- 1School of Mining, Liaoning Technical University, Fuxin, China
- 2State Key Laboratory of Coal Mining and Clean Utilization, Beijing, China
Coal burst has become a worldwide problem that needs to be solved urgently for the sake of coal mine safety production due to its complicated triggering mechanisms and numerous influencing factors. The risk assessment of coal burst disasters is particularly critical. In this work, 15 factors affecting coal burst occurrence are selected from the perspectives of geodynamic environment and geological and mining conditions, and the influence mechanism of each factor on coal bursts is analyzed. An evaluation index system of coal burst risk is put forward. A hierarchical model of coal burst prediction is established, and the weight of each influencing factor to coal burst risk is calculated. Based on the fuzzy comprehensive evaluation method, a coal burst prediction model is established, which can scientifically decompose and simplify the complicated problem and make coal burst prediction and prevention more pertinent and effective. The model is applied to assess the coal burst risk level of a coal mine in Shanxi Province, and the evaluation result is consistent with practical situations. This method considers the influencing factors comprehensively and determines the weight of each factor scientifically compared with other forecasting methods.
Introduction
Coal burst is a complex nonlinear dynamic phenomenon and a geological disaster induced by such artificial activities as coal mining (Cai et al., 2016; Zhang et al., 2017). Since the first record of coal burst occurred at the South Staffordshire coalfield in Britain in 1738, coal bursts have occurred in almost all coal mining countries in the world. With the increase of mining depth in China, the number of coal mines subjected to coal burst accidents is growing year by year, and the coal burst hazard gradually becomes more serious. Therefore, it is of great significance to understand the prediction and prevention of coal burst disasters.
Characterized by complex triggering laws and numerous influencing factors, coal burst has become an acute and complicated problem in the monitoring and control of dynamic disasters and needs to be handled throughout the world for coal mine safety (Wei et al., 2018). Risk assessment, monitoring and warning, control measures, and safety protection concerning coal bursts constitute a “quaternity” theory and technique system of coal burst prevention. As the first task in the system, coal burst prediction is particularly critical. Pan, Xu, et al. (2014) used a coal charge monitoring system to detect the charge radiation signals in the coal rock ahead of the working face and analyzed the characteristics and change rules of the charge radiation of dynamic disasters in deep coal mines. Jiang et al. (2017) studied the triggering mechanism of coal burst in the Yuncheng coal mine based on the three-zone structure model and microseismic monitoring data. They concluded that high self-weight stress and movement of thick and hard overburden were the main causes of the coal burst incident in the Yuncheng coal mine and pointed out that adopting large pressure-relief boreholes and reducing mining speed are effective methods to preventing the coal burst with similar conditions. After analyzing the correlation between concentrated static load and coal burst, Pan, Wang, et al. (2014) developed a pre-evaluation model for burst risk assessment based on concentrated static load detection. Considering the incompatibility and uncertainty of coal burst-evaluating indices, Zhizhen et al. (2011) established a prediction model for estimating coal burst risk based on set pair analysis. According to the dynamic changes of coal masses and surrounding rocks during coal mining, Wang et al. (2014) constructed a space breeding model of stopes by regarding the coal seam and its roof and floor as a whole system. Xu et al. (2014) built a mechanical model of the drill pipe in the process of drilling and obtained the relations among drill pipe torque, coal stress, coal properties, and drilling speed. By inspecting the variation of the drill pipe torque, they obtained the distribution and change rules of the stress field at the borehole, thus achieving the purpose of predicting a coal burst. Gu et al. (2013) explored the relation between surrounding rock stress and drilling amount and predicted the coal burst risk in isolated working face by drilling bits. Cai et al. (2015), Zhu et al. (2016), and Chen et al. (2021) investigated the relationship between the tomographic images of P wave velocity and coal burst hazard and believed that coal burst hazard could be detected by passive seismic velocity tomography during longwall face mining and entry excavation. Li et al. (2015), He et al. (2017), and Wang et al. (2020) proposed a static and dynamic stresses superposition-based risk evaluation method of coal burst to pre-evaluate coal burst risk. Ahmed et al. (2017) proposed the Burst Potential Index (BPI) to estimate pillar burst tendency based on the energy storage rate (ESR). Shen et al. (2017) stated that the energy density risk index (EDRI) could more accurately reflect the potential coal burst distribution and be used in multi-seam mining. Cai et al. (2019) proposed a new index named “bursting strain energy (BSE)” to quantitatively assess coal burst propensity. Mottahedi and Ataei (2019) used the fuzzy theory and fault tree analysis in the investigation of coal burst probability. Sabapathy et al. (2019) utilized Burst Energy Coefficient methodology to evaluate the bump proneness of a mine through numerical modeling. Vardar et al. (2018) considered the weightings of the risk factors and put forward a semiquantitative coal burst risk classification system. At present, China mainly adopts the composite index method to forecast coal bursts; the drawback of the composite index method is that all weights are the same. Although a great deal of research has been made on the prediction of coal burst disasters in the global scientific community, the aforementioned methods inevitably have certain limitations because of complicated triggering laws/many influencing factors and unclear occurrence processes and it is still impossible to perfectly predict coal burst events. Therefore, it is necessary to comprehensively investigate the causing factors of coal bursts, analyze the influence degree of each factor on coal burst occurrence by means of suitable and objective approaches, and evaluate the risk levels of coal bursts based on the weights of influencing factors.
According to geodynamic environment, geological, and mining factors influencing coal bursts, a total of 15 sub-factors are determined in this paper. Based on the analysis of the influencing factors of coal bursts, a prediction model of coal burst risk is established by combining analytic hierarchy process (AHP) and fuzzy comprehensive evaluation (FCE) and is verified in a coal mine. The prediction model overcomes the drawback of the composite index method that all weights are the same. The results show that the model meets the requirements of coal burst prediction.
Analysis of Influencing Factors of Coal Bursts
Geodynamic Environment Factors
Geodynamic environment relates to the characteristics of crustal structure and movement and refers to the dynamic effects of tectonic form, tectonic movement, tectonic stress, and their combination mode on coal and rock masses under natural geological conditions (Zhu et al., 2018). Coal bursts are the result of the coupling effect of mining disturbance and crustal movement induced by the endogenic geological process. The overall intensity of a coal burst or the total released energy in a mine or a mining area depends on the characteristics of the geodynamic environment (Han et al., 2014).
Tectonic Depression
Analysis of the topography of the mining areas where dynamic disasters have occurred, such as Huainan, Hebi, Fuxin, Beipiao, Jixi, and other mining districts in China and Tashtagol, Donetsk, and Vorkuta in Russia, shows that despite different elevations, the mining areas are all located in the lower part of the terrain and it is obviously higher at the periphery of these areas. This topography is manifested as a tectonic depression (Han et al., 2011; Han et al., 2014). The two sides of the tectonic depression are bound to move downward for reaching a stable state. In a tectonic depression (Figure 1), as for a point at the depth of H to the surface, the gravitational gliding force points to the center of the depression. The ultimate effect of gravitational gliding is to increase the compressive stress in the depression center and improve the compression ability. Hence, there is high tectonic stress in the tectonic depression and relatively high deformation energy is accumulated in coal and rock masses under this condition. Through the surface topography map of the coal field, the structural depression can be determined by analyzing the overall surface morphology of the coal field.
One of the remarkable characteristics of tectonic depressions is that the elevation of the center is lower and the surrounding or both sides are higher. The dip angle is used to assess the geodynamic environment of a tectonic depression by the following equation:
where
In-situ Stress Field
The energy accumulation of coal and rock masses is the outcome of the combined effect of stress and deformation (Bai et al., 2018). Therefore, stress condition is one of the important indices to judge the occurrence of a coal burst. Tectonic stress usually refers to the crustal stress produced by tectonic movement, and it is mainly horizontal. The ratio of the maximum horizontal principal stress to the vertical stress, i.e., the lateral pressure ratio (λ), suggests the level of tectonic stress. According to the magnitude of λ, tectonic stress can be classified into four levels, namely, λ < 1, 1 ≤ λ < 1.3, 1.3 ≤ λ < 2, and λ > 2. Based on the buried depth and tectonic stress levels, data analysis is carried out on 67 coal burst coal mines at which coal burst accidents have occurred and 63 coal mines have no coal burst occurrence in China (Table 1). With the increase in mining depth (less than 400 m), coal bursts have taken place in the mines with a lateral pressure ratio greater than or equal to 1.3 (λ ≥ 1.3). When the mining depth is increased to 400–600 m and the lateral pressure ratio is less than unit (λ < 1), coal bursts begin to appear in mines. On the whole, at the same mining depth, the greater the lateral pressure ratio is, the higher the proportion of coal bursts is. Thereby, without considering the buried depth, the probability of coal burst occurrence is positively correlated with the lateral pressure ratio. The relationship between the lateral pressure ratio and the risk of coal burst occurrence is shown in Table 2.
Vertical Movement of Fault Blocks
The characteristics of crustal movement in China are that the south is mainly uplifted while the north is dominated by subsiding and that vertical movement of the earth’s crust is strong in the west and weak in the east. In the present study, 160 coal burst mines in China are mapped in the velocity diagram of vertical crustal movement of the Chinese continent, and the surface vertical deformation velocity of each coal burst mine is statistically analyzed. From the statistical data, it is noted that most coal burst mines are distributed in the uplifted zones of the earth’s crust or the regions between the ascending and subsiding areas where relative movement is violent. Only a few mines are located in the descending areas. It also shows that among the 160 coal burst mines searchable in China, there are 101 coal burst mines in the areas with severe relative movement; that is, the movement velocity is between −1 and 1 (−1 <x < 1), accounting for 63.13% of the total cases. 43 coal burst mines are located in the uplifted areas (x ≥ 1), accounting for 26.87% of the statistics. 16 coal burst mines are situated in the descending areas where the crustal movement velocity is less than −3 (i.e., x < −3), accounting for 10% of the statistical data. Thereby, according to the relation between coal burst mines and vertical crustal movement, the coal burst risk level under different velocities of vertical crustal movement can be determined, as listed in Table 3. Using the refined leveling network of repeated measurements within the Chinese mainland, the pseudo-inverse dynamic adjustment was calculated, and the modern vertical crustal deformation rate map of the Chinese mainland was compiled.
Tectonic Faulting
In view of active faults in China, more than 85% of the coal burst mines are connected with active faults. The active faults and their characteristics in some coal burst mines are shown in Table 4. Normally, there are large active faults in the vicinity of coal burst mines, most of which stay in the zone of influence of these faults. This indicates that the mine within the influence zone of active faults has the probability of coal burst occurrence.
Based on the research of the influence width of faults by Russian scholars, an empirical formula is revised as
where b is the influence width of a fault. K is the activity coefficient (K = 1, 2, 3). K = 1, K = 2, and K = 3 represent strong, moderate, and weak faulting activity, respectively. h is the vertical drop of the fault, [m]. According to the “Code for investigation of geotechnical engineering,” faults that were active in the Middle and Late Pleistocene and are still vigorous in the Holocene and have an average active velocity larger than 1 mm/a (v > 1 mm/a) and a historical earthquake magnitude equal to or larger than 7 (M ≥ 7) are regarded as strongly active faults. Active since the Mid-Late Pleistocene and relatively strong in the Holocene, 0.1 mm/a ≤v ≤ 1 mm/a and 5 ≤ M < 7, faults of this kind are considered as moderately active faults. If v < 0.1 mm/a and M < 5, these faults are deemed as weakly active faults. The average active velocity can be measured by GPS. Judging the fault structure in the field mainly depends on whether the leakage part of the rock layer on the surface is continuous, repeated, or missing, whether there are scratches, and local bending.
Geological Factors
Mining Depth
With the increasing depth of coal mining, the geostatic stress in the coal seam rises, the elastic energy in coal and rock masses is also accumulating, and the possibility of coal burst becomes larger as well (Zhao et al., 2018). That is, mining depth is positively correlated with the probability of coal burst occurrence (Figure 2). When the mining depth is equal to or smaller than 350 m (H ≤ 350 m), coal burst proneness is low. If 350 m ≤ H ≤ 500 m, the coal burst risk gradually increases. Starting from 500 m, the risk of coal burst occurrence increases sharply as mining depth enlarges. When the mining depth reaches 600 and 900 m, the impact index increases to 0.12 (Wt = 0.12) and 0.65 (Wt = 0.65), respectively, which is 3 times and 16 times the impact index at the depth of 500 m (Wt = 0.04).
Coal Seam Thickness and Change Characteristics
Statistical analysis has shown that a thicker coal seam leads to more coal bursts and higher coal burst intensity. The change in coal seam thickness has a significant influence on the occurrence of coal burst events. At the positions where the coal seam suddenly becomes thinner or thicker, coal bursts easily occur, because the bearing stress in these places will change. The variation coefficient of coal seam thickness is an important index to measure the stability of a coal seam. It can directly reflect the thickness and change the characteristics of the coal seam. Thus, this index is employed to evaluate the influence degree of coal seam thickness on coal bursts. A larger variation coefficient of coal seam thickness implies that the coal seam is more unstable and the coal burst risk is much higher.
The variation coefficient of coal seam thickness is calculated by the following equation:
where n is the total number of coal points participating in the evaluation. xi is the measured coal seam thickness at each coal point, [m].
Fault
The influence of faults on coal bursts is associated with the enormous energy released by the sudden relative dislocation of faults caused by mining activities. Practice has proved that when a working face is approaching a fault, the number of coal burst incidents has an obvious increase as well as the violence of coal burst (Chen et al., 2018). As shown in Figure 3, with the advance of the working face or the cutting machine, the influence zone of front abutment pressure extends forward. When it reaches the affected area of the fault, the tectonic stress of the fault and the front abutment pressure of the working face are superimposed, leading to an increase in abutment pressure near the fault and producing a newly highly stressed area. The middle position between the fault and the working face is the area with maximal stress superposition. If the fault itself can accumulate energy, the peak stress area after superposition is also prone to accumulate enormous energy that can easily induce a coal burst (Wei et al., 2021).
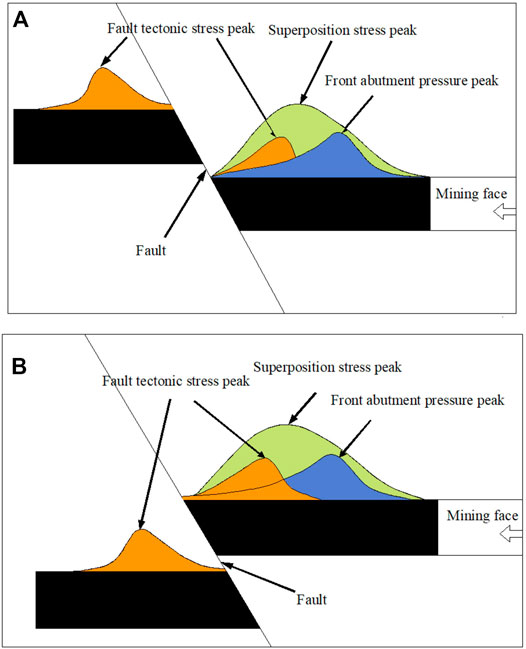
FIGURE 3. Superposition of front abutment pressure and fault tectonic stress during advancing a working face. (A) Normal fault, (B) reverse fault.
According to general experience, fault drop is a major influencing factor inducing a coal burst at the excavation face. Its corresponding stress concentration coefficient and influence zone are given in Table 5.
Coal Burst Tendency
Coal burst tendency is an inherent attribute of coal masses. It determines whether coal burst occurs and the specific risk degree under the same geological, mining, and other conditions. The impact tendency can be interpreted by the uniaxial compressive strength of coal. If the coal has a higher uniaxial compressive strength and a better integrity, more elastic energy is stored in the coal seam and coal bursts are more likely to happen under certain conditions (Bai and Shi 2017; Bai et al., 2020; Bai et al., 2019; Bai et al., 2021).
Structural Characteristics of Roof Strata
Studies have shown that the rock structure of the roof, especially hard and thick roof above the coal seam, is one of the main factors affecting the occurrence of coal bursts. The major reason is that hard and thick sandstone roof tends to accumulate a large amount of elastic energy. In the process of roof breaking or sliding, massive elastic energy is suddenly released, causing a strong vibration, leading to roof coal burst of impact pressure type or roof coal burst of impact type.
Elastic bending energy during the initial collapse of the roof is formulated as
Where q is the uniform load on the upper part of the hard roof, L is the initial breaking step of the hard roof, E is the elastic modulus of the hard roof, and I is the moment of inertia of the fracture section of the hard roof.
Elastic bending energy during the entire period of roof collapse is expressed as
From the above two equations, it can be seen that the elastic bending energy of the roof is proportionate to the fifth power of the roof span; that is, the larger the roof span (overhanging length) is, the more energy is accumulated. Generally, thicker hard rock is less likely to collapse and the resulted roof span is also larger. Hence, coal bursts can be easily triggered in thick and hard roof.
Factors of Mining Techniques
Collapse Degree of Overlying Strata
Coal burst occurrence is closely related to the collapse of overlying strata and the surface subsidence after excavation. If the thick and hard roof above the goaf has good continuity and integrity and fails to collapse in time, a large area of overhanging roof will be generated (Wu et al., 2021). When the thick and hard roof is disturbed by multiple adjacent working faces, it suddenly loses stability, which will produce a great amount of energy and trigger a coal burst. In view of this, the occurrence of a coal burst can be judged by whether the overburden collapses sufficiently.
The degree of overburden collapse can be evaluated by observing the breakage of the principle key stratum (Cheng et., 2018; Yang and Luo 2021) or geophysical detection methods. The degree of overburden collapse can also be judged based on the subsidence of the ground surface. Sufficient subsidence of the ground surface also indicates that the overlying strata have collapsed sufficiently.
Protective Seam Mining
After the protective seam is disturbed, cracks will inevitably develop in the surrounding rocks, which will make the surrounding rocks move toward the mining space. As a result, the rock strata above and below the goaf release stress, forming a “pressure relief zone” and causing damage in the nearby rock strata (Bai 2006; Bai and Li 2013; Bai et al., 2014). Rock breakage and movement are very violent at first, especially near the protective seam, and then decrease with the distance from the protective seam.
According to the national standard “Methods for test, monitoring and prevention of coal burst—Part 12: Prevention methods of protective seam mining,” when the evaluation area is in the effective zone and period of pressure relief, the pressure relief degree of the protective seam is prescribed as “good”; when the protective seam is in the effective zone of pressure relief but beyond the effective time, the pressure relief degree is considered as “medium”; if it is not in the effective zone of the protective seam, the degree of pressure relief is referred as “general”; if coal pillars are left unmined when the protective seam is mining and the plane projection of the coal pillar is in the evaluation area, the pressure relief degree of the protective seam is deemed as “poor.”
Vertical Distance Between Coal Seam and Upper Coal Pillar
With regard to mining multiple coal seams, priority should be given to the upper protective seam so that the lower coal seams are under-protected. However, in the actual mining process, some coal pillars are reserved in the overlying coal seam because of faults, changes in coal seam thickness, mining layout, and more. When mining the remaining coal seams, those reserved pillars often have a high degree of stress concentration due to the influence of mined-out space. The existence of overlying coal pillars increases the risk of coal burst occurrence at the working face. Meanwhile, coal burst risk increases with decreasing vertical distance from the coal pillar.
Width of Reserved Coal Pillar
Coal pillar reservation is a preferred method adopted in Chinese coal mines for protecting the developing roadways. It is an important factor affecting coal burst risk. In the past 22 years, about 60% of the coal bursts were induced by coal pillars, resulting in severe damage to roadways and numerous casualties. Reasonable pillar width plays a critical role in coal burst prevention. In consideration of coal burst prevention and control, the elastic zone in the coal pillar should be minimized on the premise of bearing the overburden load, so that the coal pillar is in the plastic zone to the greatest extent. This means that there is no elastic core inside the coal pillar and a large amount of elastic energy will not be stored. In this regard, small coal pillars are beneficial to the prevention and control of coal burst dangers. However, if the width of the coal pillar is too small, the pillar is easily fractured and collapsed under the overburden load and cannot exert the function of protecting the roadway. On the other hand, coal pillars with a width larger than 50 m will cause a huge loss of coal resources. As the width of coal pillars increases, the maximum vertical stress in the coal pillar increases firstly and then decreases again. In other words, when the width of the coal pillar is less than 10 m, the maximum vertical stress in the coal pillar is small. As the width of the coal pillar is 10–15 m, the maximum vertical stress in the coal pillar is relatively larger. With continuous increase in the pillar width, the maximum vertical stress in the coal pillar declines again.
Conditions of Adjacent Goafs
Due to the differences in mining methods, fault structures, and other factors, there are various forms of island working face during coal mining, such as one sidewall mined out, two sidewalls mined out, three sidewalls mined out, and four sidewalls mined out (Li et al., 2015; He et al., 2017; Wang et al., 2020). The working face is impacted by the movement of the roof but also by the instability and failure of the overlying rocks above the sidewalls and the roof of the adjacent goafs. As the working face is progressing, the surrounding rocks and the roof strata of the working face will move together and influence each other. Stress is highly concentrated in the surrounding rocks that are strongly disturbed by mining operations. In this way, the overburden movement is violent and coal burst disasters are easy to occur.
Thickness of Retaining Bottom Coal
When a coal burst takes place, it is usually accompanied by serious floor heave, and the reservation of thick bottom coal often encourages the occurrence of a coal burst. The roof and sidewalls are commonly supported whereas the floor is not supported, leading to the floor being the weakest area in the roadway. When the impact load acts upon the surrounding rocks, the energy will be released from the weakest area. This process is inevitably accompanied by slow floor heave or impacts damage to the floor.
Figure 4 shows the stress conditions of the roadway floor. The influence of rock properties on coal burst mainly depends on the strength and load of the floor. If the rock has high strength and large bearing capacity, it has obvious resistance to the impact load. However, if the retaining bottom coal is relatively thick, the coal body is inevitably being the main bearing body and more prone to fail under the impact load. Especially, the bottom coal with impact tendency has the characteristics of accumulating elastic energy and causing impact damage. Coal burst risk grades of all influencing factors are shown in Table 6.
Coal Burst Prediction Model Based on Analytic Hierarchy Process and Fuzzy Comprehensive Evaluation
Establish the Hierarchical Model
According to the influencing factors which affect the occurrence of coal bursts, the goal index of coal burst risk (U) is firstly divided into three categories, namely, geodynamic environment factors (U1), geological factors (U2), and mining factors (U3). Accordingly, the set of first-class indices is obtained, U=(U1, U2, U3). Geodynamic environment factors (U1) can be further classified into four second-class indices, including dip angle of the tectonic depression (u11), ratio of the tectonic stress to the vertical stress (u12), vertical movement velocity of fault blocks (u13), and influence zone of fault structure (u14). The corresponding second-class index set of U1 is achieved, U1=(u11, u12, u13, u14). As for the geological factors (U2), it can be further sorted into 5 second-class indices, i.e., mining depth (u21), variation coefficient of coal seam thickness (u22), fault drop (u23), uniaxial compressive strength of coal (u24), and distance between the coal seam and the thick-hard roof strata (u25). Then the second-class index set of U2 is expressed as U2=(u 21, u 22, u 23, u 24, u 25). Mining factors (U3) are further split into 6 second-class indices, that is, collapse degree of the overlying strata (u31), pressure relief degree of the protective seam (u32), distance between the mining seam and the upper coal pillars (u33), relation between the working face and the adjacent goafs (u34), coal pillar width (u35), and thickness of the retaining bottom coal (u36). Thereupon, the corresponding subclass index set of U3 is U3=(u31, u32, u33, u34, u35, u36). In this way, the coal burst risk index is separated into two grades and a three-layer index system of coal burst risk assessment is established (target layer, criterion layer, and index layer), as illustrated in Figure 5.
Build the Evaluation Set
It is determined that the evaluation set (V) consists of four appraising grades, namely, no coal burst risk (v1), weak coal burst risk (v2), moderate coal burst risk (v3), and strong coal burst risk (v4), so V=(v1, v2, v3, v4).
It should be noted that b is the influence zone of active faults; thick-hard rock strata refer to the rock strata with a uniaxial compressive strength greater than 60 MPa and a thickness larger than 10 m.
Establish the Weight Set
Weight analysis is another important content in AHP, and the weight set is used to describe the importance degree of one factor relative to another in the factor set with respect to a target object. Weight analysis is conducted by three main steps. The first is to construct the judgment matrix. The second step is to decide the single hierarchical order and performing a consistency test. The last is to calculate the weight set.
Construct the Judgment Matrix
The judgment matrix reflects the priority relationship between each two factors in the same index set. It is usually achieved based on the comparative analysis of the importance of different factors at the same level to coal burst risk by using the expert survey method. The importance degree of each factor in the factor set is evaluated by the 1–9 scale method shown in Table 7 for pairwise comparisons, and the judgment matrix (A) is established as
where
A number of experts in the field of coal burst were invited to make comprehensive judgments based on the coal burst occurrence pattern, and the pairwise comparison matrixes were determined. The pairwise comparison matrix U indicating the importance of the criterion layer to the target layer is obtained as
The pairwise comparison matrixes for explaining the importance of the index layer to the criterion layer are U1, U2, and U3 given below.
Decide the Single Hierarchical Order and Perform a Consistency Test
Calculate the weight of each factor to the target layer:
1) Normalize the column vectors of the judgment matrix by
2)
3) Then,
where
Calculate the largest eigenvalue of the matrix by the following equation:
The consistency index (CI) is formulated as
The larger value of CI indicates a higher inconsistency degree of the matrix. The random consistency index (RI) is determined by practical experience, as shown in Table 8. Then the consistency ratio of the matrix is obtained by
When the consistency ratio is less than 0.1, the consistency of the pairwise matrix is acceptable and the eigenvector of the matrix can be used as the weight vector. However, if the CR exceeds 0.1, the evaluation matrix fails the consistency test and needs to be reconstructed.
The above method can be used to obtain the weight vector of each judgment matrix and the single hierarchical order, as well as the consistency index and the consistency ratio, as given in Table 9. It is shown in Table 9 that the consistency ratios of 4 judgment matrixes are all less than 0.1, which meets the requirement of consistency.
Calculate the Weight Set
The relative importance of one layer to the upper layer is calculated from the top layer to the subclass layer. The weight vectors of individual factors with respect to coal burst risk can be used as the weight set of the FCE model.
After calculation, the weight set is W = [0.0430, 0.1598, 0.0232, 0.0859, 0.1891, 0.0328, 0.0805, 0.1114, 0.0766, 0.0160, 0.0270, 0.0270, 0.0455, 0.0719, 0.0103].
Establish the Membership Matrix
The membership matrix is used to reflect the degree of a single factor (index) that belongs to the subsets of the evaluation set, which is the basic evaluation result.
To evaluate the ith single factor ui in the factor set, the degree of the ith factor ui belonging to the jth subset vj in the evaluation set is defined as rij, and the membership set of the ith factor ui can be expressed as Ri =(ri1, ri2, ri3, ri4). The membership degrees of individual factors jointly constitute a 15 × 4 fuzzy relation matrix (R).
The membership degree can be determined by the membership function of each factor. The membership functions of the dip angle of the tectonic depression (u11), the ratio of the tectonic stress to the vertical stress (u14), the influence zone of the fault structure (u14), mining depth (u21), the variation coefficient of coal seam thickness (u22), fault drop (u23), the uniaxial compressive strength of coal (u24), the distance between the thick-hard rock strata and the coal seam (u25), the distance between the mining seam and upper coal pillars (u33), and the thickness of the retaining bottom coal (u36) present semi-trapezoidal and trapezoidal distributions based on the analysis of various factors, as shown in the following equations:
The membership functions of vertical movement velocity of fault blocks (u13), collapse degree of the overlying strata (u31), pressure relief degree of the protective seam (u32), relation between the working face and adjacent goafs (u34), and coal pillar width (u35) show a rectangular distribution based on the analysis of each factor.
In the previous equations,
Multi-Level Comprehensive Evaluation
After the weight set (W) and the fuzzy relation matrix (R) are determined, a synthetic operation is carried out and the FCE set (B) can be obtained,
where
Application Example
At the same time, the values of each factor are determined according to field test, geological data analysis, and related data of mine layout, as shown in Table 10.
The membership of each factor constitutes a 15 × 4 fuzzy matrix, which is calculated by the membership functions and developed as below:
After the weight set (W) and the fuzzy matrix (R) are determined, the synthesis operation is carried out and the FCE set (
Conclusion
Fifteen factors influencing coal bursts are selected from the perspectives of geodynamic environment, geological conditions, and mining conditions. The influence mechanism of each influencing factor on coal bursts is also analyzed. The influence of gas and groundwater can be considered as the deepening of future research. With the continuous in-depth study of rock burst mechanism, the prediction method can be further enriched.
The evaluation index system of coal bursts is put forward, a hierarchical model of coal burst prediction is established, and the weight of each influencing factor to coal burst occurrence is calculated. Based on the FCE method, a coal burst evaluation model is constructed, which can scientifically decompose and simplify the complicated problem and make the coal burst prediction and prevention more pertinent and effective. This method can be applied to the prediction of rock bursts of different scales. When predicting rock bursts in mining areas, mines, working faces, and roadways, the values of various indicators are selected in the corresponding area.
Compared with other forecasting methods, this method considers the influencing factors comprehensively and determines the weight of each factor scientifically. The model is then verified in a coal mine in Shanxi Province to predict the grade of coal burst risk in this mine, and the prediction result is consistent with practical situations.
This method can provide a reference for other dynamic disasters such as rock bursts and coal and gas outbursts. The common factors of mine dynamic disasters can be used in the prediction, the unique factors of rock bursts can be removed, and the influencing factors of other disasters can be integrated.
Data Availability Statement
The original contributions presented in the study are included in the article/supplementary material; further inquiries can be directed to the corresponding author.
Author Contributions
ZZ contributed to the conception of the study. YW contributed significantly to the analysis and manuscript preparation. JH helped perform the analysis with model discussions.
Funding
This work was supported by State Key Laboratory of Coal Mining and Clean Utilization (2021-CMCU-KF016) and Basic Scientific Research Projects of Universities in Liaoning Province (LJKZ0343).
Conflict of Interest
The authors declare that the research was conducted in the absence of any commercial or financial relationships that could be construed as a potential conflict of interest.
Publisher’s Note
All claims expressed in this article are solely those of the authors and do not necessarily represent those of their affiliated organizations, or those of the publisher, the editors, and the reviewers. Any product that may be evaluated in this article, or claim that may be made by its manufacturer, is not guaranteed or endorsed by the publisher.
Acknowledgments
The assistance and guidance of the steering group members is gratefully acknowledged.
References
Ahmed, S. S., Alheib, M., Gunzburger, Y., and Renaud, V. (2017). “Pillar Burst Assessment Based on Large-Scale Numerical Modeling,” in ISRM European Rock Mechanics Symposium (EUROCK), Ostrava, CZECH REPUBLIC, 179–187. doi:10.1016/j.proeng.2017.05.170
Bai, B. (2006). Fluctuation Responses of Saturated Porous media Subjected to Cyclic thermal Loading. Comput. Geotechnics 33, 396–403. doi:10.1016/j.compgeo.2006.08.005
Bai, B., Guo, L., and Han, S. (2014). Pore Pressure and Consolidation of Saturated Silty clay Induced by Progressively Heating/cooling. Mech. Mater. 75, 84–94. doi:10.1016/j.mechmat.2014.04.005
Bai, B., Xu, T., Nie, Q., and Li, P. (2020). Temperature-driven Migration of Heavy Metal Pb2+ along with Moisture Movement in Unsaturated Soils. Int. J. Heat Mass Transfer 153, 119573. doi:10.1016/j.ijheatmasstransfer.2020.119573
Bai, B., Yang, G.-C., Li, T., and Yang, G.-S. (2019). A Thermodynamic Constitutive Model with Temperature Effect Based on Particle Rearrangement for Geomaterials. Mech. Mater. 139, 103180. doi:10.1016/j.mechmat.2019.103180
Bai, B., Zhou, R., Cai, G., Hu, W., and Yang, G. (2021). Coupled Thermo-Hydro-Mechanical Mechanism in View of the Soil Particle Rearrangement of Granular Thermodynamics. Comput. Geotechnics 137, 104272. doi:10.1016/j.compgeo.2021.104272
Bai, B., and Li, T. (2013). Irreversible Consolidation Problem of a Saturated Porothermoelastic Spherical Body with a Spherical Cavity. Appl. Math. Model. 37, 1973–1982. doi:10.1016/j.apm.2012.05.003
Bai, B., Rao, D., Xu, T., and Chen, P. (2018). SPH-FDM Boundary for the Analysis of thermal Process in Homogeneous media with a Discontinuous Interface. Int. J. Heat Mass Transfer 117, 517–526. doi:10.1016/j.ijheatmasstransfer.2017.10.004
Bai, B., and Shi, X. (2017). Experimental Study on the Consolidation of Saturated Silty clay Subjected to Cyclic thermal Loading. Geomech. Eng. 12, 707–721. doi:10.12989/gae.2017.12.4.707
Cai, W., Dou, L., Gong, S., Li, Z., and Yuan, S. (2015). Quantitative Analysis of Seismic Velocity Tomography in Rock Burst hazard Assessment. Nat. Hazards 75, 2453–2465. doi:10.1007/s11069-014-1443-6
Cai, W., Dou, L., Si, G., Cao, A., He, J., and Liu, S. (2016). A Principal Component Analysis/fuzzy Comprehensive Evaluation Model for Coal Burst Liability Assessment. Int. J. Rock Mech. Mining Sci. 81, 62–69. doi:10.1016/j.ijrmms.2015.09.028
Cai, W., Dou, L., Si, G., Cao, A., Gong, S., Wang, G., et al. (2019). A New Seismic-Based Strain Energy Methodology for Coal Burst Forecasting in Underground Coal Mines. Int. J. Rock Mech. Mining Sci. 123, 104086. doi:10.1016/j.ijrmms.2019.104086
Chen, F., Cao, A., Liang, Z., and Liu, Y. (2021). A Coal Burst Risk Assessment Model of Seismic Events Based on Multiple Seismic Source Parameters: A Case Study of the Huating Coal Mine, Gansu Province, China. Nat. Resour. Res. 30 (6), 4515–4532. doi:10.1007/s11053-021-09938-x
Chen, L., Shen, B., and Dlamini, B. (2018). Effect of Faulting on Coal Burst - A Numerical Modelling Study. Int. J. Mining Sci. Technol. 28, 739–743. doi:10.1016/j.ijmst.2018.07.010
Cheng, J., Zhao, G., and Li, S. (2018). Predicting Underground Strata Movements Model with Considering Key Strata Effects. Geotech Geol. Eng. 36, 621–640. doi:10.1007/s10706-017-0307-8
Gu, S., Huang, R., Jiang, B., and Zhang, R. (2013). “Study on the Critical Exponent of the Rock Burst Risk with Method of Drilling Bits in Island Working Face,” in 3rd International Workshop on Mine Hazards Prevention and Control (Brisbane, AUSTRALIA: CSIRO Earth Sci & Resource Engn), 79–83.
Han, J., Zhang, H., Lan, T., and Li, S. (2014). Geodynamic Environment of Rockburst in Western Beijing coalfield. J. China Coal Soc. 39, 1056–1062. doi:10.13225/j.cnki.jccs.2013.1426
Han, J., Zhang, H. W., Song, W. H., and Li, S., and (2011). Coal and Gas Outburst Mechanism and Risk Analysis of Tectonic Concave. J. China Coal Soc. 36, 108–113. doi:10.13225/j.cnki.jccs.2011.s1.029
He, J., Dou, L., Gong, S., Li, J., and Ma, Z. (2017). Rock Burst Assessment and Prediction by Dynamic and Static Stress Analysis Based on Micro-seismic Monitoring. Int. J. Rock Mech. Mining Sci. 93, 46–53. doi:10.1016/j.ijrmms.2017.01.005
Jiang, F., Liu, Yi., Yang, W., Wen, J., Zhang, Y., Liu, X., et al. (2017). Relationship between Rock Burst and the Three Zone Structure Loading Model in Yuncheng Coal Mine. J. Mining Saf. Eng. 34, 405–410. 10.13545/j.cnki.jmse.2017.03.001.
Li, Z.-l., Dou, L.-m., Wang, G.-f., Cai, W., He, J., and Ding, Y.-l. (2015). Risk Evaluation of Rock Burst through Theory of Static and Dynamic Stresses Superposition. J. Cent. South. Univ. 22, 676–683. doi:10.1007/s11771-015-2570-2
Mottahedi, A., and Ataei, M. (2019). Fuzzy Fault Tree Analysis for Coal Burst Occurrence Probability in Underground Coal Mining. Tunnelling Underground Space Technol. 83, 165–174. doi:10.1016/j.tust.2018.09.029
Pan, J., Wang, S., Liu, S., and Feng, M. (2014). Pre-evaluation of Burst Hazards Based on Concentrated Static Load Detection. Chin. J. Geotechnical Eng. 36, 1227–1234. doi:10.11779/CJGE201407006
Pan, Y., Xu, L., Li, G., Zeng, X., Li, Z., and Song, Y. (2014). Characteristics of Charge Radiation of Dynamic Disasters in Deep Coal Mine, Chin. J. Rock Mech. Eng. 33 (8), 1619–1625. doi:10.13722/j.cnki.jrme.2014.08.012
Sabapathy, R., Paul, P. S., and Mandal, P. K. (2019). Evaluation of Bump-Proneness of Underground Coal Mines Using Burst Energy Coefficient. Arabian J. Geosciences 12. doi:10.1007/s12517-019-4746-9
Shen, W., Dou, L-m., Hu, H., and Zhu, G-a. (2017). Rock Burst Assessment in Multi-Seam Mining: a Case Study. Arabian J. Geosciences 10. doi:10.1007/s12517-017-2979-z
Vardar, O., Zhang, C., Canbulat, I., and Hebblewhite, B. (2018). A Semi-quantitative Coal Burst Risk Classification System. Int. J. Mining Sci. Technol. 28, 721–727. doi:10.1016/j.ijmst.2018.08.001
Wang, C., Cao, A., Zhang, C., and Canbulat, I. (2020). A New Method to Assess Coal Burst Risks Using Dynamic and Static Loading Analysis. Rock Mech. Rock Eng. 53, 1113–1128. doi:10.1007/s00603-019-01968-5
Wang, G., Dou, L., Li, Z., Gong, S., and Cai, W. (2014). Space breeding mechanism of rock burst and its microseismic characteristics. J. Caikuang Yu Anquan Gongcheng Xuebao/journal Mining Saf. Engineering 31, 41–48. doi:10.13545/j.issn1673-3363.2014.01.007
Wei, C., Zhang, C., Canbulat, I., and Huang, W. (2021). Numerical Investigation into Impacts of Major Fault on Coal Burst in Longwall Mining-A Case Study. Int. J. Rock Mech. Mining Sci. 147, 104907. doi:10.1016/j.ijrmms.2021.104907
Wei, C., Zhang, C., Canbulat, I., Cao, A., and Dou, L. (2018). Evaluation of Current Coal Burst Control Techniques and Development of a Coal Burst Management Framework. Tunnelling Underground Space Technol. 81, 129–143. doi:10.1016/j.tust.2018.07.008
Wu, W-d., Bai, J-b., Feng, G-r., and Wang, X-y. (2021). Investigation on the Mechanism and Control Methods for Roof Collapse Caused by cable Bolt Shear Rupture. Eng. Fail. Anal. 130, 105724. doi:10.1016/j.engfailanal.2021.105724
Xu, L., Qi, L., Pan, Y., Li, Z., Li, G., and Xiao, M. (2014). Study on Forecasting Rockburst of the Drill Pipe Torque Method. Eng. Mech. 31, 251–256. doi:10.6052/j.issn.1000-4750.2013.05.0456
Yang, J., and Luo, Y. (2021). Enhanced Subsurface Subsidence Prediction Model Incorporating Key Strata Theory. Mining, Metall. Exploration 38, 995–1008. doi:10.1007/s42461-021-00383-1
Zhang, C., Canbulat, I., Hebblewhite, B., and Ward, C. R. (2017). Assessing Coal Burst Phenomena in Mining and Insights into Directions for Future Research. Int. J. Coal Geology. 179, 28–44. doi:10.1016/j.coal.2017.05.011
Zhang, Z., Feng, G., Xu, A., and Liu, G. (2011). 'Model for Estimating Rock Burst Risk in a Coal Mine Based on Set Pair Analysis. J. China Univ. Mining Technol. 40, 379–384.
Zhao, T.-b., Guo, W.-y., Tan, Y.-l., Yin, Y.-c., Cai, L.-s., and Pan, J.-f. (2018). Case Studies of Rock Bursts under Complicated Geological Conditions during Multi-Seam Mining at a Depth of 800 M. Rock Mech. Rock Eng. 51, 1539–1564. doi:10.1007/s00603-018-1411-7
Zhu, G.-a., Dou, L.-m., Cai, W., Li, Z.-l., Zhang, M., Kong, Y., et al. (2016). Case Study of Passive Seismic Velocity Tomography in Rock Burst Hazard Assessment during Underground Coal Entry Excavation. Rock Mech. Rock Eng. 49, 4945–4955. doi:10.1007/s00603-016-1026-9
Keywords: coal burst prediction, analytic hierarchy process, fuzzy comprehensive evaluation, geodynamic environment, coal burst
Citation: Zhu Z, Wu Y and Han J (2022) A Prediction Method of Coal Burst Based on Analytic Hierarchy Process and Fuzzy Comprehensive Evaluation. Front. Earth Sci. 9:834958. doi: 10.3389/feart.2021.834958
Received: 14 December 2021; Accepted: 24 December 2021;
Published: 10 February 2022.
Edited by:
Xiaodong Fu, Institute of Rock and Soil Mechanics (CAS), ChinaReviewed by:
Zizheng Zhang, Hunan University of Science and Technology, ChinaZhenlei Li, University of Science and Technology Beijing, China
Wenda Wu, Taiyuan University of Technology, China
Copyright © 2022 Zhu, Wu and Han. This is an open-access article distributed under the terms of the Creative Commons Attribution License (CC BY). The use, distribution or reproduction in other forums is permitted, provided the original author(s) and the copyright owner(s) are credited and that the original publication in this journal is cited, in accordance with accepted academic practice. No use, distribution or reproduction is permitted which does not comply with these terms.
*Correspondence: Zhijie Zhu, emh1emhpamllQGxudHUuZWR1LmNu