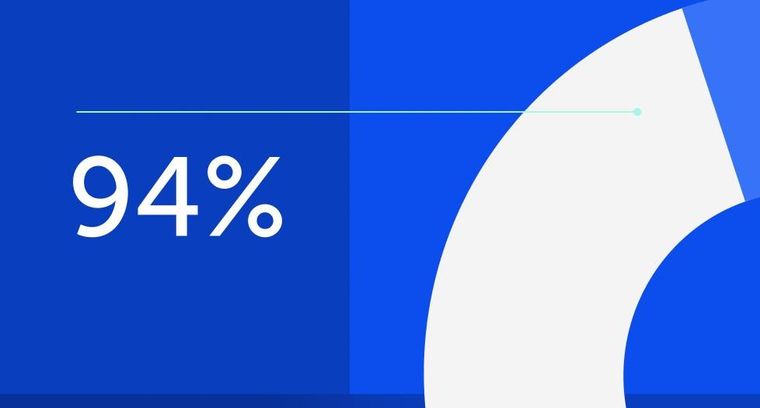
94% of researchers rate our articles as excellent or good
Learn more about the work of our research integrity team to safeguard the quality of each article we publish.
Find out more
REVIEW article
Front. Earth Sci., 05 December 2022
Sec. Geochemistry
Volume 10 - 2022 | https://doi.org/10.3389/feart.2022.1011717
It is challenging to evaluate the relevance of any given chemical system or geological environment to the origin of life. At the same time, life is the product of prebiotic chemistry that took place in some environment. We may attempt to quantify the probability landscape of organic systems and their host environments on prebiotic worlds as a preliminary step to solving the origin of life. Mapping out the environments and chemical systems of prebiotic habitable worlds requires an integration of at least two fields: prebiotic chemistry, which can discover relevant systems of reactions; and geosciences, which can identify likely planetary environments in which prebiotic systems can develop. However, parallel exploration of prebiotic environmental conditions and chemical systems is inefficient given the immense parameter space available. Here, we propose to emphasize the combined experimental study of prebiotic systems and their proposed host environments, which we term interference chemistry. Environmental variables may interfere either constructively, neutrally, or destructively with specific pathways of organic chemical synthesis, as tracked by e.g., yields or rates of reactions. In turn, prebiotic chemical systems may modify the wider environment, e.g., bulk solution chemistry. Interference chemistry therefore offers an efficient way to construct, describe, and discover prebiotic environmental scenarios, which should in turn assist us assess plausibility for origin of life scenarios.
Life relies upon reactions between organic molecules that do not proceed in the absence of enzymes (Benkovic and Hammes-Schiffer, 2003). In doing so, biology exploits energy sources in the environment that abiotic chemical pathways fail to eliminate. However, enzyme assembly without biochemical intervention has not yet been observed in naturally occurring settings. Therefore, whilst almost everything about the origin of life remains uncertain, we can be sure that organic chemical pathways on the prebiotic Earth, occurring at least to begin with in the absence of enzymes, differed hugely from those observed today.
The diversity and prevalence of organic molecules on prebiotic Earth will have depended crucially on their stability and interactions with habitable—but uninhabited—geochemical environments. It is therefore necessary at some level to link geoscience constraints on planetary environments with experimentally demonstrable chemical systems when defining a prebiotic scenario (Schwartz, 2013). This situation applies both to scenarios simply describing environmental chemistry and those that attempt to explain the emergence of biochemistry.
Prebiotic scenarios must describe reaction pathways (collectively a chemical system) hosted in one or more geochemical environments. Prebiotically plausible syntheses have now been published for membranes, information-storing polymers, and compounds that appear universally in extant metabolism, and systems chemistry research linking some of these pathways has been pursued (Deamer and Oro, 1980; Lebouteiller and KuassiviFerlet, 2005; Griffith and Vaida, 2012; Ritson and Sutherland, 2012; Barge et al., 2014; Damer and Deamer, 2015; Patel et al., 2015; Islam and Powner, 2017; Milshteyn et al., 2018; Barge et al., 2019; Bonfio et al., 2019; Morasch et al., 2019; Pasek, 2019; Wu and Sutherland, 2019; Bonfio et al., 2020; Liu et al., 2020; Yadav et al., 2020). New discoveries about early Earth geochemistry offer constraints on the possible environmental settings for these systems (see Section 3, Table 1). Despite this progress, substantial challenges remain with linking these promising chemical and geological constraints into prebiotically plausible scenarios.
Experiments are necessarily run under controlled conditions that are generally absent in naturally occurring environmental scenarios. The mismatched complexity between experiments investigating prebiotic chemistry and naturally occurring environments may be seen as broadly problematic (Shapiro, 1984). On the other hand, this discrepancy might be viewed as an opportunity for great progress. In order to make that progress, we require some broad agreement on the nature of the problem, the tools at our disposal, and the way in which discrete approaches to the study of prebiotic chemistry should interact.
Firstly, it is important to state that prebiotic systems experimentation is often carefully designed in order to 1) obtain a result in the timeframe of a project, 2) understand the reaction(s) at hand, and 3) to satisfy the burden of proof that is typically expected for a novel chemical synthesis. Including the complete inorganic and organic complexity inherent to a naturally occurring environment in a novel synthesis experiment is not conducive to these aims—especially to understanding the novel chemistry being explored in any given experiment. However, as an understanding of plausible prebiotic chemistry accumulates, increasingly multi-faceted approaches may usefully be pursued to evaluate their efficacy. In particular, it is now increasingly common to study a series of linked reactions—prebiotic systems chemistry (Islam and Powner, 2017)—and, separately, the efficacy of those prebiotic systems in the context of environmental perturbation (Miller, 1987; Robertson et al., 1996; Miyakawa et al., 2002; Todd et al., 2019). What is currently lacking is a widely shared and systematic approach to recording, reporting, and ultimately leveraging insights from interference chemistry. Here, we present a formalisation of the latter approach: interference chemistry.
We define interference chemistry as the study of interactions between a prebiotic system and a host environment (Figure 1). The environmental scenario encompasses the pressure and temperature conditions at which reactions take place as well as environmental context, e.g., atmospheric, aqueous and substrate composition, environmental geometry, fluid dynamics, timescales, etc. Prebiotic systems encompass abiotic organic chemical reactions, which may or may not have synthesized molecules of purported relevance to the origin of life. Natural environments in which prebiotic systems could have developed are messy, containing many spectator ions, mineral phases, and spatially and temporally variable physical processes, e.g., wet/dry cycles. Each of these environmental variables may interfere with prebiotic systems.
FIGURE 1. The scientific context of interference chemistry. In prebiotic systems chemistry, an experimentally verified synthesis is plausible if all reactants may have co-occurred on early Earth in one or several linked environments (a scenario). In geoscience, theoretical and analytical constraints are leveraged to describe ancient environments in which prebiotic chemistry may have taken place. Interference chemistry is the interface between prebiotic chemistry and geoscience: the response of a chemical system across a range of apparently self-consistent environmental factors. From an interference chemistry perspective, a prebiotic scenario is plausible if a prebiotic system proves robust to those interferences associated with a proposed environmental setting. A critical caveat is the fact that constraints from prebiotic chemistry and geoscience are not final, but instead should be weighted by the evidence available. Interference chemistry may therefore be leveraged to quantify and compare prebiotic plausibility for scenarios given the available chemical and geological evidence, but can only ever yield results that are as certain or uncertain as the input data.
Interference chemistry involves tracking some measurable outcome of inorganic (environmental or chemical) and abiotic organic (potentially prebiotically relevant) processes. This outcome may be as complex and broad as the overall chemical make-up of an environment of interest (this may be local, e.g., a pond, or global, e.g., a planetary atmosphere) or as simple as the yield of a reaction deemed to be of particular interest. Where the goal is simply to describe in detail plausibly prebiotic environments then individual interferences need not be categorized as constructive or destructive. The outcome in this general case is of interest regardless of the details, as we are learning about which environments may have prevailed on early Earth.
Alternatively, we may test the performance of the system given some environment, e.g., yield of a specific product. In this case we require some way to categorize the outcomes and implications of interference chemistry. Take the example of a reaction pathway known to produce a molecule of known prebiotic interest, e.g., synthesis of RNA in water, and temperature—a universal environmental parameter. In this case, interference may be constructive, e.g., the warming of an environment to melt ice and allow aqueous reactions to occur; destructive, e.g., the continued heating of the environment and eventual thermal degradation of organic products; or neutral, having no effect on the pathway at hand, e.g., insensitivity of a particular reaction to temperature across some range of measurement.
Combining the logic of systems and interference chemistry approaches, we propose a new over-arching definition of prebiotic plausibility: a prebiotically plausible scenario is one in which the proposed prebiotic system is 1) demonstrable in the laboratory setting, 2) utilises only reactants and processes that likely co-occurred in geological environments. Where the scenario is to be extended from merely a description of plausible environmental chemistry to a plausible synthesis for a specific molecule or set of molecules (or complex chemical interaction, or life itself) then additional constraints must be met: 3) remaining efficacious given interference by the local environment, or suite of connected environments, and 4) producing sufficiently high yields at each step to continue—without arbitrary intervention—through to completion.
Interference chemistry encapsulates the testing of point (3): the direct incorporation of multiple environmental factors into successful prebiotic systems, and then—by a process of iteration—identifying prebiotically plausible parameter space (Sasselov et al., 2020). As such, interference chemistry draws on distinct types of information to geoscience or systems chemistry alone (Figure 2). Moreover, by leveraging insights unique to geoscience, systems chemistry, and interference chemistry, quantitative constraints may be obtained for scenarios that address each component of prebiotic plausibility, as defined above (Figure 3). Crucially, this definition of prebiotic plausibility applies to the conjunction of chemistry and environment, and only provides information within certain logical bounds. For example, if some proposed chemistry fails in environment A (contradicting points 1 and 3), it might still work in environment B, and if some species X needed for the chemistry is unlikely to be present in environment A (contradicting 2), it says nothing about whether species X is likely to be found in environment B.
FIGURE 2. Venn-diagram illustrating the constraints that can be achieved on prebiotic scenarios with different scientific approaches. Geoscience can constrain localised environmental chemistry as well as planetary-scale boundary conditions via the analysis of preserved rocks or with theoretical models, while systems chemistry can identify reactions leading to pre-biological molecules. An interference chemistry approach to (i) geoscience involves the systematisation (self-consistency, ranges of uncertainty) of existing constraints, and to (ii) systems chemistry involves the application of interferences to experiments and collation of resulting effect sizes (delta yield/rate, and so on). Where geoscientific, systems chemistry, and interference chemistry constraints all indicate that a scenario is robust, an evaluation of prebiotic plausibility can be assessed (subject to change in all three types of input).
FIGURE 3. Schematic example of interference chemistry workflow to determine prebiotic plausibility. (A) Initial experimental conditions for a proposed prebiotic reaction step or system that has been considered successful. (B) Reported range of conditions for various types of environment (i, ii, and iii). Outside of the reported range may be considered prebiotically implausible in a strict sense, but this should never be considered final given the ever-improving geoscientific knowledge base. (C) Results from interference chemistry exploration of the reaction/system under consideration. Some experimental initial conditions emerge as being close to the local maximum scenario, e.g., in the parameter space encompassed by environment ii. In other cases, significant yield improvement is observed by traversing parameter space, e.g., traversing the conditions that occur in environment i. More information might be gained by evaluating the delta yield that results from applying numerous interferences, i.e., approximating more closely the messy environments that occur in nature. (D) The combination of experimental, environmental, and interference chemistry constraints yields one way to quantify prebiotic plausibility for any given scenario—subject to propagated chemical/geological uncertainties. (E) Prebiotic plausibility could be further formalised in terms of probability. In our case, this would be in terms of yield: the product of probability that an environmental condition may occur, combined with the yield at those conditions.
An interference chemistry study is distinct from a chemical or geoscience study in that it does not set out to demonstrate a novel synthetic system or to place novel constraints on an environment, but to systematically examine the response of the chemical system to relevant environmental conditions (Figure 3), i.e., a multi-factor experimental approach. In Figure 3 we have illustrated for the sake of simplicity a (schematic) two-dimensional parameter space showing interactions between pH and anion composition. In reality, prebiotic chemical systems are high-dimensional systems. Visualizations of real systems will therefore be correspondingly more complex. Tackling and overcoming this complexity is the main opportunity and difficulty of implementing interference chemistry.
Implementing interference chemistry requires overcoming three main challenges: 1) that mapping destructive interferences necessarily means running experiments that produce low yields of a desired product—this is a challenge to a culture where we may be encouraged to report only on successful experiments; 2) the volume of environmental parameter space to be explored is too large to be exhausted in the lab; and 3) the reality that what is considered environmentally plausible is uncertain, and subject to continual advances being made in geoscience.
Screening for multi-factor interactions is commonplace in the study of certain biological and chemical industrial processes (Novack, 2000), and has been commented on before in the context of synthetic biology (Moschner et al., 2022) and even in prebiotic chemistry (Cleaves, 2013). The outstanding question, then, is not merely whether interference chemistry might be a useful pursuit, but whether its widespread implementation is both feasible and justifiable.
We examine the experimental feasibility of interference chemistry by considering both published studies that might be categorized as investigating the interference chemistry prebiotic systems, and by briefly reviewing what is currently known about early Earth environmental conditions. We propose distinct burdens of proof for progress in the study of prebiotic systems, environments, and interference chemistry, respectively, arguing that agreement on what these different approaches should individually seek to achieve has the potential to unlock information flow between disparate fields that collectively seek to understand the environmental chemistry of prebiotic Earth.
There are numerous published studies that might be considered as falling under the umbrella of interference chemistry. These studies share the common trait of having taken a particular prebiotically relevant species/reaction/system and systematically exploring its response to environmental variables of interest. We consider several specific examples, from prebiotic compartmentalisation, to UV transmission, to bystander-ion-driven catalysis.
Prebiotic membrane-bound compartments have previously been subjected to a diverse range of environmental analogue conditions—both in the lab, and in the field. An outcome of these studies is that certain simple prebiotically plausible amphiphiles will spontaneously assemble into compartments in freshwater and modern hot spring systems but will not do so in seawater (Milshteyn et al., 2018). Meanwhile, other classes of amphiphiles are quite robust to the interferences present in seawater (Jordan et al., 2019a). Much is also known about the response of these structures to pH, ionic strength, and temperature (Yanagawa et al., 1988; Terasawa et al., 2012; Joshi et al., 2017; Milshteyn et al., 2018; Jordan et al., 2019a; Jordan et al., 2019b; Lopez and Fiore, 2019; Bonfio et al., 2020), from which a picture is emerging of which compartments may plausibly have been present in end-member prebiotic environments.
Ultra-violet light is a crucial component of several recently proposed reaction schemes, acting to drive otherwise challenging steps in a high yielding and stereoselective manner (Ritson and Sutherland, 2012; Patel et al., 2015; Rimmer et al., 2018; Rimmer et al., 2021). However, many by-stander species may attenuate the transmission of UV light in geochemically realistic complex aqueous solutions. Ranjan et al. (2021) found that Fe-containing species in e.g., ferrocyanide lakes are efficient at attenuating UV transmission, diminishing the potential for UV-driven constructive organic synthesis, but similarly slowing UV-driven degradation of other molecules that may go on to accumulate and constructively interact along different paths, e.g., meteoritic nucleobases (Pearce et al., 2017). The existing body of work on compartment stability and UV light transmission in the presence of varying environmental interferences provides a model for future work on more complex systems.
The projects outlined above are strong examples of how interference chemistry may be experimentally tractable in some cases. However, there are other examples where novel combinations of prebiotic systems and geological conditions yielded surprising constructive interferences, without necessarily having focussed on parameter space exploration. An important example are the cases of phosphate and borate in prebiotic chemistry. These topics have received a great deal of attention previously (Fernández-García et al., 2017; Liu et al., 2019), and we make no attempt to summarise the breadth of previous discussion on the issue here. Instead, we point to the fact that these anions have proved diversely useful in prebiotic chemical synthesis when used at high concentrations. Phosphate in these experiments has utility either as a general acid-base catalyst or as a chemical buffer, greatly enhancing reaction selectivity and yield (Powner and Sutherland, 2010; Patel et al., 2015; Islam and Powner, 2017; Morasch et al., 2019). Similarly, borate acts to make the formidably messy formose reaction far more selective for the synthesis of compounds of apparent prebiotic interest, e.g., by stabilizing, ribose sugar (Benner et al., 2012; Kim et al., 2016).
The above constructive interferences by phosphate and borate in prebiotic chemical synthesis occur only when these anions are present at high concentrations. Both phosphate and borate are scarce in most modern aqueous environments, which may at first glance appear to damage the plausibility of prebiotic scenarios relying on the use of either (Table 1). However, geochemical scenarios are now being independently developed in which phosphate and/or borate may indeed have been present at the high concentrations required by published syntheses (>10 mM), reshaping our view of what may count as prebiotically plausible (Rasmussen et al., 2021; Ingalls et al., 2022).
If the once prevailing view of limited phosphate and/or borate availability on early had been used as a stringent guide for experimental progress, the valuable constructive roles in prebiotic organic synthesis of anions which are currently scarce in surface environments—but might not always have been—may never have been fully recognized. Examples of such unexpected interactions inform us that it is crucial for prebiotic environmental constraints to be explored in the intentionally broad manner that we hope interference chemistry can help to promote. That is to say, prebiotic plausibility only applies to a combination of both chemistry and environment, and is a matter of probabilities and degrees of confidence. Wandering outside what we think of as plausible, even as defined here, can be immensely scientifically rewarding. However, this type of research is limited not only by experimental practicality, but also by our knowledge of which specific interferences are associated with prebiotic environments—an uncertainty that makes it difficult to bridge lab and environment (Figure 1).
Much is uncertain about the nature of prebiotic Earth. The timeline, surface conditions, and basic geology of the planet in this state are all difficult to ascertain, owing to a lack of a rock record for the first half billion years of Earth history (4.45–4.0 Ga—early Earth, from here-on-in). Via a combination of extrapolation and theoretical study, there is consensus that early Earth atmosphere was anoxic, with somewhat clement surface temperatures, and that there were surface oceans rich in dissolved iron (Valley et al., 2002; Zahnle et al., 2007; Shaw, 2008; Harrison, 2009; Sessions et al., 2009; Planavsky et al., 2011; Partin et al., 2013; Scott et al., 2013; Lyons et al., 2014; Planavsky et al., 2014; Ge et al., 2018). Much more uncertain is the extent of emergent land (of any rock type), the composition and mere existence of continents, whether or not the atmosphere was highly reducing, and if those generically habitable surface conditions leaned towards global icehouse or hothouse conditions (Valley et al., 2002; Barley et al., 2005; Rollinson, 2007; Zahnle et al., 2007; Harrison, 2009; Sleep, 2010; Bell et al., 2011; Marchi et al., 2014; Roberts and Spencer, 2014; Thomassot et al., 2015; Hastie et al., 2016; Johnson et al., 2017; Smit and Mezger, 2017; O’Neill et al., 2018).
In Table 1, we summarize the geochemical characteristics of commonly invoked prebiotic environmental scenarios for early Earth. Our compendium provides an overview of environmental parameters that can be used as a starting point for interference chemistry research, e.g., by the exposure of a proposed prebiotic systems to a set of environmental interferences.
We rely on modern analogues that have been studied in detail as the basis of our compendium, supplemented where possible with what is known about each specific environmental interference on early Earth. Some species found in key environments for prebiotic chemistry are highly sensitive to surface conditions yet have not received detailed modelling or experimental studies relevant to early Earth conditions. In Table 1, such especially uncertain estimates are shaded in orange. Conversely, estimates derived from studies designed to investigate prebiotic and/or early Earth (Archean) conditions are shaded in green.
As a key example of these uncertainties, we highlight alkaline lakes. Restricted subaerial and confined submarine crustal environments are increasingly proposed in scenarios for the origin of life (Westall et al., 2018; Deamer et al., 2019; Toner and Catling, 2019; Toner and Catling, 2020). However, there are many open questions about the interference chemistry of such basins on early Earth. Sulphate concentrations in alkaline lakes are very high in the modern, approaching molal concentrations (Table 1). However, such systems are unlikely to have generated the same sulphate concentrations under an anoxic atmosphere and in the absence of sulphide-oxidising bacteria (Visscher et al., 2020). Similarly, dissolved iron concentrations are directly linked to oxidising power in the environment. With soluble ferrous iron (Fe2+) being dominant in early Earth surface environments, it is likely that prebiotic analogues of modern surficial aqueous environments may have been fed by iron-rich inflow—although, iron carbonate mineral precipitation may have acted to limit final concentrations (Toner and Catling, 2020).
Finally, atmospheric pCO2 of early Earth is poorly constrained, rendering Dissolved Inorganic Carbon (DIC) estimates uncertain for all environments (Krissansen-Totton et al., 2018; Kadoya et al., 2020). This uncertainty is problematic, as pCO2 and DIC are a major control on the pH and overall chemistry of restricted subaerial solutions in equilibrium with the atmosphere, e.g., phosphate content of soda lakes (Toner and Catling, 2020). Available DIC is also required for abiotic production of organic carbon in many proposed prebiotic environments (Lang et al., 2018).
On the other hand, dedicated studies on ancient weathering processes and ocean chemistry more tightly constrain several key interferences in early river and ocean water (see Table 1). However, for most environments in Table 1, we do not highlight interferences as especially likely or unlikely to be reflective of plausibly prebiotic environments: for now, we simply do not know either way.
Our survey is far from all-encompassing. Mapping out environmental chemistry on Earth prior to the rise of life is a huge undertaking—independent of the specific question of where life first began, and currently lacking deep integration with organic prebiotic chemistry. The critical next step is to understand more deeply the feedbacks between inorganic planetary chemistry and prebiotic organic chemistry, i.e., the defining features of prebiotic Earth. It is this broad base of knowledge that we argue interference chemistry can contribute to.
The interference chemistry framework has at its core the two-way flow of information between prebiotic chemistry and the study of early Earth conditions: whereby the reactions that drove the origin of life—as well as those that did not, but still widely occurred—will have acted to shape both local environments and ultimately global planetary conditions. At the same time, prevailing environmental conditions will have determined the plausibility and feasibility of proposed origin of life scenarios. Interference chemistry may therefore be leveraged in pursuit of dual constraints on 1) wider environmental conditions at the dawn of life and 2) the specific environments in which life may have emerged.
From the perspective of planetary science, it is often much easier to say what interferences were not present than to establish those which were, and to establish limited global constraints on environmental conditions, rather than to specify the detailed local environmental conditions needed to constrain origin of life scenarios. Especially when studying the ancient past, geoscience may be more suited to obtaining constraints on processes that operate over long timescales and large length-scales. In contrast, prebiotic chemistry—especially those systems that are tractable in the lab—may largely operate under ephemeral conditions in local environments (Table 2). Published environmental constraints may therefore fail to readily describe local environments that may have hosted prebiotic chemistry. Moreover, geoscience constraints are constantly updated as new data is made available. Formulating an assessment of prebiotic plausibility with environmental constraints may therefore be flawed, creating an unhelpful barrier to synthetic chemistry exploration.
Our proposal for the recognition of interference chemistry as a discrete approach (Figure 1) is intended to smooth out the difficulties that may arise when judging systems chemistry results with a geochemical filter. Geoscience should continue to strive to understand the initial environmental conditions of early Earth. Meanwhile, projects explicitly pursuing interference chemistry can examine the interplay between state-of-the-art prebiotic systems and knowledge of the early Earth. In doing so, insights should be gained about which processes are viable based on current knowledge, offering up new routes for biomolecule synthesis and novel perspectives on early environmental chemistry. Here, we suggested a path for systematic exploration of interference chemistry parameter space. This exploration can be systematic without being comprehensive (which represents an impossible ideal).
It is worth explicitly stating that the implementation of interference chemistry might be considered as a valuable opportunity to diminish the extent of geochemical gatekeeping in prebiotic chemistry. The lessons of the past inform us that even widely held views about what is plausible from a geochemical perspective should not be used to inhibit progress in the field of systems chemistry, as those conceptual boundary conditions may 1-day change. However, given that we must ultimately strive to find a scenario for the origin of life that is both experimentally verified and consistent with our understanding of environmental chemistry on the early Earth, interference chemistry offers a conceptual space where attempting to fulfil this high burden of proof seems reasonable.
Interferences provide a sensible metric to quantify whether or not a chemical system is valuable on its own merits, e.g., can it provide the products we desire, exist in an environment known to exist in nature, and function in a timeframe that is testable in the lab? However, quantifying the relevance of a given system to the origin of life is a much more challenging prospect. We might contemplate metrics such as degrees of self-organisation, self-catalysis, longevity, discovery of new function, and so on, but the challenge here is formidable. Until prebiotic synthesis can in any sense bridge the gap between chemical and biological, it seems more tractable at present to tackle the smaller—but still vast—problem of mapping out prebiotic environments and their organic chemical systems.
We have argued that interference chemistry provides a way to explore, make sense of, and leverage geochemical complexity in a prebiotic context, in terms of evaluating the plausibility of specific regions of chemical-environmental parameter space with respect to a specific system of reactions. However, there are numerous theoretical issues branching from an interference chemistry approach that will demand future attention. One such issue is data collection versus integration.
Interference chemistry as we have pitched it could help to collect increasingly prebiotically relevant data. However, we are still then faced with evaluating the relative plausibility of one prebiotic scenario versus another, some of which may be mutually exclusive, i.e., integrating disparate data. More complex forms of environmental and chemical interference then emerge for us to consider in our quest to map out the nature of prebiotic Earth, related to the dynamics, timescales, and sizes of environments.
For example, if we propose that one environment (e.g., glacier ice) hosting a particular prebiotic chemistry existed on early Earth, could it have co-existed with another environment (e.g., high pCO2 atmosphere) invoked in another step of the same proposed sequence of events? Are these environmental conditions mutually exclusive? Similarly, would the prebiotic chemistry of one environment have in fact inhibited that proposed to have occurred in another, after considering local/regional/global feedbacks? Can we be sure that the regions of parameter space involved in a given scenario—explored and tested for plausibility (using interference chemistry or otherwise)—remain plausible when placed into a complete planetary context?
Integrating interference chemistry constraints on the nature of prebiotic Earth will requires reaching ever further beyond the initial focus of in-situ prebiotic chemistry, e.g., considering the compositions of planetary crusts that determine local environmental diversity, and to astrophysical constraints that determine the chemistry of those crusts. There is no way to directly account for these types of interferences and boundary conditions in the context of an individual experiment. Therefore, whilst the relatively simple multi-factorial experimental design that we suggested here for interference chemistry may give us some measure of plausibility for a specific system (within available experimental or theoretical constraints at the time) it will fall short of helping us integrate resulting data from many disparate experiments.
Given the absence of empirical evidence for the nature of environments and chemistry on the prebiotic Earth, we envisage that data integration accounting for interference effects will at some level rely on linked simulations. Progress is this regard being made in several relevant areas, including the formulation of self-consistent global biogeochemical models, which are being employed to reconstruct the evolution of Earth’s surface environment over time (Lenton et al., 2018; Mills et al., 2018), and rule-based models of complex chemical systems in the modelling of physical chemistry (Goldford et al., 2019; Wołos et al., 2020; Arya et al., 2022). We view these tools as being likely essential for bridging spatial and timescales that are not tractable in the lab and for linking fields (e.g., feeding simulations of prebiotic systems into models of planetary geochemistry). Experimental and observational approaches then have the pivotal role of informing the mechanics at play in such simulations.
As with experiments, which we have focused on in this article (systems and interference chemistry), results from simulations will only be as strong as the validity of their core assumptions. Predictions made by simulations may be less unhelpful if they are left untested and, in some cases, it may not be tractable to perform an equivalent experiment in the lab. However, data generated by exoplanetary science may provide us with the empirical data needed to test planetary-scale simulation results. Exoplanetary science provides the possibility to observe worlds of a similar stellar parent body, orbital configuration, and chemical composition to Earth, of various ages. Mapping out the distribution of life in our galaxy will permit us to test origin of life scenarios using real planetary laboratories, and to build statistical descriptions of prebiotic chemistry at the planetary scale. Ultimately, we will be able to answer crucial questions: what do prebiotic Earth-like worlds look like, and how many Earth-like worlds give rise to life? These data may then enable cross-environmental data integration in prebiotic chemistry, i.e., the ability to test predictions made by planetary-scale simulations of prebiotic chemistry, and then within that context to re-evaluate which scenarios for prebiotic environments (and eventually, the origin of life) can be constructed in a truly self-consistent manner.
As far back as we have been able to reach in Earth’s past, whether through rocks or through genetics, life’s origins have remained beyond the horizon. In stark contrast, the alternative route of working from the ground up via prebiotic chemistry, aiming to build life in the laboratory, has made outstanding progress. Carefully regulated laboratory experiments have now been able to create the building blocks of life: RNA, protein and lipid precursors. These experiments place prebiotic chemistry in a firmly testable physical and chemical context, i.e., they point to specific astrophysical and geological settings where this chemistry could occur. Whilst in the lab these reactions are carried out under ideal conditions, in nature the chemistry will inevitably be messier. Nonetheless, prebiotic chemistry must have emerged from geochemistry; linking the two is therefore fundamental for establishing how rocky worlds can give rise to life.
We propose a term to describe research that explicitly investigates the robustness of prebiotic chemistry to environmental conditions: interference chemistry. By identifying the geochemical characteristics of prebiotic environments, and testing prebiotic organic systems under these natural conditions, we will refine our view of the prebiotic planetary ‘wild’. In this article, we summarised previous experimental approaches fall under our definition of interference chemistry and presented an initial library of environmental conditions that could be implemented in future experiments.
Interference chemistry has the potential to help evaluate the prebiotic plausibility of a given scenario. However, this possibility must come with the overarching caveat that any and all input constraints are subject to change, as our knowledge of fundamental physical chemistry and the nature of early Earth continuously improves. Nonetheless, seperating the burden of proof for environmental plausiblity into a separate strand of research—interference chemistry—might have the positive outcome of diminishing the role of geochemical ‘gatekeeping’ in prebiotic chemical research. The revised approach in interference chemistry, where these two fields are inherently linked, may more often lead to geoscientific constraints that inspire, rather than unproductively inhibit, exploration and innovation in the field of prebiotic chemistry.
All authors contributed equally to the conceptual aspects of the manuscript. CW wrote the manuscript and prepared the figures. All authors reviewed and edited the final manuscript.
CW acknowledges NERC and UKRI for support through a NERC DTP studentship, grant number NE/L002507/1. CW acknowledges LCLU funding support. PR is grateful for funding by the Simons Foundation (SCOL Grant #599634).
Many members of the Cambridge Leverhulme Centre for Life and Planets in the Universe and Oliver Maguire, Matt Powner, Dougal Ritson, and Helen Williams are all thanked for their constructive interference in the origin of this paper.
The authors declare that the research was conducted in the absence of any commercial or financial relationships that could be construed as a potential conflict of interest.
All claims expressed in this article are solely those of the authors and do not necessarily represent those of their affiliated organizations, or those of the publisher, the editors and the reviewers. Any product that may be evaluated in this article, or claim that may be made by its manufacturer, is not guaranteed or endorsed by the publisher.
Arya, A., Ray, J., Sharma, S., Simbron, R. C., Lozano, A., Smith, H. B., et al. (2022). An open source computational workflow for the discovery of autocatalytic networks in abiotic reactions. Chem. Sci. 13, 4838–4853. doi:10.1039/d2sc00256f
Barge, L. M., Doloboff, I. J., Russell, M. J., VanderVelde, D., White, L. M., Stucky, G. D., et al. (2014). Pyrophosphate synthesis in iron mineral films and membranes simulating prebiotic submarine hydrothermal precipitates. Geochim. Cosmochim. Acta 128, 1–12. doi:10.1016/j.gca.2013.12.006
Barge, L. M., Flores, E., Baum, M. M., VanderVelde, D. G., and Russell, M. J. (2019). Redox and PH gradients drive amino acid synthesis in iron oxyhydroxide mineral systems. Proc. Natl. Acad. Sci. U. S. A. 116, 4828–4833. doi:10.1073/pnas.1812098116
Barley, M. E., Bekker, A., and Krapež, B. (2005). Late archean to early paleoproterozoic global tectonics, environmental change and the rise of atmospheric oxygen. Earth Planet. Sci. Lett. 238, 156–171. doi:10.1016/j.epsl.2005.06.062
Bell, E. A., Harrison, T. M., McCulloch, M. T., and Young, E. D. (2011). Early archean crustal evolution of the jack hills zircon source terrane inferred from Lu–Hf, 207Pb/206Pb, and Δ18O systematics of jack hills zircons. Geochim. Cosmochim. Acta 75, 4816–4829. doi:10.1016/j.gca.2011.06.007
Benkovic, S. J., and Hammes-Schiffer, S. (2003). A perspective on enzyme catalysis. Science 301, 1196–1202. doi:10.1126/science.1085515
Benner, S. A., Kim, H.-J., and Carrigan, M. A. (2012). Asphalt, water, and the prebiotic synthesis of ribose, ribonucleosides, and RNA. Acc. Chem. Res. 45, 2025–2034. doi:10.1021/ar200332w
Berndt, M. E., and Seyfried, W. E. (1990). Boron, bromine, and other trace elements as clues to the fate of chlorine in mid-ocean ridge vent fluids. Geochim. Cosmochim. Acta 54, 2235–2245. doi:10.1016/0016-7037(90)90048-p
Bonfio, C., Caumes, C., Duffy, C. D., Patel, B. H., Percivalle, C., Tsanakopoulou, M., et al. (2019). Length-selective synthesis of acylglycerol-phosphates through energy-dissipative cycling. J. Am. Chem. Soc. 141, 3934–3939. doi:10.1021/jacs.8b12331
Bonfio, C., Russell, D. A., Green, N. J., Mariani, A., and Sutherland, J. D. (2020). Activation chemistry drives the emergence of functionalised protocells. Chem. Sci. 11, 10688–10697. doi:10.1039/d0sc04506c
Bradley, A. S., Hayes, J. M., and Summons, R. E. (2009). Extraordinary 13C enrichment of diether lipids at the lost city hydrothermal field indicates a carbon-limited ecosystem. Geochim. Cosmochim. Acta 73, 102–118. doi:10.1016/j.gca.2008.10.005
Charlou, J. L., Donval, J. P., Fouquet, Y., Jean-Baptiste, P., and Holm, N. (2002). Geochemistry of high H2 and CH4 vent fluids issuing from ultramafic rocks at the rainbow hydrothermal field (36°14′N, MAR). Chem. Geol. 191, 345–359. doi:10.1016/s0009-2541(02)00134-1
Cleaves, H. J. (2013). Prebiotic chemistry: Geochemical context and reaction screening. Life 3, 331–345. doi:10.3390/life3020331
Crowe, S. A., Paris, G., Katsev, S., Jones, C., Kim, S.-T., Zerkle, A. L., et al. (2014). Sulfate was a trace constituent of archean seawater. Science 346, 735–739. doi:10.1126/science.1258966
Damer, B., and Deamer, D. (2015). Coupled phases and combinatorial selection in fluctuating hydrothermal pools: A scenario to guide experimental approaches to the origin of cellular life. Life 5, 872–887. doi:10.3390/life5010872
Deamer, D., Damer, B., and Kompanichenko, V. (2019). Hydrothermal chemistry and the origin of cellular life. Astrobiology 19, 1523–1537. doi:10.1089/ast.2018.1979
Deamer, D., and Oro, J. (1980). Role of lipids in prebiotic structures. BioSystems 12, 167–175. doi:10.1016/0303-2647(80)90014-3
Dellinger, M., Gaillardet, J., Bouchez, J., Calmels, D., Galy, V., Hilton, R. G., et al. (2014). Lithium isotopes in large rivers reveal the cannibalistic nature of modern continental weathering and erosion. Earth Planet. Sci. Lett. 401, 359–372. doi:10.1016/j.epsl.2014.05.061
Douville, E., Charlou, J. L., Oelkers, E. H., Bienvenu, P., Colon, C. F. J., Donval, J. P., et al. (2002). The rainbow vent fluids (36°14′N, MAR): The influence of ultramafic rocks and phase separation on trace metal content in mid-atlantic ridge hydrothermal fluids. Chem. Geol. 184, 37–48. doi:10.1016/s0009-2541(01)00351-5
Edmonds, H. N., and German, C. R. (2004). Particle geochemistry in the rainbow hydrothermal plume, mid-atlantic ridge. Geochimica Cosmochimica Acta 68, 759–772. doi:10.1016/s0016-7037(03)00498-8
Fernández-García, C., Coggins, A. J., and Powner, M. W. (2017). A chemist’s perspective on the role of phosphorus at the origins of life. Life 7, 31. doi:10.3390/life7030031
Ge, R., Wilde, S. A., Nemchin, A. A., Whitehouse, M. J., Bellucci, J. J., Erickson, T. M., et al. (2018). A 4463 Ma apparent zircon age from the Jack Hills (Western Australia) resulting from ancient Pb mobilization. Geology 46, 303–306. doi:10.1130/g39894.1
Goldford, J. E., Hartman, H., Marsland, R., and Segrè, D. (2019). Environmental boundary conditions for the origin of life converge to an organo-sulfur metabolism. Nat. Ecol. Evol. 3, 1715–1724. doi:10.1038/s41559-019-1018-8
Griffith, E. C., and Vaida, V. (2012). In situ observation of peptide bond formation at the water–air interface. Proc. Natl. Acad. Sci. U. S. A. 109, 15697–15701. doi:10.1073/pnas.1210029109
Hao, J., Sverjensky, D. A., and Hazen, R. M. (2017). A model for late archean chemical weathering and world average river water. Earth Planet. Sci. Lett. 457, 191–203. doi:10.1016/j.epsl.2016.10.021
Harrison, T. M. (2009). The hadean crust: Evidence from >4 Ga zircons. Annu. Rev. Earth Planet. Sci. 37, 479–505. doi:10.1146/annurev.earth.031208.100151
Hastie, A. R., Fitton, J. G., Bromiley, G. D., Butler, I. B., and Odling, N. W. A. (2016). The origin of Earth’s first continents and the onset of plate tectonics. Geology 44, 855–858. doi:10.1130/g38226.1
Hodgkinson, M. R. S., Webber, A. P., Roberts, S., Mills, R. A., Connelly, D. P., and Murton, B. J. (2015). Talc-dominated seafloor deposits reveal a new class of hydrothermal system. Nat. Commun. 6, 10150. doi:10.1038/ncomms10150
Hurwitz, S., Clor, L. E., McCleskey, R. B., Nordstrom, D. K., Hunt, A. G., and Evans, W. C. (2016). Dissolved gases in hydrothermal (phreatic) and geyser eruptions at yellowstone national park, USA. Geology 44, 235–238. doi:10.1130/g37478.1
Ingalls, M., Grotzinger, J. P., Present, T., Rasmussen, B., and Fischer, W. W. (2022). Carbonate-associated phosphate (CAP) indicates elevated phosphate availability in neoarchean shallow marine environments. Geophys. Res. Lett. 49, 49. doi:10.1029/2022gl098100
Islam, S., and Powner, M. W. (2017). Prebiotic systems chemistry: Complexity overcoming clutter. Chem 2, 470–501. doi:10.1016/j.chempr.2017.03.001
Johnson, T. E., Brown, M., Gardiner, N. J., Kirkland, C. L., and Smithies, R. H. (2017). Earth’s first stable continents did not form by subduction. Nature 543, 239–242. doi:10.1038/nature21383
Jones, C., Nomosatryo, S., Crowe, S. A., Bjerrum, C. J., and Canfield, D. E. (2015). Iron oxides, divalent cations, silica, and the early Earth phosphorus crisis. Geology 43, 135–138. doi:10.1130/g36044.1
Jordan, S. F., Nee, E., and Lane, N. (2019). Isoprenoids enhance the stability of fatty acid membranes at the emergence of life potentially leading to an early lipid divide. Interface Focus 9, 20190067. doi:10.1098/rsfs.2019.0067
Jordan, S. F., Rammu, H., Zheludev, I. N., Hartley, A. M., Maréchal, A., and Lane, N. (2019). Promotion of protocell self-assembly from mixed amphiphiles at the origin of life. Nat. Ecol. Evol. 3, 1705–1714. doi:10.1038/s41559-019-1015-y
Joshi, M. P., Samanta, A., Tripathy, G. R., and Rajamani, S. (2017). Formation and stability of prebiotically relevant vesicular systems in terrestrial geothermal environments. Life 7, 51. doi:10.3390/life7040051
Kadoya, S., Krissansen-Totton, J., and Catling, D. C. (2020). Probable cold and alkaline surface environment of the hadean Earth caused by impact ejecta weathering. Geochem. Geophys. Geosyst. 21. doi:10.1029/2019gc008734
Kelley, D. S., Karson, J. A., Blackman, D. K., Früh-Green, G. L., Butterfield, D. A., Lilley, M. D., et al. (2001). An off-Axis hydrothermal vent field near the mid-atlantic ridge at 30° N. Nature 412, 145–149. doi:10.1038/35084000
Kim, H., Furukawa, Y., Kakegawa, T., Bita, A., Scorei, R., and Benner, S. A. (2016). Evaporite borate-containing mineral ensembles make phosphate available and regiospecifically phosphorylate ribonucleosides: Borate as a multifaceted problem solver in prebiotic chemistry. Angew. Chem. Int. Ed. 55, 15816–15820. doi:10.1002/anie.201608001
Krissansen-Totton, J., Arney, G. N., and Catling, D. C. (2018). Constraining the climate and ocean PH of the early Earth with a geological carbon cycle model. Proc. Natl. Acad. Sci. U. S. A. 115, 4105–4110. doi:10.1073/pnas.1721296115
Lang, S. Q., Früh-Green, G. L., Bernasconi, S. M., Brazelton, W. J., Schrenk, M. O., and McGonigle, J. M. (2018). Deeply-sourced formate fuels sulfate reducers but not methanogens at lost city hydrothermal field. Sci. Rep. 8, 755. doi:10.1038/s41598-017-19002-5
Lebouteiller, V., Kuassivi, , , and Ferlet, R. (2005). Phosphorus in the diffuse interstellar medium. Astronomy \& Astrophysics, 443 509-517, doi:10.1051/0004-6361:20053448
Lenton, T. M., Daines, S. J., and Mills, B. J. W. (2018). COPSE reloaded: An improved model of biogeochemical cycling over phanerozoic time. Earth. Sci. Rev. 178, 1–28. doi:10.1016/j.earscirev.2017.12.004
Liu, Z., Rossi, J.-C., and Pascal, R. (2019). How prebiotic chemistry and early life chose phosphate. Life 9, 26. doi:10.3390/life9010026
Liu, Z., Wu, L.-F., Xu, J., Bonfio, C., Russell, D. A., and Sutherland, J. D. (2020). Harnessing chemical energy for the activation and joining of prebiotic building blocks. Nat. Chem. 12, 1023–1028. doi:10.1038/s41557-020-00564-3
Lopez, A., and Fiore, M. (2019). Investigating prebiotic protocells for A comprehensive understanding of the origins of life: A prebiotic systems chemistry perspective. Life 9, 49. doi:10.3390/life9020049
Lyons, T. W., Reinhard, C. T., and Planavsky, N. J. (2014). The rise of oxygen in Earth’s early ocean and atmosphere. Nature 506, 307–315. doi:10.1038/nature13068
Lyons, W. B., Mikucki, J. A., German, L. A., Welch, K. A., Welch, S. A., Gardner, C. B., et al. (2019). The geochemistry of englacial brine from taylor glacier, Antarctica. J. Geophys. Res. Biogeosci. 124, 633–648. doi:10.1029/2018jg004411
Marchi, S., Bottke, W. F., Elkins-Tanton, L. T., Bierhaus, M., Wuennemann, K., Morbidelli, A., et al. (2014). Widespread mixing and burial of Earth’s hadean crust by asteroid impacts. Nature 511, 578–582. doi:10.1038/nature13539
McCleskey, B., Ball, J. W., Nordstrom, D. K., Holloway, J. M., and Taylor, H. E. Water-chemistry data for selected hot springs, geysers, and streams in yellowstone national park, Wyoming, 2001-2002.Reston, Virginia USA, U.S. Geological Survey Open-File Report 2004-1316 2005. doi:10.3133/ofr20041316
Miller, S. L. (1987). Which organic compounds could have occurred on the prebiotic Earth? Cold Spring Harb. Symp. Quant. Biol. 52, 17–27. doi:10.1101/sqb.1987.052.01.005
Mills, B. J. W., Krause, A. J., Scotese, C. R., Hill, D. J., Shields, G. A., and Lenton, T. M. (2018). Modelling the long-term carbon cycle, atmospheric CO2, and Earth surface temperature from late neoproterozoic to present day. Gondwana Res. 67, 172–186. doi:10.1016/j.gr.2018.12.001
Milshteyn, D., Damer, B., Havig, J., and Deamer, D. (2018). Amphiphilic compounds assemble into membranous vesicles in hydrothermal hot spring water but not in seawater. Life 8, 11. doi:10.3390/life8020011
Miyakawa, S., Cleaves, H. J., and Miller, S. L. (2002). The cold origin of life: A. Implications based on the hydrolytic stabilities of hydrogen cyanide and formamide. Orig. Life Evol. Biosph. 32, 195–208. doi:10.1023/a:1016514305984
Morasch, M., Liu, J., Dirscherl, C. F., Ianeselli, A., Kühnlein, A., Vay, K. L., et al. (2019). Heated gas bubbles enrich, crystallize, dry, phosphorylate and encapsulate prebiotic molecules. Nat. Chem. 11, 779–788. doi:10.1038/s41557-019-0299-5
Morse, J. W., and Mackenzie, Fred.F. (1998). Hadean Ocean carbonate chemistry. Aquat. Geochem. 4, 301–319. doi:10.1023/a:1009632230875
Moschner, C., Wedd, C., and Bakshi, S. (2022). The context matrix: Navigating biological complexity for advanced biodesign. Front. Bioeng. Biotechnol. 10, 954707. doi:10.3389/fbioe.2022.954707
Mulkidjanian, A. Y., Bychkov, A. Yu., Dibrova, D. V., Galperin, M. Y., and Koonin, E. V. (2012). Origin of first cells at terrestrial, anoxic geothermal fields. Proc. Natl. Acad. Sci. U. S. A. 109, E821–E830. doi:10.1073/pnas.1117774109
Neal, C., Neal, M., Hughes, S., Wickham, H., Hill, L., and Harman, S. (2007). Bromine and bromide in rainfall, cloud, stream and groundwater in the plynlimon area of mid-wales. Hydrol. Earth Syst. Sci. 11, 301–312. doi:10.5194/hess-11-301-2007
Negrel, P., Petelet Giraud, E., Kloppmann, W., and Casanova, J. (2002). Boron isotope signatures in the coastal groundwaters of French guiana. Water Resour. Res. 38, 44-1–44-5. 44–45. doi:10.1029/2002wr001299
Novack, V. (2000).Process chemistry in the pharmaceutical industry edited by Kumar Gadamasetti. New York: Marcel Dekker, Inc. Xii + 474 Pp. 18 × 26 Cm 43, 526–526. doi:10.1021/jm990629g
O’Neill, C., Turner, S., and Rushmer, T. (2018). The inception of plate tectonics: A record of failure. Phil. Trans. R. Soc. A 376, 20170414. doi:10.1098/rsta.2017.0414
Partin, C. A., Bekker, A., Planavsky, N. J., Scott, C. T., Gill, B. C., Li, C., et al. (2013). Large-scale fluctuations in precambrian atmospheric and oceanic oxygen levels from the record of U in shales. Earth Planet. Sci. Lett. 369, 284–293. doi:10.1016/j.epsl.2013.03.031
Pasek, M. A. (2019). A role for phosphorus redox in emerging and modern biochemistry. Curr. Opin. Chem. Biol. 49, 53–58. doi:10.1016/j.cbpa.2018.09.018
Patel, B. H., Percivalle, C., Ritson, D. J., Duffy, C. D., and Sutherland, J. D. (2015). Common origins of RNA, protein and lipid precursors in a cyanosulfidic protometabolism. Nat. Chem. 7, 301–307. doi:10.1038/nchem.2202
Pearce, B. K. D., Pudritz, R. E., Semenov, D. A., and Henning, T. K. (2017). Origin of the rna world: the fate of nucleobases in warm little ponds.Proceedings of the National Academy of Sciences,114 11327-11332 doi:10.1073/pnas.1710339114
Planavsky, N. J., Asael, D., Hofmann, A., Reinhard, C. T., Lalonde, S. V., Knudsen, A., et al. (2014). Evidence for oxygenic photosynthesis half a billion years before the great oxidation event. Nat. Geosci. 7, 283–286. doi:10.1038/ngeo2122
Planavsky, N. J., McGoldrick, P., Scott, C. T., Li, C., Reinhard, C. T., Kelly, A. E., et al. (2011). Widespread iron-rich conditions in the mid-proterozoic ocean. Nature 477, 448–451. doi:10.1038/nature10327
Powner, M. W., and Sutherland, J. D. (2010). Phosphate-mediated interconversion of ribo and arabino configured prebiotic nucleotide intermediates. Angew. Chem. Int. Ed. 49, 4641–4643. doi:10.1002/anie.201001662
Ranjan, S., Kufner, C. L., Lozano, G. G., Todd, Z. R., Haseki, A., and Sasselov, D. D. UV transmission in natural waters on prebiotic Earth. Astrobiology,22 242-262, Arxiv 2021.
Ranjan, S., Todd, Z. R., Sutherland, J. D., and Sasselov, D. D. (2018). Sulfidic anion concentrations on early Earth for surficial origins-of-life chemistry. Astrobiology 18, 1023–1040. doi:10.1089/ast.2017.1770
Rasmussen, B., Muhling, J. R., Suvorova, A., and Fischer, W. W. (2021). Apatite nanoparticles in 3.46–2.46 Ga iron formations: Evidence for phosphorus-rich hydrothermal plumes on early Earth. Geology 49, 647–651. doi:10.1130/g48374.1
Rimmer, P. B., Thompson, S. J., Xu, J., Russell, D. A., Green, N. J., Ritson, D. J., et al. (2021). Timescales for prebiotic photochemistry under realistic surface ultraviolet conditions. Astrobiology 21, 1099–1120. doi:10.1089/ast.2020.2335
Rimmer, P. B., Xu, J., Thompson, S. J., Gillen, E., Sutherland, J. D., and Queloz, D. (2018). The origin of RNA precursors on exoplanets. Sci. Adv. 4, eaar3302. eaar3302. doi:10.1126/sciadv.aar3302
Ritson, D., and Sutherland, J. D. (2012). Prebiotic synthesis of simple sugars by photoredox systems chemistry. Nat. Chem. 4, 895–899. doi:10.1038/nchem.1467
Roberts, N. M. W., and Spencer, C. J. (2014). The zircon archive of continent formation through time. Geol. Soc. Lond Spec. Publ. 389, 197–225. doi:10.1144/sp389.14
Robertson, M. P., Levy, M., and Miller, S. L. (1996). Prebiotic synthesis of diaminopyrimidine and thiocytosine. J. Mol. Evol. 43, 543–550. doi:10.1007/bf02202102
Rollinson, H. (2007). When did plate tectonics begin? Geol. Today 23, 186–191. doi:10.1111/j.1365-2451.2007.00631.x
Sasselov, D. D., Grotzinger, J. P., and Sutherland, J. D. (2020). The origin of life as a planetary phenomenon. Sci. Adv. 6, eaax3419. doi:10.1126/sciadv.aax3419
Schwartz, A. W. (2013). Evaluating the plausibility of prebiotic multistage syntheses. Astrobiology 13, 784–789. doi:10.1089/ast.2013.1057
Scott, C., Planavsky, N. J., Dupont, C. L., Kendall, B., Gill, B. C., Robbins, L. J., et al. (2013). Bioavailability of zinc in marine systems through time. Nat. Geosci. 6, 125–128. doi:10.1038/ngeo1679
Sessions, A. L., Doughty, D. M., Welander, P. V., Summons, R. E., and Newman, D. K. (2009). The continuing puzzle of the great oxidation event. Curr. Biol. 19, R567–R574. doi:10.1016/j.cub.2009.05.054
Seyfried, W. E., Pester, N. J., Ding, K., and Rough, M. (2011). Vent fluid chemistry of the rainbow hydrothermal system (36°N, MAR): Phase equilibria and in situ PH controls on subseafloor alteration processes. Geochim. Cosmochim. Acta 75, 1574–1593. doi:10.1016/j.gca.2011.01.001
Seyfried, W. E., Pester, N. J., Tutolo, B. M., and Ding, K. (2015). The lost city hydrothermal system: Constraints imposed by vent fluid chemistry and reaction path models on subseafloor heat and mass transfer processes. Geochim. Cosmochim. Acta 163, 59–79. doi:10.1016/j.gca.2015.04.040
Shapiro, R. (1984). The improbability of prebiotic nucleic acid synthesis. Orig. Life 14, 565–570. doi:10.1007/bf00933705
Shaw, G. H. (2008). Earth’s atmosphere – hadean to early proterozoic. Geochemistry 68, 235–264. doi:10.1016/j.chemer.2008.05.001
Sleep, N. H. (2010). The hadean-archaean environment. Cold Spring Harb. Perspect. Biol. 2, a002527. doi:10.1101/cshperspect.a002527
Smit, M. A., and Mezger, K. (2017). Earth’s early O2 cycle suppressed by primitive continents. Nat. Geosci. 10, 788–792. doi:10.1038/ngeo3030
Terasawa, H., Nishimura, K., Suzuki, H., Matsuura, T., and Yomo, T. (2012). Coupling of the fusion and budding of giant phospholipid vesicles containing macromolecules. Proc. Natl. Acad. Sci. U. S. A. 109, 5942–5947. doi:10.1073/pnas.1120327109
Thomassot, E., O’Neil, J., Francis, D., Cartigny, P., and Wing, B. A. (2015). Atmospheric record in the hadean eon from multiple sulfur isotope measurements in nuvvuagittuq greenstone belt (nunavik, quebec). Proc. Natl. Acad. Sci. U. S. A. 112, 707–712. doi:10.1073/pnas.1419681112
Todd, Z. R., Szabla, R., Szostak, J. W., and Sasselov, D. D. (2019). UV photostability of three 2-aminoazoles with key roles in prebiotic chemistry on the early Earth. Chem. Commun. 55, 10388–10391. doi:10.1039/c9cc05265h
Toner, J. D., and Catling, D. C. (2020). A carbonate-rich lake solution to the phosphate problem of the origin of life. Proc. Natl. Acad. Sci. U. S. A. 117, 883–888. doi:10.1073/pnas.1916109117
Toner, J. D., and Catling, D. C. (2019). Alkaline Lake settings for concentrated prebiotic cyanide and the origin of life. Geochim. Cosmochim. Acta 260, 124–132. doi:10.1016/j.gca.2019.06.031
Uppström, L. R. (1974). The boron/chlorinity ratio of deep-sea water from the pacific ocean. Deep Sea Res. Oceanogr. Abstr. 21, 161–162. doi:10.1016/0011-7471(74)90074-6
Valley, J. W., Peck, W. H., King, E. M., and Wilde, S. A. (2002). A cool early Earth. Geol. 30, 351–354. doi:10.1130/0091-7613(2002)030<0351:acee>2.0.co;2
Visscher, P. T., Gallagher, K. L., Bouton, A., Farias, M. E., Kurth, D., Sancho-Tomás, M., et al. (2020). Modern arsenotrophic microbial mats provide an analogue for life in the anoxic archean. Commun. Earth Environ. 1, 24. doi:10.1038/s43247-020-00025-2
Westall, F., Hickman-Lewis, K., Hinman, N., Gautret, P., Campbell, K. A., Bréhéret, J. G., et al. (2018). A hydrothermal-sedimentary context for the origin of life. Astrobiology 18, 259–293. doi:10.1089/ast.2017.1680
Wołos, A., Roszak, R., Żądło-Dobrowolska, A., Beker, W., Mikulak-Klucznik, B., Spólnik, G., et al. (2020). Synthetic connectivity, emergence, and self-regeneration in the network of prebiotic chemistry. Science 369, eaaw1955. doi:10.1126/science.aaw1955
Wu, L.-F., and Sutherland, J. D. (2019). Provisioning the origin and early evolution of life. Emerg. Top. Life Sci. 3, 459–468. doi:10.1042/etls20190011
Yadav, M., Kumar, R., and Krishnamurthy, R. (2020). Chemistry of abiotic nucleotide synthesis. Chem. Rev. 120, 4766–4805. doi:10.1021/acs.chemrev.9b00546
Yanagawa, H., Ogawa, Y., Kojima, K., and Ito, M. (1988). Construction of protocellular structures under simulated primitive Earth conditions. Orig. Life Evol. Biosph. 18, 179–207. doi:10.1007/bf01804670
Zahnle, K., Arndt, N., Cockell, C., Halliday, A., Nisbet, E., Selsis, F., et al. (2007). Emergence of a habitable planet. Space Sci. Rev. 129, 35–78. doi:10.1007/s11214-007-9225-z
Zheng, X.-Y., Beard, B. L., Reddy, T. R., Roden, E. E., and Johnson, C. M. (2016). Abiologic silicon isotope fractionation between aqueous Si and Fe(III)–Si gel in simulated archean seawater: Implications for Si isotope records in precambrian sedimentary rocks. Geochim. Cosmochim. Acta 187, 102–122. doi:10.1016/j.gca.2016.05.012
Keywords: origins of life, prebiotic chemical evolution, early earth, geochemistry, environments
Citation: Walton CR, Rimmer P and Shorttle O (2022) Can prebiotic systems survive in the wild? An interference chemistry approach. Front. Earth Sci. 10:1011717. doi: 10.3389/feart.2022.1011717
Received: 04 August 2022; Accepted: 21 November 2022;
Published: 05 December 2022.
Edited by:
Jean-louis Vigneresse, Université de Lorraine, FranceReviewed by:
Terence Phillip Kee, University of Leeds, United KingdomCopyright © 2022 Walton, Rimmer and Shorttle. This is an open-access article distributed under the terms of the Creative Commons Attribution License (CC BY). The use, distribution or reproduction in other forums is permitted, provided the original author(s) and the copyright owner(s) are credited and that the original publication in this journal is cited, in accordance with accepted academic practice. No use, distribution or reproduction is permitted which does not comply with these terms.
*Correspondence: Craig R. Walton, Y3J3NTlAY2FtLmFjLnVr
Disclaimer: All claims expressed in this article are solely those of the authors and do not necessarily represent those of their affiliated organizations, or those of the publisher, the editors and the reviewers. Any product that may be evaluated in this article or claim that may be made by its manufacturer is not guaranteed or endorsed by the publisher.
Research integrity at Frontiers
Learn more about the work of our research integrity team to safeguard the quality of each article we publish.