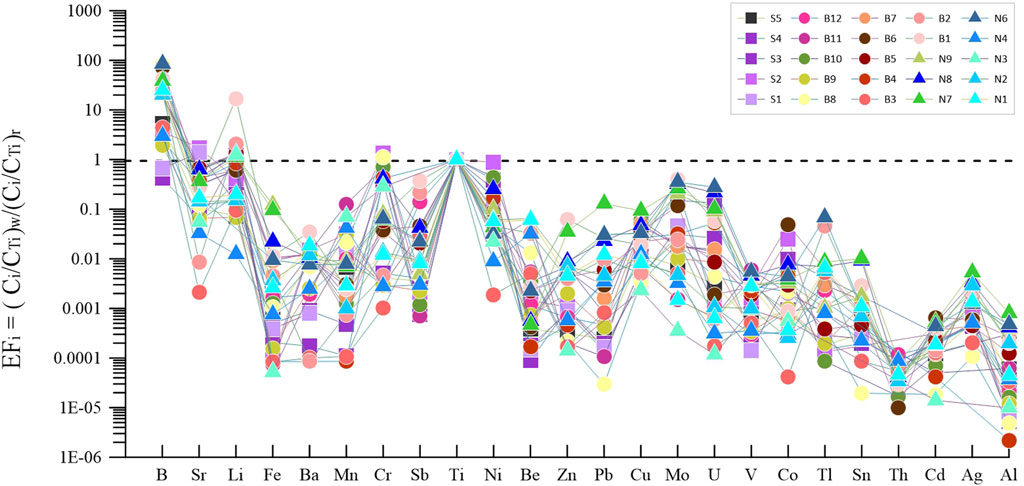
FIGURE 3. Trace element distribution in terms of enrichment coefficient (weight ratios) normalized to Ti for waters of the JSJFZ. Notes: The EFi means the degree of enrichment of an element in a given geological body, from which the source of trace elements in a hot spring can be determined qualitatively, and the EFi of Li, B, and Sr are relatively higher than the other elements.
Studies have shown that the deeper the groundwater cycle, the higher the B content, because the solubility of B in groundwater increases with depth and pressure (Cai et al., 2001; Zhang et al., 2003). Hot springs with high B content are mainly located in the middle segment of the JSJFZ, i.e. the BTF, indicating that the circulation depth of hot springs in the BTF is larger.
Alkaline-earth metal Sr is a disperse element with high abundance in the crust, mantle and weak alkaline water with a pH of 7.0–8.5 (Cai et al., 2001). In addition, Sr is usually associated with calcium and potassium and is therefore present in calcium and potassium-rich minerals such as potassium feldspar and hornblende (Zhang et al., 2003). Volcanic and granitic rocks rich in potassium feldspar and hornblende are developed in this study area. The pH value of spring water samples in the JSJFZ ranged from 6.57 to 8.89 with a mean value of 7.53, which was favorable for Sr enrichment. Groundwater is heated and reacted at fracture depths, and is recycled into hot spring water after a series of reactions.
The cluster analysis of the major ions in the water samples from the JSJFZ shows that sample B2 in the BTF is different from the other samples (Figure 4) when the center distance is set as 10. Combined with the δD and δ18O values of sample B2 (Table 3; Figure 5), it indicates that the water-rock reaction here is stronger than elsewhere.
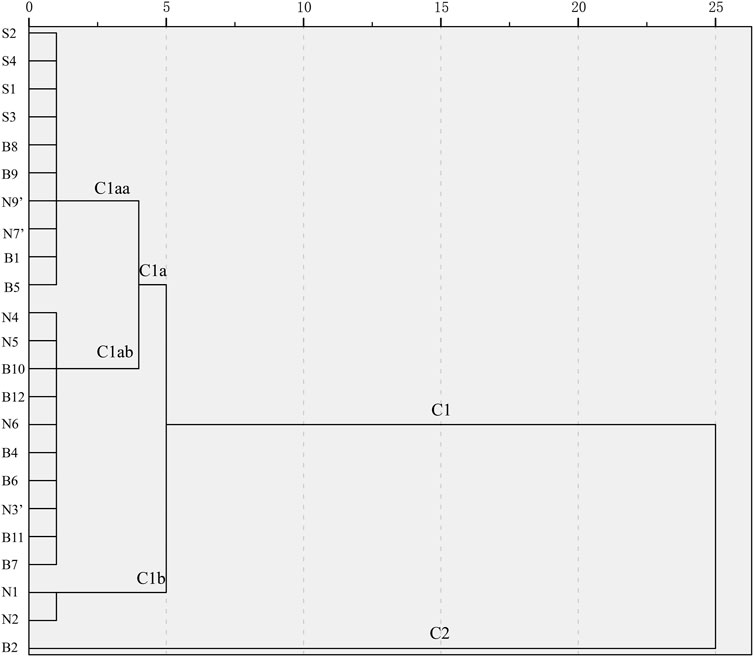
FIGURE 4. Cluster analysis of major ions of the spring water samples. Notes: All the samples can be divided into two parts, namely C1 and C2. And for C1, it can be divided into C1a and C1b. And furthermore, the C1a can be divided into C1aa and C1ab.
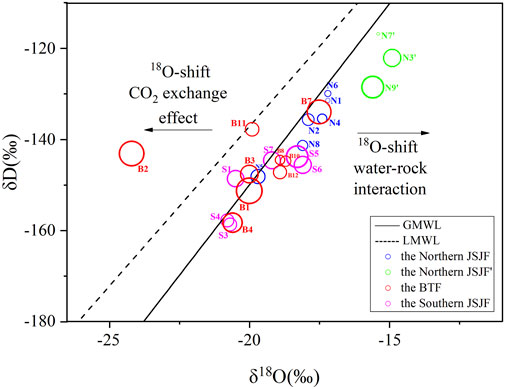
FIGURE 5. Stable hydrogen and oxygen isotope composition of samples in the JSJFZ. Notes: The circles with different colors represents hot springs in different faults. The size of the circles means the different reservoir temperatures, and the larger the size of the circle, the higher the reservoir temperature.
4.3 Origin of hot spring waters
Stable oxygen and hydrogen isotopes have been widely used to determine the source, transit time of geothermal fluids (Tian et al., 2018). Therefore, the relationship between δDand δ18O of water samples was plotted to trace the source of replenishment of geothermal fluids. In the study area, the δD and δ18O values of geothermal water samples (Table 3) ranged from −158.7‰ to −116.9‰ and −24.2‰ to −14.9‰ (VSMOW). As shown in Figure 5, the majority of the geothermal waters is plotted close to the Global Meteoric Water Line (Craig, 1961) and the western Sichuan Meteoric Water Line (Chen et al., 2014), indicating the recharge source of meteoric water. An exception is the spring water sample (B2) in the BTF, which may be attributable to the isotopic exchange reaction between water and CO2 isotopes (Pang et al., 2017). Groundwater is induced by precipitation recharging from high altitude regions. Aquifer rocks dissolved in the infiltrating water and freshening started, consisting of cation exchange during groundwater flow. δD and δ18O values are more negative for springs at high mountains or high latitudes, and less negative for springs at low altitudes and low latitudes.
Sr concentration and 87/86Sr values varied from 0.03 to 13.51 μg/L and 0.7078 to 0.7234, respectively. The spring waters in the NJSJF had lower Sr concentrations than those in the NJSJF and BTF. The 87Sr/86Sr values of the spring waters in the NJSJF were closer to the average 87Sr/86Sr value of carbonate rock (Figure 6), while the 87Sr/86Sr values of the spring waters in the BTF and the SJSJF belonged to the aluminosilicate weathering. The segmental characteristics of the different strontium isotopes suggest that the hot springs were formed by the interaction with Sr-bearing source rocks in the crust during the deep circulation of atmospheric precipitation in the local heat flow system. This is consistent with the geochemical characteristics of the hot spring waters and the lithology of the surrounding rocks.
4.4 Water-rock interaction of hot springs in the JSJFZ
4.4.1 The water-rock reaction equilibrium
The Na-K-Mg ternary diagram (Figure 7) can indicate the degree of ionic equilibrium reaction of the water sample. As shown in Figure 7, the blue and green circles represent water samples in the NJSJF. Except for sample N7′, the other samples are distributed in the “immature waters zone”. The red circles represent the water samples in the BTF, which are distributed in the “immature waters zone” or the “partially equilibrated and mixed waters zone.” And the purple circles represent water samples in the SJSJF that are distributed in the “immature waters zone” or the “partially equilibrated and mixed waters zone”. It can be preliminarily estimated that the spring waters in the BTF have the highest reservoir temperatures in the JSJFZ.
4.4.2 Mineral saturation states
Mineral equilibrium calculations help predict the presence of reactive minerals and estimate mineral reactivity in groundwater systems. By using the saturation index (SI) approach, reactive minerals in host rocks and minerals that may precipitate during the extraction and use of thermal fluids can be predicted from groundwater data without the need to examine solid phases samples (Deutsch, 1997). The mineral saturation indices of hydrothermal minerals that may be present in the reservoirs of geothermal systems were calculated by PHREEQCI-2.12 (USGS) at their outlet temperatures and pH values. Results are presented in Figure 8. Almost all groundwater samples were supersaturated (SI > 0) relative to calcite at the sampling temperature, suggesting that CO2 degassing may have occurred (Figure 8). Only the value of sample B7 was negative (−0.39), but it was also almost in equilibrium with calcite. This super-saturation state demonstrates the presence of substantial amounts of these minerals and sufficient residence time in the aquifer system (Rouabhia et al., 2012). Except for B4 and B7, groundwater samples were in equilibrium with dolomite (SI∼0). The possible cause of B4 and B7’s characteristics is that high CO2 concentrations lead to lower pH values and lower dolomite SI values (Zhou et al., 2020a). Almost all groundwater samples are in under saturation with halite (SI <−4). The rest of minerals in Supplementary Table S2 have various saturation indices.
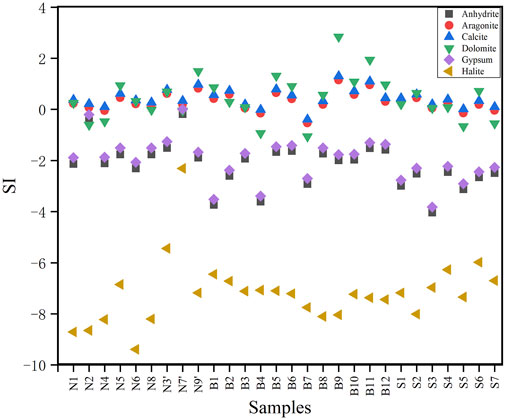
FIGURE 8. Saturation indices values of water samples with respect to minerals. Notes: The value of SI is divided by zero.
4.4.3 Reservoir temperature and circulation depth
Chemical geothermometers (including quartz and chalcedony) and cationic geothermometers (including Na-K and Na-Li system) are commonly used to estimate reservoir temperatures. The equations are as follows:
These geothermometers consist of equations or models based on temperature-dependent chemical reactions, from which equilibrium temperatures can be calculated. In fact, due to the complex geological settings, different chemical geothermometers always yield very different reservoir temperatures. In this study, we compared these calculation results (Table 4). The Na-K-Mg triangle diagram method could help to judge the equibibrium state of the geothermal water (Giggenbach, 1988). In Figure 7, the spring water samples are almost belong to immature waters, indicating that they are not fully equilibrated with the reservoir rocks, so the cation ratio geothermometers cannot provide reliable results than silica geothermeter with no-steam loss. Finally, we chose the quartz geothermometers with no steam loss (Fournier, 1981) as the optimum one. The circulation depth of the spring waters and the proportion of cold water were also deduced using the reservoir temperatures calculated by the Silicon-enthalpy mixing model (Fournier and Truesdell, 1974). The results showed that the TSiO2 of the NJSJF, BTF and SJSJF ranged from 36 to 133°C, 93 to 215°C and 100 to 134°C, respectively; the TS-E of the NJSJF, BTF and SJSJF ranged from 73 to 125°C, 115 to 272°C and 135 to 158°C, respectively. The results are close to those of Tian et al. (2019) on the BTF hot springs. The spatial distribution of outlet temperature and thermal reservoir temperature was consistent with each other. The spring water temperaure in the southern segment of the JSJFZ was higher than that of the northern segment, with the peak located in the BTF.
The circulation depths were evaluated according to the following equation:
D is the circulation depth; T is the reservoir temperature (◦C); T0 is the temperature of the local average temperature (◦C); Δt is the geothermal gradient (◦C/km) and h is the depth of constant temperature zone (km). For the Sichuan province, Δt, T0, h was assumed as 47.5°C/km, 16.42°C and 0.02km, respectively (Zhang et al., 2019).
The DSiO2 of the NJSJF, BTF and SJSJF was 0.4∼2.5 km, 1.6∼4.2 km and 1.8∼2.3 km, respectively. And the DS-E of the NJSJF, BTF and SJSJF was 1.2∼2.3 km, 2.1∼5.4 km and 2.5∼3.0 km. Similarly, the circulation depth of the spring waters in the BTF was the deepest.
4.5 Correlation between hydro-geochemical changes and seismic activities
4.5.1 Segmental hydro-chemical characteristics and spatial distribution of earthquakes
Generally, the ranking of the JSJFZ spring waters in terms of temperature, thermal reservoir temperature, circulation depth, water maturity, water-rock interaction intensity is roughly NJSJF < SJSJF < BTF. For historical earthquakes (M ≥ 5) in the JSJFZ (Figure 1), they used to appear in the NJSJF and BTF, and for recent earthquakes (M ≥ 1) in the JSJFZ, they gathered in the BTF (Figure 9A). It may be due to two mechanisms. The spring waters in the BTF contain more mantle derived materials (Zhou et al., 2017; Zhou et al., 2020a), which confirms that the BTF is characterized by a deep and large fault cutting through the crust or an ultra-crustal fault. Given its strong fault activity, the BTF became an earthquake-prone area. As shown in Figures 9B–E, the outlet temperature, circulation depth and mantle source helium in the hot springs of the BTF were the highest in the JSJFZ. Deep fluid circulation and facture coupling are important triggers of earthquakes. It can be inferred that the first mechanism is more consistent with the characteristics of the JSJFZ. In addition, the effects between fluids and earthquakes are mutual. On the one hand, deep-source fluids promote the occurrence of earthquakes, and on the other hand, the occurrence of earthquakes changes the crustal structure and fluid permeability (Manga and Wang, 2015).
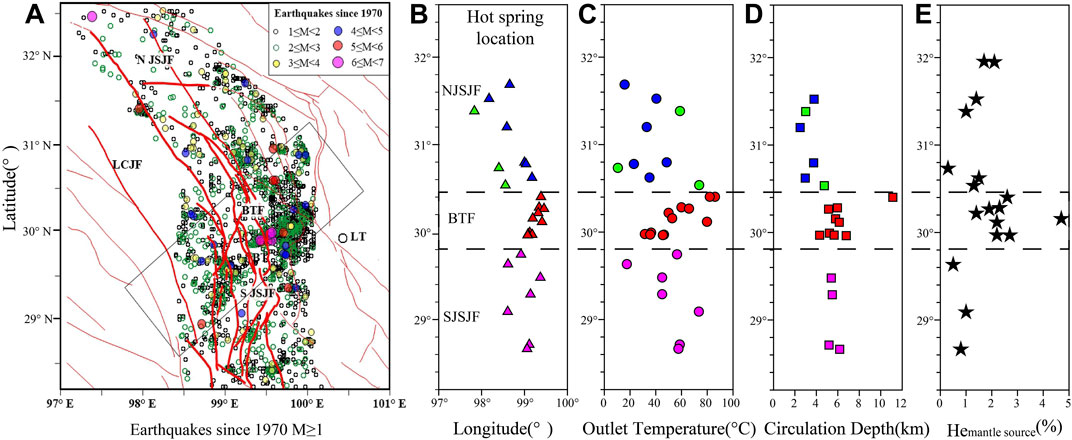
FIGURE 9. Earthquake (M ≥ 1) distribution in the JSJFZ since 1970 (A), hot spring location (B), outlet temperature (C), circulation depth (D) and mantle source helium (E), data from Zhou et al., 2020a).
Strong earthquakes may occur in the transition zone of geothermal anomalies (Liu et al., 2022). According to spatial distribution of the reservoir temperatures in the JSJFZ, the BTF coincides with the high and low temperature transition zone where the north-south segment intersects. Earthquakes are more expected to occur in the BTF rather than other areas due to its strong fluid activity characteristics and deep cutting depth. In fact, the BTF has been active since the late Quaternary. In 1870, the Batang M seven earthquake occurred here. According to the GPS measurements, the BTF has a dextral strike slip rate of 8.7 ± 2.1 mm/a (Wang et al., 2008). In the NJSJF, the fault slip is not significant, while in the BTF and the SJSJF, the slip rate reached ∼4.9 mm/a (Xu et al., 2020). Correspondingly, the hot springs in the BTF were the most active, and a large number of earthquakes also gathered here. In the BTF and SJSJF area, the locking depth is about 20 km (Xu et al., 2020) and there is a certain degree of strain accumulation in the faults. Earthquakes usually occur on faults with accumulated stresses, especially in areas with strong and weak stress transitions. Based on fracture activity, seismic distribution and geochemical characteristics of the hot springs, the BTF is currently at higher seismic risk than other areas in the JSJFZ.
4.5.2 Conceptual model and evolution of geothermal fluid in JSJFZ
Deep fracture zones can serve not only as a channel for further infiltration of groundwater, but also as a channel for rapid ascent of deep-derived geothermal fluid. It is noted that deep source fluids can trigger earthquakes (Fairley et al., 2003; Shi and wang., 2017; Hou et al., 2018; Wang et al., 2021). When a large amount of fluid invades into a fault, it may change the pore pressure and the stress state in the fault zone. Therefore, it may increase the frequency of minor and intermediate earthquakes. Besides, heat sources, permeability pathways and fault activity also play important roles in formation of hot springs (Chen et al., 2014; Zhou et al., 2020b). Tracing the sources and migration pathways of groundwater in active fault zones is of paramount importance in terms of studying hydro-geochemical precursors in seismic hazards zones.
A conceptual model for the origin of groundwater and the hydro-geochemical cycling process in the JSJFZ is summarized in Figure 10 according to the results of this study. Meteoric waters permeates into the aquifers along the fractures between and around mountains and river terraces through the water-conducting fault zone. Due to the difference in circulation depth, reservoir temperature and degree of water-rock reaction, partially equilibrated water or immature water were formed in the NJSJF, the BTF and the SJSJF. The spring water may mix with cold surface water or shallow groundwater with different mixing ratios during fluid ascent in the fault channel. Finally, it became exposed to the earth’s surface as a hot spring. When the crustal stress in the JSJFZ changes, the pressure in the aquifer system and the equilibrated state of hot spring water will be disrupted, resulting in the different hydro-chemical characteristics. The infiltrated waters in the BTF had a considerable deep circulation (∼5.4 km) in a high heat flow reservoir. This is the reason for the high mantle helium contribution (Tian et al., 2018; Zhou et al., 2020a), and the frequent high-intensity earthquakes here. Deeply-sourced volatile emissions may have responded rapidly to the onset of sustained plateau growth (Zhang et al., 2021).
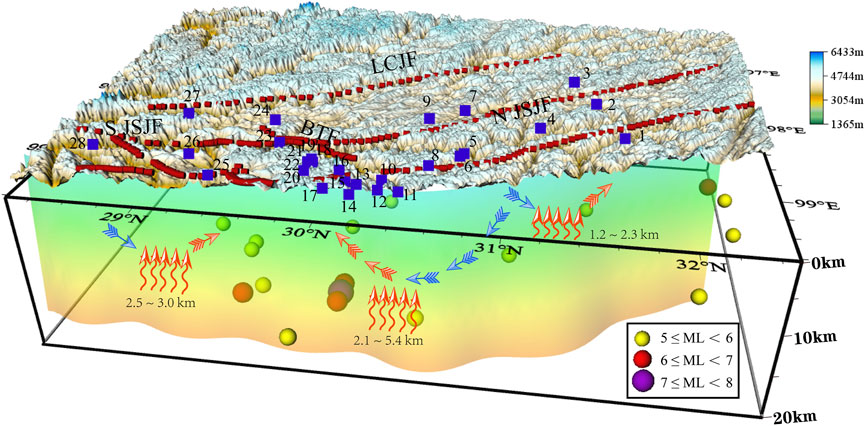
FIGURE 10. Conceptual model of the origin of groundwater and the hydrogeochemical cycling process in the JSJFZ. Notes: The red dashed line represents faults in the study area. The blue square represents the hot springs. The red and blue arrows represent runoff of hot and cold waters, respectively. The red curved arrow represents the suspected heat source location.
The Rayleigh-wave velocity of the NJSJF is higher than that of the southern SYDSB (Fan et al., 2015). The SJSJF is located in the low-velocity channel bounded by major strike-slip faults around the EHS and in the southeastern margin of Tibet (Bao et al., 2015; Hu et al., 2018). The high-resolution Qlg model showed that the main flow channel emerged from the northern end of plateau, extended east and southeast, and then turned south after being blocked by the rigid Sichuan Basin (Zhao et al., 2013). Consistently, the conductance values present very high conductivity regions near the SJSJF. As the fluid content increases, the crust weakens and flows (Unsworth et al., 2005). And crustal flows can occur in orogenic belts and contribute to the uplift of plateau (Bai et al., 2011). High conductivity and low velocity layer is a relatively soft medium, which is prone to deformation, stress and strain conduction, concentration and accumulation. The BTF is located in the transition area of the NJSJF and SJSJF (Wang and Shen, 2020) that is prone to earthquakes. The geophysical and fluid geochemical signatures here are well confirmed by each other. In summary, the hydrological characteristics of hot spring water in fault zones play a crucial role in receiving information on tectonic movements and seismic activities in advance.
5 Conclusion
Fault structures and tectonic movements jointly control the formation and geochemical characteristics of hot springs. The high-temperature geothermal system along the lithospheric-scale strike-slip JSJFZ was studied.
The geochemical characteristics of the 28 hot springs suggested that they were mainly recharged by atmospheric precipitation from the nearby mountains. Chemical geo-thermometry applications, together with silicon enthalpy mixing model calculation, presented a reservoir temperature range of 73 ∼ 272°C. The proportion of cold water ranged from 34% to 86%. And the circulation depths varied from 1.2 km to 5.4 km.
A conceptual model for the hot spring water origin and circulation cycle showed that the meteoric water firstly seeped into the fault and was heated by the wall rocks. Then, it circulated along faults and fissures to the surface, where it eventually formed hot springs.
The segmental characteristics of the fault, the hydration characteristics together with the H, O, and Sr isotopes of the hot springs located in different segments of the JSJFZ and the seismic activities are closely related to each other. Notably, the hot springs in the BTF had deeper recharges source and high reservoir temperatures than those in the NJSJF and the SJSJF. And it was coincident with strong tectonic and seismological activity here in the BTF. Thus, the spatial distribution hot springs, the hydro-geochemical characteristics and the influence of controlling factors are of great importance to further exploration of strong seismic information.
Data availability statement
The original contributions presented in the study are included in the article/Supplementary Material, further inquiries can be directed to the corresponding authors.
Author contributions
JL as the first author of this manuscript, wrote the main part of the article. XZ and YL as the corresponding authors, controlled the article ideas. MH, JL, JD, and FL helped collect the water samples. JT, YY, and SO made their contributions in the data analysis.
Funding
The work was funded by the Natural Science Foundation of Shaanxi Province, China (2022JQ-254), Spark Program of Earthquake Sciences ( XH21032), National Key Research and Development Project (2017YFC1500501-05 and 2019YFC1509203), the National Natural ScienceFoundation of China (41673106, 42073063, and 4193000170), the Special Fund of the Institute of Earthquake Forecasting, China Earthquake Administration (2021IEF0101, 2021IEF0201, and 2021IEF1201) and Open Fund for Earthquake Forecasting (2021EFOF03).
Acknowledgments
We thank the Editor and two reviewers for their constructive comments and suggestions.
Conflict of interest
The authors declare that the research was conducted in the absence of any commercial or financial relationships that could be construed as a potential conflict of interest.
Publisher’s note
All claims expressed in this article are solely those of the authors and do not necessarily represent those of their affiliated organizations, or those of the publisher, the editors and the reviewers. Any product that may be evaluated in this article, or claim that may be made by its manufacturer, is not guaranteed or endorsed by the publisher.
Supplementary Material
The Supplementary Material for this article can be found online at: https://www.frontiersin.org/articles/10.3389/feart.2022.1015134/full#supplementary-material
References
Bai, D. H., Unsworth, M. J., Meju, M. A., Ma, X., Teng, J., Kong, X., et al. (2011). Crustal deformation of the eastern Tibetan plateau revealed by magnetotelluric imaging. Nat. Geosci. 497, 358–362. doi:10.1038/ngeo830
Bao, X., Sun, X., Xu, M., Eaton, D. W., Song, X., Wang, L., et al. (2015). Two crustal low-velocity channels beneath SE Tibet revealed by joint inversion of Rayleigh wave dispersion and receiver functions. Earth Planet. Sci. Lett. 415, 16–24. doi:10.1016/j.epsl.2015.01.020
Bo, Y., Liu, C., Zhao, Y., and Wang, L. (2015). Chemical and isotopic characteristics and origin of spring waters in the lanping–simao basin, yunnan, southwestern China. Geochemistry 75 (3), 287–300. doi:10.1016/j.chemer.2015.04.002
Chen, Z., Du, J. G., Zhou, X. C., Yi, L., Liu, L., Xie, C., et al. (2014). Hydrochemistry of the hot springs in western sichuan province related to the WenchuanMS8.0 earthquake. Sci. World J. 2014, 1–13. doi:10.1155/2014/901432
Craig, H. (1961). Isotopic variations in meteoric waters. Science 133 (3465), 1702–1703. doi:10.1126/science.133.3465.1702
Deutsch, W. J. (1997). Groundwater geochemistry: Fundamentals and applications to contamination. New York, NY, USA: Lewis Publisher.
Fairley, J., Heffner, J., and Hinds, J. (2003). Geostatistical evaluation of permeability in an active fault zone. Geophys. Res. Lett. 30 (18), 1962. doi:10.1029/2003gl018064
Fan, L. P., Wu, J. P., and Fang, L. H. (2015). The characteristics of Rayleigh group velocities in the southeastern margin of the Tibetan Plateau and its tectonic implications. Chin. J. Geophys. 58 (5), 1555–1567. in Chinese. doi:10.6038/cjg20150509
Fournier, R. O. (1981). “Application of water geochemistry to geothermal exploration and reservoir engineering,” in Geothermal systems, principles and case histories. Editors L. Rybach, and L. J. P. Muffler (New York: Wiley), 109–143.
Fournier, R. O., and Truesdell, A. H. (1974). Geochemical indicators of subsurface temperature PartII, estimate of temperature and fractions of hot water mixed with cold water. J. Res. U.S. Geol. Surv. 2 (3), 263–270. doi:10.3133/ofr741032
Fu, C. C., Lai, C. W., Yang, T. F., Hilton, D. R., Chen, C. H., Walia, V., et al. (2021). An automatic system for continuous monitoring and sampling of groundwater geochemistry in earthquake-prone regions of SW Taiwan. Front. Earth Sci. (Lausanne). 9, 635913. doi:10.3389/feart.2021.635913
Fulton, P. M., and Saffer, D. M. (2009). Potential role of mantle-derived fluids in weakening the San Andreas Fault. J. Geophys. Res. 114, B07408. doi:10.1029/2008JB006087
Giggenbach, W. F. (1988). Geothermal solute equilibria. derivation of Na-K-Mg-Ca geoindicators. Geochim. Cosmochim. Acta 52, 2749–2765. doi:10.1016/0016-7037(88)90143-3
Hou, Y. Y., Shi, Z. M., and Mu, W. Q. (2018). Fluid geochemistry of fault zone hydrothermal system in the yidun-litang area, eastern Tibetan Plateau geothermal belt. Geofluids 2018, 1–13. doi:10.1155/2018/6872563
Hu, J. F., Badal, J., Yang, H. Y., Li, G., and Peng, H. (2018). Comprehensive crustal structure and seismological evidence for lower crustal flow in the southeastern margin of Tibet revealed by receiver functions. Gondwana Res. 55, 42–59. doi:10.1016/j.gr.2017.11.007
Ji, L., Liu, F. L., and Wang, F. (2017). Multiple granitic magma events in north middle segment of Diancangshan Ailaoshan complex zone: Impications for tectonic evolution. Acta Pet. Sin. 33, 2957–2974. in Chinese.
Jonsson, S., Segall, P., Pedersen, R., and Bjornsson, G. (2003). Post-earthquake ground movements correlated to pore-pressure transients. Nature 424, 179–183. doi:10.1038/nature01776
King, C. Y. (1986). Gas geochemistry applied to earthquake prediction: An overview. J. Geophys. Res. 91 (B12), 12269–12281. doi:10.1029/jb091ib12p12269
Kitagawa, Y., Koizumi, N., and Tsukuda, T. (1996). Comparison of postseismic groundwater temperature changes with earthquake-induced volumetric strain release: Yudani Hot Spring, Japan. Geophys. Res. Lett. 23 (22), 3147–3150. doi:10.1029/96GL02517
Li, Y. H., Hao, M., Ji, L. Y., and Qin, S. L. (2014). Fault slip rate and seismic moment deficit on major active faults in mid and south part of the Eastern margin of Tibet plateau. Chin. J. Geophys. 57 (4), 1062–1078. in Chinese. doi:10.6038/cjg20140405
Linde, A. T., Suyehiro, K., Miura, S., Sacks, I. S., and Takagi, A. (1988). Episodic aseismic earthquake precursors. Nature 334, 513–515. doi:10.1038/334513a0
Liu, W., Guan, L. F., Liu, Y., Xie, X., Zhang, M., Chen, B., et al. (2022). Fluid geochemistry and geothermal anomaly along the yushu-ganzi-xianshuihe fault system, eastern Tibetan plateau: Implications for regional seismic activity. J. Hydrol. X. 607, 127554. doi:10.1016/j.jhydrol.2022.127554
Manga, M., and Wang, C. Y. (2015). Earthquake hydrology, treatise on geophysics. Amsterdam: Elsevier.
Martinelli, G., and Tamburello, G. (2020). Geological and geophysical factors constraining the occurrence of earthquake precursors in geofluids: A review and reinterpretation. Front. Earth Sci. 8, 596050. doi:10.3389/feart.2020.596050
Nakagawa, K., Yu, Z. Q., Berndtsson, R., and Hosono, T. (2020). Temporal characteristics of groundwater chemistry affected by the 2016 Kumamoto earthquake using selforganizing maps. J. Hydrol. X. 582, 124519. doi:10.1016/j.jhydrol.2019.124519
Pang, Z. H., Kong, Y. L., Li, J., and Tian, J. (2017). An isotopic geoindicator in the hydrological cycle. Procedia Earth Planet. Sci. 17, 534–537. doi:10.1016/j.proeps.2016.12.135
Rosen, M. R., Binda, G., Archer, C., Pozzi, A., Michetti, A. M., and Noble, P. J. (2018). Mechanisms of earthquake-induced chemical and fluid transport to carbonate groundwater springs after earthquakes. Water Resour. Res. 54, 5225–5244. doi:10.1029/2017wr022097
Rouabhia, A., Djabri, L., Hadji, R., Baali, F., and FehdiHani, C. A. (2012). Geochemical characterization of groundwater from shallow aquifer surrounding Fetzara Lake NE Algeria. Arab. J. Geosci. 5, 1–13. doi:10.1007/s12517-010-0202-6
Scholz, C. H., Sykes, L. R., and Aggarwal, Y. P. (1973). Earthquake prediction: A physical basis. Science 181, 803–810. doi:10.1126/science.181.4102.803
Shi, Z. M., and Wang, G. C. (2017). Evaluation of the permeability properties of the Xiaojiang Fault Zone using hot springs and water wells. Geophys. J. Int. 209 (3), 1526–1533. doi:10.1093/gji/ggx113
Skelton, A., Andren, M., Kristmannsdottir, H., Stockmann, G., Morth, C. M., Sveinbjornsdottir, A., et al. (2014). Changes in groundwater chemistry before two consecutive earthquakes in Iceland. Nat. Geosci. 7, 752–756. doi:10.1038/ngeo2250
Tang, X. C., Zhang, J., Pang, Z. H., Hu, S., Wu, Y., and Bao, S. (2017). Distribution and Genesis of the eastern Tibetan Plateau geothermal belt, Western China. Environ. Earth Sci. 76 (1), 31. doi:10.1007/s12665-016-6342-6
Thomas, D. (1988). Geochemical precursors to seismic activity. Pure Appl. Geophys. 126, 241–266. doi:10.1007/BF00878998
Tian, J., Pang, Z. H., Guo, Q., Wang, Y., Li, J., Huang, T., et al. (2018). Geochemistry of geothermal fluids with implications on the sources of water and heat recharge to the Rekeng high-temperature geothermal system in the Eastern Himalayan Syntax. Geothermics 74, 92–105. doi:10.1016/j.geothermics.2018.02.006
Tian, J., Pang, Z. H., Wang, Y. C., and Guo, Q. (2019). Fluid geochemistry of the Cuopu high temperature geothermal system in the eastern Himalayan syntaxis with implication on its Genesis. Appl. Geochem. 110, 104422. doi:10.1016/j.apgeochem.2019.104422
Tian, J., Pang, Z., Liao, D. W., and Zhou, X. (2021). Fluid geochemistry and its implications on the role of deep faults in the Genesis of high temperature systems in the eastern edge of the Qinghai Tibet Plateau. Appl. Geochem. 131, 105036. doi:10.1016/j.apgeochem.2021.105036
Unsworth, M. J., Jones, A. G., Wei, W., Marquis, G., Gokarn, S. G., and Spratt, J. E. (2005). Crustal rheology of the Himalaya and Southern Tibet inferred from magnetotelluric data. Nature 438 (7064), 78–81. doi:10.1038/nature04154
Wang, B., Zhou, X. C., Zhou, Y. S., Yan, Y., Li, Y., Ouyang, S., et al. (2021). Hydrogeochemistry and precursory anomalies in thermal springs of Fujian (Southeastern China) associated with earthquakes in the Taiwan Strait. Water 13, 3523. doi:10.3390/w13243523
Wang, C. Y., Cheng, L. H., Chin, C. V., and Yu, S. B. (2001). Coseismic hydrologic response of an alluvial fan to the 1999 Chi-Chi earthquake, Taiwan. Geol. 29 (9), 831–834. doi:10.1130/0091-7613(2001)029<0831:chroaa>2.0.co;2
Wang, E. Q., Meng, K., Xu, G., Li, T. M., Ren, J. W., Qiao, X., et al. (2018). Cenozoic two-stage obduction of the Indian subcontinent: On the interaction between the Indian Ocean, Tethyan and Eurasian plates. Acta Pet. Sin. 34 (7), 1867–1875. in Chinese.
Wang, M., Shen, Z. K., Gan, W. J., Liao, H., Li, T. M., Ren, J. W., et al. (2008). GPS monitoring of temporal deformation of the Xianshuihe fault. Sci. Chi. Ser. D-Earth Sci. 51, 1259–1266. doi:10.1007/s11430-008-0095-3
Wang, M., and Shen, Z. K. (2020). Present-day crustal deformation of continental China derived from GPS and its tectonic implications. J. Geophys. Res. Solid Earth 125 (2), 1–22. doi:10.1029/2019JB018774
Wang, P., Song, X., Han, D., Zhang, Y., and Liu, X. (2010). A study of root water uptake of crops indicated by hydrogen and oxygen stable isotopes: A case in shanxi province, China. Agric. Water Manag. 97, 475–482. doi:10.1016/j.agwat.2009.11.008
Xia, J. W., and Zhu, M. (2020). Study on tectonic characteristics and activity of middle section of Jinshajiang main fault zoon. Yangtze River 51 (5), 131–137. in Chinese.
Xu, X. X., Ji, L. Y., Jiang, F. Y., and Zhang, W. T. (2020). Study on current activity features of Jinshajiang Fault Zone based on GPS and small earthquakes. J. Geod. Geodyn. 40 (10), 1062–1067. in Chinese. doi:10.14075/j.jgg.2020.10.013
Yan, R., Woith, H., and Wang, R. (2014). Groundwater level changes induced by the 2011 Tohoku earthquake in China mainland. Geophys. J. Int. 199, 533–548. doi:10.1093/gji/ggu196
Yan, Y. C., Liu, F. L., Guo, L. S., Zhou, X. C., Ouyang, S. P., Li, J. C., et al. (2021). Hydrogeochemical characteristics of the hot springs in the longmenshan fault zone. J. Seismol. Res. 44 (2), 96–110. in Chinese. doi:10.3969/j.issn.1000-0666.2021.02.005
Yang, T. F., Wen, H. Y., Fu, C. C., Lee, H. F., Lan, T. F., Chen, A. T., et al. (2011). Soil radon flux and concentrations in hydrothermal area of the tatun volcano group, northern taiwan. Geochem. J. 45 (6), 483–490. doi:10.2343/geochemj.1.0144
Yi, L., Qi, J. H., Li, X., Xu, M., Zhang, X., Zhang, Q., et al. (2021). Geochemical characteristics and Genesis of the high-temperature geothermal systems in the north section of the Sanjiang Orogenic belt in southeast Tibetan Plateau. J. Volcanol. Geotherm. Res. 414 (2021), 107244. doi:10.1016/j.jvolgeores.2021.107244
Yin, A., and Harrison, T. M. (2003). Geologic evolution of the Himalayan-Tibetan orogen. Annu. Rev. Earth Planet. Sci. 28 (28), 211–280. doi:10.1146/annurev.earth.28.1.211
Yuce, G., Italiano, F., and D'Alessandro, W. (2014). Origin and interactions of fluids circulating over the Amik Basin (Hatay-Turkey) and relationships with the hydrologic, geologic and tectonic settings. Chem. Geol. 388, 23–39. doi:10.1016/j.chemgeo.2014.09.006
Zhang, C. S., Zhang, Y. C., and Wu, M. L. (2003). Study on relationship between earthquake and hydro-geochemistry of groundwater in southern part of North-South earthquake belt in China. J. Geomechaics 9 (1), 21–30. in Chinese.
Zhang, J., Li, W., Tang, X., Tian, J., Wang, Y., Guo, Q., et al. (2017). Geothermal data analysis at the high-temperature hydrothermal area in Western Sichuan. Sci. China Earth Sci. 08 (60), 1507–1521. doi:10.1007/s11430-016-9053-2
Zhang, M. L., Guo, Z., Xu, S., Barry, P. H., Sano, Y., Zhang, L., et al. (2021). Linking deeply-sourced volatile emissions to plateau growth dynamics in southeastern Tibetan Plateau. Nat. Commun. 12, 4157. doi:10.1038/s41467-021-24415-y
Zhang, W., Wang, G. L., Xing, L. X., and Zhao, J. (2019). Geochemical response of deep geothermal processes in the Litang region, Western Sichuan. Energy Explor. Exploitation 2 (37), 626–645. doi:10.1177/0144598718812550
Zhao, L. F., Xie, X. B., He, J. K., Tian, X., and Yao, Z. X. (2013). Crustal flow pattern beneath the Tibetan Plateau constrained by regional Lg-wave Q tomography. Earth Planet. Sci. Lett. 383, 113–122. doi:10.1016/j.epsl.2013.09.038
Zhou, X. C., Liu, L., Chen, Z., Cui, Y., and Du, J. (2017). Gas geochemistry of the hot spring in the Litang fault zone, Southeast Tibetan Plateau. Appl. Geochem. 79, 17–26. doi:10.1016/j.apgeochem.2017.01.022
Zhou, X. C., Wang, W. L., Li, L. W., JianMin, H., LanTian, X., ZhongPing, L., et al. (2020a). Geochemical features of hot spring gases in the Jinshajiang-Red River fault zone, Southeast Tibetan Plateau. Acta Pet. Sin. 36 (7), 2197–2214. (in Chinese). doi:10.18654/1000-0569/2020.07.18
Zhou, Z. H., Tian, L., Zhao, J., Wang, H., and Liu, J. (2020b). Stress-related pre-seismic water radon concentration variations in the Panjin observation well, China (1994–2020). Front. Earth Sci. (Lausanne). 8. doi:10.3389/feart.2020.596283
Zhou, Z. H., Zhong, J., Zhao, J., Yan, R., Tian, L., and Fu, H. (2021). Two mechanisms of earthquake-induced hydrochemical variations in an observation well. Water 13 (17), 2385. doi:10.3390/w13172385
Keywords: thermal spring, hydrogeochemistry, seismic activity, jinshajiang fault zone, southeast Tibetan plateau
Citation: Liu J, Zhou X, Li Y, He M, Li J, Dong J, Tian J, Yan Y, Ouyang S and Liu F (2023) Relationship between hydrogeochemical characteristics of hot springs and seismic activity in the Jinshajiang fault zone, Southeast Tibetan Plateau. Front. Earth Sci. 10:1015134. doi: 10.3389/feart.2022.1015134
Received: 09 August 2022; Accepted: 25 October 2022;
Published: 16 January 2023.
Edited by:
Maodu Yan, Institute of Tibetan Plateau Research, Chinese Academy of Sciences (CAS), ChinaReviewed by:
Vivek Walia, National Center for Research on Earthquake Engineering, TaiwanDongliang Liu, Chinese Academy of Geological Sciences (CAGS), China
Copyright © 2023 Liu, Zhou, Li, He, Li, Dong, Tian, Yan, Ouyang and Liu. This is an open-access article distributed under the terms of the Creative Commons Attribution License (CC BY). The use, distribution or reproduction in other forums is permitted, provided the original author(s) and the copyright owner(s) are credited and that the original publication in this journal is cited, in accordance with accepted academic practice. No use, distribution or reproduction is permitted which does not comply with these terms.
*Correspondence: Xiaocheng Zhou, emhvdXhpYW9jaGVuZzE4OEAxNjMuY29t; Ying Li, c3ViZHVjdGlvbjZAaG90bWFpbC5jb20=