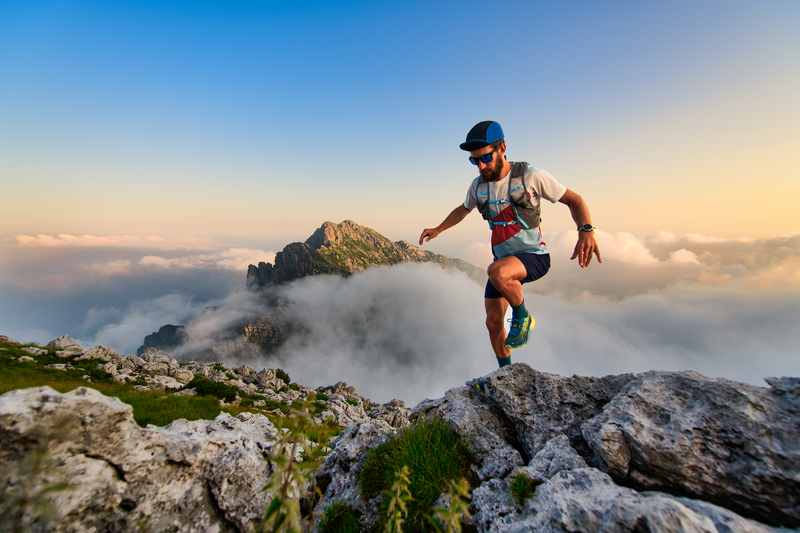
95% of researchers rate our articles as excellent or good
Learn more about the work of our research integrity team to safeguard the quality of each article we publish.
Find out more
ORIGINAL RESEARCH article
Front. Earth Sci. , 01 December 2021
Sec. Structural Geology and Tectonics
Volume 9 - 2021 | https://doi.org/10.3389/feart.2021.793016
This article is part of the Research Topic Advances in the Study of Natural Fractures in Deep and Unconventional Reservoirs View all 29 articles
China’s Paleozoic deep carbonate effective reservoirs, mainly non-porous reservoirs, are generally formed under the interaction of late diagenesis, hydrothermal fluids, and structural fractures. Faults and their deformation mechanism and internal structure of fault zones play an important role in the formation of carbonate reservoirs and hydrocarbon accumulation. Based on the detailed analysis of outcrop data in Xike’er area, Tarim Basin, this paper systematically studies the deformation mechanism and internal structure of reverse fault in the carbonate rock, and discusses the reservoir characteristics, control factors and development rules. The study shows that the deformation mechanism of the fault in carbonate rocks is faulting and fracturing, and the dual structure of fault core and damage zone is developed. The fault core is mainly composed of fault breccia, fault gouge and calcite zone, and a large number of fractures are formed in the damage zone, which are cemented by calcite locally. The mineral composition and rare earth element tests show that the fault core has the dual effect of hydrothermal fluids and atmospheric fresh water, which is easy to be cemented by calcite; while the damage zone is dominated by atmospheric fresh water, which is a favorable zone for the development of fracture-vuggy reservoirs. Therefore, the damage zone is the “sweet spot” area of carbonate oil and gas enrichment, and generally shows strip distribution along the fault.
Carbonate reservoir is an important part of global oil and gas. Its conventional oil and gas reserves account for about 60% of the world’s total reserves, and its production accounts for about 50%. The distribution area of carbonate rocks in China is nearly 3 million square kilometers, and the oil and gas exploration potential is huge. After the discovery of Renqiu oilfield in Hebei Province in the 1970s, China’s marine carbonate oil and gas exploration has successively discovered a number of large oil and gas fields, such as Shanzhong gas field in Ordos basin, Tahe-Lunnan, Tazhong and Shunbei oil and gas fields in Tarim Basin, and Northeast Sichuan gas field in Sichuan basin, etc. In recent years, China’s deep marine carbonate rocks are at the peak of discovery of large oil and gas fields. With the discovery of “fault controlled-karst” reservoirs in Shunbei area of Tarim Basin, exploration practice has proved that fault is not only an important pathway for oil and gas migration, but also an important reservoir space (Jiao., 2018; Cahngyu et al., 2019; Li et al., 2019; Baozeng, 2020; Zhiwen et al., 2020). Fault is a “three-dimensional geological body” with a certain width and containing fault rocks with different characteristics (Xiaofei et al., 2013; Lingdong et al., 2014). Faults zones localize shear deformation and are made up of intensely deformed fault cores encompassed within the fault damage zones (Sibson, 1977; Caine Saul et al., 1996; Shipton and Cowie, 2003; Crider and Peacock, 2004; Agosta and Aydin, 2006; De Joussineau and Aydin, 2007; Wibberley et al., 2008; Faulkner et al., 2010). The fault core has been transformed from the host into new rock types, such as mudstone, cataclastic rock, breccia and mylinite, due to high strain processes such as crushing, cataclastic, brecciation and crystal plasticity (Higgins 1971; Engelder 1974; Sibson 1977; Caine Saul et al., 1996; Evans et al., 1997; Chester et al., 2004; Berg and Skar 2005; Agosta and Aydin 2006; Mort and Woodcock 2008; Mitchell and Faulkner 2009; Faulkner et al., 2010; Michie 2015). Lower strain mechanisms create damage zones surrounding the fault core, such as fracturing and veining in low porosity or overconsolidated rock (e.g. Caine Saul et al., 1996; Chester et al., 2004; Agosta et al., 2007; De Joussineau and Aydin 2007; Gaviglio et al., 2009; Mitchell and Faulkner 2009; Bastesen and Braathen, 2010; Faulkner et al., 2010; Hausegger et al., 2010), or cataclasis and cementation creating deformation bands in high porosity rock (e.g. Shipton and Cowie 2003; Fossen et al., 2007; Schueller et al., 2013). Other processes, such as mineral reaction or precipitation/dissolution, can affect both zones (Kim et al., 2004; Agosta et al., 2007). Beyond the damage zone is the protolith, where no alteration from faulting is observed. The complex structural characteristics of the fault zone are formed due to the combined action of fault deformation and fluid modification. At present, oil and gas drilling in Tarim carbonate strike slip fault zone has achieved good results, but the exploration deployment of reverse fault has not been carried out yet. The fundamental reason lies in the unclear understanding of the internal structure and genetic mechanism of reverse fault. How to scientifically understand the mechanism of fault modification on carbonate reservoir is an important basis for exploration of large-scale reservoirs. Taking the field reverse fault in Xike’er area of Tarim Basin as the anatomical object, the internal structural characteristics and formation mechanism of reverse fault are discussed through the Analysis of internal structure characteristics and geochemical tests of macro to micro fault zone, and the reservoir control mode of reverse fault is clarified, which has important practical significance for improving the success rate of carbonate oil and gas exploration.
Tarim Basin is a multi-cycle, superimposed petroliferous basin composed of Paleozoic craton basin and Mesozoic-Cenozoic foreland basin (Chengzao, 1997). After multi-stage tectonic movement, Tarim Basin was associated with a series of fold and thrust belts. Aksu-Keping area is located in the northwest margin of Tarim Basin, which is the transition zone between the South Tianshan orogenic belt and the Tarim Basin and belongs to Tabei uplift belt. The Keping uplift is divided into Xike’er area, Keping area and Aksu area by Piqiang fault and Yingan mountain. The compression strike slip and thrust nappe are very strong in the area, so the reverse faults are relatively developed. Controlled by the Cambrian Ordovician global inundation event, the early Ordovician to Middle Ordovician in Tarim Basin showed an overall sea-level rise, from semi restricted platform to open platform. The early Ordovician is a large epicontinental sea platform, which basically inherited the Cambrian sedimentary pattern. After the Middle Ordovician, the plate movement in the southern margin of Tarim Basin changed from discrete to convergent. The basin entered the tectonic compression system, the basin pattern evolved to the North-South zoning, and the sedimentary environment also changed from marine facies to marine continental transitional facies (Yang et al., 2016).
The study area is located in the Akesu-Keping area of the Tarim Basin, and comprises the Bachu Uplift in the west (Figure 1A). It is composed of a series of marine carbonate rocks formed in open platform margin and evaporative lagoonal to restricted platform margin during the Cambrian- Lower Ordovician Periods. The Cambrian can be divided into three sub-sequences as follows: the Lower Cambrian includes the Yuertusi, Xiaoerbulake and Wusongger Formations, which contains thick dolomites, limestones, dolomicrites, microbialites/stromatolites bed, characterized by restricted to evaporative-lagoonal carbonate platform and open platform environment (Lin et al., 2009). The Middle Cambrian includes the Shayilike and Awatage Formations, which contains thick gypsum, anhydrite and salt layers. The upper Cambrian consists of the Xiaqiulitage Formation, which contains algae dolomite and argillaceous dolomite, limestone, atromatolitic dolomite etc (Ngia et al., 2019) (Figure 1C). The Lower-Middle Ordovician succession is divided into the Penglaiba Formation and the Yingshan Formation, and is composed mainly of thick bedded dolomites, dolomitic limestones, packstones-grainstones, formed on open platform to platform margins as reef and shoal deposits (Figure 1C) (Lin et al., 2012).
FIGURE 1. Location and geological map and lithological of the Akesu-Keping area in Tarim Basin. (A) Regional location map of the Tarim Basin. (B) 3D topographic map of the Akesu-Keping area, the Xike’er fault is located in the west of the Piqiang fault, which is a NW reverse fault with a steep dip of 83°, and the surrounding strata are mainly Cambrian, Ordovician and Silurian. (C) Lithologic histogram of Tarim Basin. It can be found in the bar chart that the outcropping strata in the study area are mainly carbonate strata.
The Ordovician carbonate rocks are widely exposed in the Xike’er area, Tarim Basin. The exposed strata are mainly Penglaiba formation (O1p), Yingshan formation (O1-2y) and Yijianfang Formation (O2y) (Figure 1). Different types and characteristics of fault zones are developed in this area (Wenyuan et al., 2002), but reverse faults are the main ones, such as Xike’er fault, the orientation of the reverse fault is NWW or EW. The orientation of the strike-slip faults, such as Piqiang fault, are NNW or NS (Figure 1). This paper mainly studies the structural characteristics and genetic mechanism of reverse fault in the carbonate rock.
Samples were collected from the Xike’er area to capture representative portions of fault rock and undeformed host rock. Block samples with weight greater than 200 g were taken for sample analysis, and polished thin sections were produced for optical microscopy. These samples have been perpendicular to fault strike. Samples were taken adjacent to the representative thin sections, to accurately capture the microstructures fully representing the measured petrophysical properties. Representative oriented host samples have also been collected, with core plugs and thin sections taken. For the Xike’er reverse fault, 16 samples were mainly selected, of which 4 were taken from the host rock, five were taken from the damage zone, six were taken from the fault core, and one was taken from the overlap zone.
All samples were cleaned using carbonate-saturated deionized water to remove salts that were likely to impact the petrophysical property measurements by occluding pore space. Cleaned core plugs were then placed in an oven and heated to 60 °C until dry.
All 16 samples are made of thin slices and observed under a Leica microscope made in Germany. The model of the microscope is DM4P. The maximum available field of view is 25 mm and supports 50-500x zoom. As the porosity of the fault zone samples is difficult to obtain, the porosity can only be reflected by observing the plane porosity of the thin section. The face plane porosity to the visible porosity of the rock under the microscope (without micro-voids), that is, the percentage of the void area to the total area of the observation field. A low-viscosity resin containing blue dye was used to make pore spaces more apparent when viewed in plane polarized light. Optical images from the thin sections were analysed to assess the deformation mechanisms in each sample based on documented deformation and/or diagenetic microstructures. These observations were used to divide samples into different fault rock categories and to interpret how different deformation mechanisms might govern the petrophysical properties of fault rocks.
The whole-rock mineralogy of all samples was determined by X-ray powder diffraction analysis (XRD) performed on randomly oriented powders following the sideloading method (Środoń et al., 2001). The XRD analysis was performed on the Brook X-ray diffractometer of Northeast Petroleum University. The model of the instrument is D8AA25, and the rated voltage and rated current are 60 kV and 80 mA, respectively.Data collection was carried out in the 2θ range -10–168° with a step size of 0.0001°. Operating temperature of 10–40°C and relative humidity less than or equal to 75% are the working conditions of this instrument.
The rare earth element analysis of samples at different positions of the fault zone was carried out to obtain the source of the fluid that affected the Hickel fault zone. Rare earth element analysis was carried out by ICP-MS X-Series-2 inductively coupled plasma mass spectrometer. The mass spectrum of the instrument was in the range of 2–255 amu, and the sensitivity was Y(89)>200Mcps/ppm. The testing accuracy of trace elements with the content of ppm-ppb level is high, the error is less than 5%
Based on the differences and influencing factors of deformation in the different rocks, the carbonate can be divided into high porosity (porosity greater than 15%) and low to non-porosity (tight) carbonate rock (porosity less than 15%) (Kaminskaite et al., 2019). The high porosity carbonate rocks are mainly cataclastic, and the associated sub-seismic structures are mainly deformation zones, which are the most common structures in high porosity carbonate rocks (Fossen et al., 2007; Agosta and Tondi, 2010; Bastesen et al., 2013; Rotevatn et al., 2016; 2017). However, low to non-porous carbonate rocks are dominated by shear fracturing, and associated sub-seismic structures are mainly fractures (Billi et al., 2003; Agosta, 2008; Woodcock et al., 2008; Bastesen et al., 2009; Michie and Haines, 2016; Panza et al., 2016). No matter what type of carbonate rock, cementation is also a common phenomenon in the fault zone of carbonate rock (Cooke et al., 2018).
From the geological map and stratigraphic relationship on both sides of the outcrop fault, it can be seen that the Xike’er fault is a NW trending reverse fault with a certain strike-slip property, and the fault dip angle is about 83° (Figure 2). The fault core is the most concentrated part of the rock mass displacement on both sides of the fault, it absorbs most of the displacement of the fault and develops sliding surfaces and fault rocks (Caine Saul et al., 1996; Cooke et al., 2019). The xike’er fault has a typical dual structure, and its fault core is characterized by multi-layer structure, which is filled with calcite vein, fault breccia and fault gouge along the sliding surface (Figure 3A). Multiple slip surfaces and fault breccias are developed in the fault core, and the fault plane is relatively smooth; several Calcite veins are developed along the fault plane, with the maximum width of 5 cm (Figures 3B,E). The fractures are relatively developed in the damage zone, which have a high permeability with the width of about 8.5 m (Figure 3C), the fault gouge is characterized by zonal distribution, with banded calcite vein filling and a small amount of small-scale breccia, with a width of about 1.3 m (Figures 3D,F).
FIGURE 2. The Xike’er fault pattern and macroscopic characteristics. The location of the section is shown in the geological map on the plane plan. In the profile the red line is the fault plane and the arrow points to the hanging wall of the reverse fault.
FIGURE 3. The development characteristics of the Xike’er reverse fault core. (A) A total of 16 samples were selected from the Xike’er fault zone, including fault core, damage zone, host rock and overlap zone. The blue dot indicates the position of samples and the dotted line indicates the sliding surface. The morphology of the fault core is different from that of the hostrock. (B) The fault core of the Xike’er fault. (C) The red line indicates a slip plane fault. (D) Fault breccia is mainly developed in the fault core. (E) The fault core is developed by the fault breccia, calcite veins and fault gouge.
The damage zone is located on both sides of the fault core, mainly secondary structures associated with the formation of the fault (Chester and Logan, 1986), including minor faults (Choi et al., 2015) and fractures (Vermilye and Scholz, 1999; Fu et al., 2012; Laubach et al., 2014; Lingdong et al., 2014; Xiaofei et al., 2014). There are two main methods for measuring the damage zone in the outcrop area: one is to measure the fractures density and compile the fractures density-distance change. The number of associated sub-seismic structures in the damage zone gradually decreases as the distance from the fault core increases. When the density is consistent with the regional fractures or deformation zones, it marks the end of the damage zone. The distribution of the of infection zone (fluid effect damage zone), and a graph of the distance-distance between the infected zone is compiled. As the distance from the fault zone increases, the disappearance of the infected zone marks the end of the damage zone (Walsh et al., 1998; Shipton and Cowie, 2003; Flodin and Aydin, 2004; Berg and Skar, 2005; Lubiniecki et al., 2019).
The damage zone of the Xike’er reverse fault mainly develops conjugate shear fractures, tensile fractures, mineral veins and caves, mostly with high-angle fractures. The fractures exposed in the study area show different orientation, include NE, NEE, SN. Four groups of fractures are developed in the Xike’er reverse fault (Figure 4). The macroscopic observation of the damage zone shows that the farther away from the fault core, the lower the degree of fracture development (Figure 5). Fractures are developed in the strongly deformed damage zone near the fault core, and the density of the fractures can reach nearly 30/m. The orientation of the fractures is complex and changeable, and the fractures are interwoven into a network. Far from the weakly deformed damage zone of the fault core, the degree of fracture development is relatively high, and the fracture density is mainly distributed at 5–15 lines/m; the fracture occurrence is relatively stable and the directionality is obvious. The degree of fracture development in the host rock is relatively low, with an average fracture density of 4.2 lines/m; the occurrence of fractures is relatively stable (Figure 5). Through the two methods mentioned above, as the distance from the fault core, the degree of fracture development decreases in a negative logarithmic function, and gradually stabilizes. Therefore, the width of the damage zone in the hanging wall of the Xike’er fault is determined to be 91.5 m, and the width of the damage zone in the footwall is determined as 41 m (Figure 5).
FIGURE 4. Orientation of fracture in the Xike’er reverse fault. Four groups of fractures are developed in the Xike’er reverse fault.
FIGURE 5. Width of damage zone of the Xike’er reverse fault. Fractures in the hanging wall of the fault are developed obviously and footwall fluid acted obviously. The blue dots indicate fracture densities at different locations in the hanging wall of the Xike’er fault, and the green dots indicate the width of the infection zone due to fluid action. The yellow area is the scope of damage zone.
From the perspective of the microstructure of different fault zone locations, the fault core, damage zone and undeformed host rock have completely different characteristics, all of which are mainly fractures, and the characteristics of fracture density distribution are: fault gouge > breccia > damage zone > host rock (Figure 6).The interparticle pore is developed in the fault breccia. The fault core has undergone significant faulting and the particle size has been significantly reduced, forming a fault gouge (Figure 6D). In terms of the mineral composition of different parts, the composition of the fault core is somewhat different from the host rock and damage zone, mainly dolomite, calcite, and quartz are developed. However, but the fault core is developed with foreign minerals such as analcite, barite, quartz, and gypsum (Figure 7).
FIGURE 6. Microstructure characteristics of host rock, fault core and damage zone. (A) Host rock (XKE 1) sample wafer microstructure. (B) Damage zone (XKE 8) sample, and the fractures are well developed, and sometimes the fractures are cemented by the calcite. (C) Fault breccia (XKE 7), more visible fractures and intergranular pore are developed under microscopic. (D) Fault gouge (XKE 9) in the fault core, embedded in a fine-grained matrix, develops intergranular pore.
FIGURE 7. Mineral composition of fault gouge and breccia zone. All four samples are located in the fault core, where XKR 9 and XKR 14 are fault gouges and the rest are fault breccias. (A,B) are mainly composed of calcite, dolomite and quartz, and contain a variety of hydrothermal minerals. (C,D) fault breccia has few mineral compositions, mainly calcite, but also contains a small part of hydrothermal minerals.
The practice of oil and gas exploration shows that the reservoir properties of matrix of Ordovician carbonate rocks in the Tarim Basin are low, and the porosity is generally less than 5%. Therefore, the Ordovician carbonate rocks belong to non-porous (tight) reservoirs. Fault breccia and cataclastic rocks are widely developed in the Xike’er fault core, and fractures of various origins are developed in damage zone. However, some fault cores and fractures in damage zone are cemented by calcite. Through the analysis of REE curve, it can be seen that the REE distribution curve of fault core calcite in the Xike’er section has obvious positive uranium (EU) anomaly, and the uranium isotope is between 2.22 and 9.14, with an average value of 6.52, which is very similar to that of hydrothermal fluorite, indicating that calcite in the fault plane is of hydrothermal fluid transformation origin. The REE curves of fault gouge, damage zone and host rock are basically consistent with those of buried calcite, reflecting the genesis of atmospheric fresh water. Minerals such as analcite, barite, quartz and gypsum are developed in the fault core, which indirectly reflects effect of the hydrothermal fluid (Figure 8). Therefore, the fault core is strongly affected by the hydrothermal fluid, while the damage zone is mainly affected by atmospheric fresh water.
FIGURE 8. Distribution characteristics of rare earth elements in different parts of fault core. (A) The REE curves of the parent rock samples show little fluctuation. (B) The REE curves of the samples in the damage zone are basically consistent with those of the late buried calcite. (C,D) REE curves of fault gouge and slip plane calcite show obvious positive Eu anomaly.
The deformation mechanism of fracture in rock mainly depends on rock porosity and buried depth (temperature and pressure) conditions. When the burial depth of non-porous rocks is less than 3 km, the fault is dominated by fracturing mechanism, which produces a large number of intergranular and intragranular fractures, forming non-cohesive fault breccia and fault gouge (Blenkinsop, 2000; Braathen et al., 2004), which evolve with the formation of fractures, and the permeability of rock.
Has increased significantly (Xiaofei et al., 2014). When the buried depth is more than 3 km, friction sliding along the faults and rolling of the particles is called cataclasis, which generates fragmentation flow, and the deformation results in the formation of fault gouge, cohesive fault breccia and fragmentation. Faults zones localize shear deformation and are made up of intensely deformed fault cores encompassed within the fault damage zones (Wibberley et al., 2008; Faulkner et al., 2010). The fault core has been transformed from the host into new rock types, such as mudstone, cataclastic rock, breccia and mylinite, due to high strain processes such as crushing, cataclastic and brecciation (Agosta and Aydin 2006; Mort and Woodcock 2008; Mitchell and Faulkner 2009; Faulkner et al., 2010; Michie 2015; Michie et al., 2021). From the microscopic characteristics (Figure 6), the particle size of fault core has been significantly reduced, forming a fault gouge. At the same time, these fault core permeability reductions are due to the pervasive cementation in the fault rocks reported here. Therefore, the fault core may contain fault rocks with permeability that are sufficiently low to act as barriers to flow. The fault gouge and breccia effect the overall across-fault fluid flow potential. Nevertheless, it is important to be able to predict the properties of fault rock that are present within a fault zone.
Field and laboratory analyses support a three-stage evolutionary model of reverse fault. At the early stage of reverse fault formation, the fault core is narrow and the damage zone of certain width is developed (Figure 9A). As a consequence of progressive consolidation, deformation, cementation, and the increase of confining stress during deformation (Marone and Scholz, 1989), Fault core and damage zone thicknesses continue increasing and porosity and permeablity is reduced. Fault core is dramatically reduced by mechanical compaction and the production of finer particles during grain fracturing and abrasion, favouring sealing behavior (Figure 9B). In the damage zone, intergranular extensional fractures and dilation bands start to develop. So, damage zones are further widened to form highly permeable formations. Development of fractures results in an effective network of secondary fracture porosity (Laubach and Ward, 2006) which strongly increases macroporosity and secondary pore connectivity. At the third stage, atmospheric water and hydrothermal fluid enter the fault zone, in which the fault core with high permeability is mainly cemented by fluid, and the damage zone forms dissolution fractures. Comprehensive testing and analysis of REE(rare earth elements) show that the fault core is sheared strongly, leading to relatively lower reservoir properties. At the same time, it is easy to be cemented by calcite under the dual action of hydrothermal and atmospheric fresh water, which is not conducive to the development of the reservoir; while the damage zone is dominated by fractures, forming a large number of high-angle fractures are conducive to the formation of fractured reservoirs. The damage zone is dominated by fracture development, forming a large number of high-angle fractures, which is conducive to the formation of fracture reservoirs (Figure 9C). The heterogeneous occurrence of intergranular extensional fracturs in the damage zone are possibly favoured by faulting and fracturing during fault history. Fault zone Evolution lead to the hydraulic differentiation between low-permeable fault core and high permeable damage zone.
FIGURE 9. | The evolution model of the reverse fault controlling the reservoir. (A) At the early stage of reverse fault formation, the fault core is narrow and the damage zone of certain width is developed. (B) Both fault cores and damage zones are further widened to form highly permeable formations. (C) Atmospheric water and hydrothermal fluid enter the fault zone, in which the fault core with high permeability is mainly cemented by fluid, and the damage zone forms dissolution fractures. It can be seen from the microstructure that the fault core is mainly cementation of non-cohesive breccia and calcite, and the damage zone is mainly developed by the fractures.
The proposed evolutionary model implies that, the permeability of fault gouge is lower, while the permeability of cohesive fault breccia zone and cataclastic rock zone is higher than that of surrounding rock. As low porosity, low permeability fault core rocks develop, they provide increasingly efficient barriers to cross-fault fluid flow, and the hydraulic behaviour of fault zones progressively changes to conduit-barrier systems. The porosity of matrix limestone shows dense physical characteristics and indicates that the primary pores were almost lost by the strong compaction. High permeability damage zones are expected to form in low permeability carbonate that faults in such rocks can channelize fluid flow by fractures. Similarly, substantial numbers of fractures or vugs and relatively high porosity in the carbonate indicating that hydrothermal alteration may play positive roles in improving the quality of the reservoir (Figure 9C). Due to the release of pressure, a large number of fractures developed, and the fracture deformation formed non-cohesive fault breccia and fault gouge (Figure 3), forming a high permeability damage zone. The Xike’er fault has a large number of fault-related fractures, which is a favorable fracture area for carbonate reservoirs (Figure 10). The damage zone is a “sweet spot” area where carbonate oil and gas are enriched, and it generally appears to be distributed along the fault in a strip.
FIGURE 10. The reservoir development rule is controlled by fracture of Xike’er reverse fault zone. The typical Xike’er profile shows that fractures are generally not developed in the host rock. The damage zone is seriously damaged, and a large number of fractures are developed, and calcite cementation and dissolution coexist. The shape of fault core changes greatly, and fault gouge, fault breccia and calcite cement are mainly developed.
1) The reverse faults in the low permeability carbonate rock are mainly fractured, and fault cores and damage zones are developed. The fault cores are developed and filled with calcite veins, fault breccia and fault gouge along the sliding surface. Damage zones are mainly developed with fractures, and some fractures are cemented by calcite.
2) The fault is the main pathway of fluid migration, and the fault core is mainly transformed by hydrothermal fluid and atmospheric fresh water, which is easy to be cemented by calcite; while the damage zone is dominated by atmospheric fresh water, which is easy to form high angle fractures and is partially cemented. Therefore, the damage zone is the “sweet spot” area of carbonate oil and gas accumulations, which generally shows strip distribution along the fault.
The original contributions presented in the study are included in the article/supplementary files, further inquiries can be directed to the corresponding author.
FG: Conceptualization, Methodology, Software, Investigation, Writing - Original Draft. HW: Validation, Data Curation, Visualization, Software, Supervision. JH: Data Curation, Visualization. PG: Data Curation Visualization.
This research was funded by the National Natural Science Foundation of China, Mechanism of fault destroying cap and quantitative evaluation of sealing (No. 41972157), and University Nursing Program for Young Scholars with Creative Talents in Heilongjiang Province, Study on deformation mechanism of strike-slip fault in carbonate rock (No. UNPYSCT-2020142).
FG and JH were employed by the company Sinopec Northwest Oilfield Company Exploration.
The remaining authors declare that the research was conducted in the absence of any commercial or financial relationships that could be construed as a potential conflict of interest.
All claims expressed in this article are solely those of the authors and do not necessarily represent those of their affiliated organizations, or those of the publisher, the editors and the reviewers. Any product that may be evaluated in this article, or claim that may be made by its manufacturer, is not guaranteed or endorsed by the publisher.
Agosta, F., and Tondi, E. (2010). Faulting and Fracturing of Carbonate Rocks: New Insights into Deformation Mechanisms, Petrophysics and Fluid Flow Properties[J]. J. Struct. Geology. 9 (32), 1185–1186. doi:10.1016/j.jsg.2010.04.008
Agosta, F., and Aydin, A. (2006). Architecture and Deformation Mechanism of a basin-bounding normal Fault in Mesozoic Platform Carbonates, central Italy. J. Struct. Geology. 28 (8), 1445–1467. doi:10.1016/j.jsg.2006.04.006
Agosta, F. (2008). Fluid Flow Properties of basin-bounding normal Faults in Platform Carbonates, Fucino Basin, central Italy. Geol. Soc. Lond. Spec. Publications 299 (1), 277–291. doi:10.1144/sp299.17
Agosta, F., Prasad, M., and Aydin, A. (2007). Physical Properties of Carbonate Fault Rocks, Fucino basin (Central Italy): Implications for Fault Seal in Platform Carbonates. Geofluids 7 (1), 19–32. doi:10.1111/j.1468-8123.2006.00158.x
Baozeng, L. (2020). Analysis of the Main Controlling Factors of Oil and Gas Differential Accumulation in the Shunbei Area, Tarim Basin-taking Shunbei No. 1 and No. 5 Strike-Slip Fault Zones as Examples[J]. China Pet. Exploration 25 (3), 83.
Bastesen, E., and Braathen, A. (2010). Extensional Faults in fine Grained Carbonates - Analysis of Fault Core Lithology and Thickness-Displacement Relationships. J. Struct. Geology. 32 (11), 1609–1628. doi:10.1016/j.jsg.2010.09.008
Bastesen, E., Braathen, A., Nøttveit, H., Gabrielsen, R. H., and Skar, T. (2009). Extensional Fault Cores in Micritic Carbonate - Case Studies from the Gulf of Corinth, Greece. J. Struct. Geology. 31 (4), 403–420. doi:10.1016/j.jsg.2009.01.005
Bastesen, E., Braathen, A., and Skar, T. (2013). Comparison of Scaling Relationships of Extensional Fault Cores in Tight Carbonate and Porous sandstone Reservoirs. Pet. Geosci. 19 (4), 385–398. doi:10.1144/petgeo2011-020
Berg, S. S., and Skar, T. (2005). Controls on Damage Zone Asymmetry of a normal Fault Zone: Outcrop Analyses of a Segment of the Moab Fault, SE Utah. J. Struct. Geology. 27 (10), 1803–1822. doi:10.1016/j.jsg.2005.04.012
Billi, A., Salvini, F., and Storti, F. (2003). The Damage Zone-Fault Core Transition in Carbonate Rocks: Implications for Fault Growth, Structure and Permeability. J. Struct. Geology. 25 (11), 1779–1794. doi:10.1016/s0191-8141(03)00037-3
Blenkinsop, Thomas. G. (2000). Deformation Mincrostructures and Meachnisms in Minerals and rocks[M]//Deformation Microstructures and Mechanisms in Minerals and Rocks. Kluwer Academic Publishers.
Braathen, A., Osmundsen, P. T., and Gabrielsen, R. H. (2004). Dynamic Development of Fault Rocks in a Crustal- Scale Detachment: An Example from Western Norway [J]. Tectonics 23, 4. doi:10.1029/2003tc001558
Caine Saul, J., Evans, J. P., and Forster, C. B. (1996). Fault Zone Architecture and Permeability structure.[J]. Geology.
Changyu, F., Braathen, A., Zhenliang, W., Xiaoqiang, Z., Suiying, C., Nana, F., et al. (2019). Flow Pathway and Evolution of Water and Oil along Reverse Faults in the Northwestern Sichuan Basin, China [J]. AAPG Bull. 103 (5), 1153–1177. doi:10.1306/10261816501
Chengzao, J. (1997). Structural Characteristics and Hydrocarbon Accumulation in Tarim Basin, China[M]. Pet. industry press.
Chester, F. M., and Logan, J. M. (1986). Implications for Mechanical Properties of Brittle Faults from Observations of the Punchbowl Fault Zone, California[J]. Pure Appl. Geophys. 124 (1), 79–106. doi:10.1007/bf00875720
Chester, F. M., Chester, J. S., Kirschner, D. L., Schulz, S. E., and Evans, J. P. (2004). 8. Structure of Large-Displacement, Strike-Slip Fault Zones in the Brittle Continental Crust [M]//Rheology and Deformation of the Lithosphere at Continental Margins. Columbia University Press, 223–260. doi:10.7312/karn12738-009
Choi, J.-H., Yang, S.-J., Han, S.-R., and Kim, Y.-S. (2015). Fault Zone Evolution during Cenozoic Tectonic Inversion in SE Korea. J. Asian Earth Sci. 98, 167–177. doi:10.1016/j.jseaes.2014.11.009
Cooke, A. P., Fisher, Q. J., Michie, E., and Yielding, G. (2019). Permeability of Carbonate Fault Rocks: a Case Study from Malta[J]. Pet. Geosci. 26 (3), petgeo2019–055. doi:10.1144/petgeo2019-055
Cooke, A. P., Fisher, Q. J., Michie, E. A., and Yielding, G. (2018). Investigating the Controls on Fault Rock Distribution in normal Faulted Shallow Burial Limestones, Malta, and the Implications for Fluid Flow. J. Struct. Geology. 114, 22–42. doi:10.1016/j.jsg.2018.05.024
Crider, J. G., and Peacock, D. C. P. (2004). Initiation of Brittle Faults in the Upper Crust: a Review of Field Observations. J. Struct. Geology. 26 (4), 691–707. doi:10.1016/j.jsg.2003.07.007
De Joussineau, G., and Aydin, A. (2007). The Evolution of the Damage Zone with Fault Growth in sandstone and its Multiscale Characteristics[J]. J. Geophys. Res. Solid Earth 112 (B12). doi:10.1029/2006jb004711
Engelder, J. T. (1974). Cataclasis and the Generation of Fault Gouge. Geol. Soc. America Bull. 85 (10), 1515–1522. doi:10.1130/0016-7606(1974)85<1515:catgof>2.0.co;2
Evans, J. P., Forster, C. B., and Goddard, J. V. (1997). Permeability of Fault-Related Rocks, and Implications for Hydraulic Structure of Fault Zones. J. Struct. Geology. 19 (11), 1393–1404. doi:10.1016/s0191-8141(97)00057-6
Faulkner, D. R., Jackson, C. A. L., Lunn, R. J., Schlische, R. W., Shipton, Z. K., Wibberley, C. A. J., et al. (2010). A Review of Recent Developments Concerning the Structure, Mechanics and Fluid Flow Properties of Fault Zones. J. Struct. Geology. 32 (11), 1557–1575. doi:10.1016/j.jsg.2010.06.009
Flodin, E. A., and Aydin, A. (2004). Evolution of a Strike-Slip Fault Network, Valley of Fire State Park, Southern Nevada[J]. Geol. Soc. America Bull. 116 (1-2), 42–59. doi:10.1130/b25282.1
Fossen, H., Schultz, R. A., Shipton, Z. K., and Mair, K. (2007). Deformation Bands in sandstone: a Review. J. Geol. Soc. 164 (4), 755–769. doi:10.1144/0016-76492006-036
Fu, X. F., Xu, P., Wei, C. Z., and Lü, Y. F. (2012). Internal Structure of normal Fault Zone and Hydrocarbon Migration and Conservation[J]. Earth Sci. Front. 19 (6), 200–212.
Gaviglio, P., Bekri, S., Vandycke, S., Adler, P. M., Schroeder, C., Bergerat, F., et al. (2009). Faulting and Deformation in Chalk. J. Struct. Geology. 31 (2), 194–207. doi:10.1016/j.jsg.2008.11.011
Hausegger, S., Kurz, W., Rabitsch, R., Kiechl, E., and Brosch, F.-J. (2010). Analysis of the Internal Structure of a Carbonate Damage Zone: Implications for the Mechanisms of Fault Breccia Formation and Fluid Flow. J. Struct. Geology. 32 (9), 1349–1362. doi:10.1016/j.jsg.2009.04.014
Jiao, F. Z. (2018). Significance and prospect of Ultra-deep Carbonate Fault-Karst Reservoirs in Shunbei Area, Tarim Basin[J]. Oil Gas Geology. 39 (2), 207–216. doi:10.11743/ogg20180201
Kaminskaite, I., Fisher, Q. J., and Michie, E. H. (2019). Impact of Laboratory-Induced Deformation and Naturally-Occurring Faults on Fluid Flow in Carbonates[C]//Fifth International Conference on Fault and Top Seals.
Laubach, S. E., Eichhubl, P., Hargrove, P., Ellis, M. A., and Hooker, J. N. (2014). Fault Core and Damage Zone Fracture Attributes Vary along Strike Owing to Interaction of Fracture Growth, Quartz Accumulation, and Differing sandstone Composition. J. Struct. Geology. 68, 207–226. doi:10.1016/j.jsg.2014.08.007
Laubach, S. E., and Ward, M. E. (2006). Diagenesis in Porosity Evolution of Opening-Mode Fractures, Middle Triassic to Lower Jurassic La Boca Formation, NE Mexico. Tectonophysics 419, 75–97. doi:10.1016/j.tecto.2006.03.020
Li, Y. T., Qi, L. X., Zhang, S. N., Yun, L., and Cao, Z. C. (2019). Characteristics and Development Mode of the Middle and Lower Ordovician Fault-Karst Reservoir in Shunbei Area, Tarim Basin[J]. Acta Petrolei Sinica 40, 1470–1484.
Lin, C., Yang, H., Liu, J., Peng, L., Cai, Z., Yang, X., et al. (2009). Paleostructural Geomorphology of the Paleozoic central Uplift belt and its Constraint on the Development of Depositional Facies in the Tarim Basin. Sci. China Ser. D-earth Sci. 52 (6), 823–834. doi:10.1007/s11430-009-0061-8
Lin, C., Yang, H., Liu, J., Rui, Z., Cai, Z., Li, S., et al. (2012). Sequence Architecture and Depositional Evolution of the Ordovician Carbonate Platform Margins in the Tarim Basin and its Response to Tectonism and Sea-Level Change. Basin Res. 24, 559–582. doi:10.1111/j.1365-2117.2011.00536.x
Lingdong, M., Xiaofei, F. U., Yachun, W., Xiaoling, Z., and Yanfang, L. (2014). Internal Structure and Sealing Properties of the Volcanic Fault Zones in Xujiaweizi Fault Depression, Songliao Basin, China[J]. Pet. Exploration Dev. 41 (2), 165–174. doi:10.1016/S1876-3804(14)60019-7
Lubiniecki, D. C., White, S. R. S., King, R. C., Holford, S. P., Bunch, M. A., and Hill, S. M. (2019). Structural Evolution of Carbonate-Hosted Cataclastic Bands Adjacent to a Major Neotectonic Fault, Sellicks Beach, South Australia. J. Struct. Geology. 126 (SEP), 11–24. doi:10.1016/j.jsg.2019.05.004
Marone, C., and Scholz, C. H. (1989). Particle-size Distribution and Microstructures within Simulated Fault Gouge. J. Struct. Geology. 11, 799–814. doi:10.1016/0191-8141(89)90099-0
Michie, E. A. H. (2015). Influence of Host Lithofacies on Fault Rock Variation in Carbonate Fault Zones: A Case Study from the Island of Malta. J. Struct. Geology. 76, 61–79. doi:10.1016/j.jsg.2015.04.005
Michie, E. A. H., and Haines, T. J. (2016). Variability and Heterogeneity of the Petrophysical Properties of Extensional Carbonate Fault Rocks, Malta. Pet. Geosci. 22 (2), 136–152. doi:10.1144/petgeo2015-027
Michie, E. A. H., Kaminskaite, I., Cooke, A. P., Fisher, Q. J., Yielding, G., and Tobiss, S. D. (2021). Along-strike Permeability Variation in Carbonate-Hosted Fault Zones. J. Struct. Geology. 142, 104236. doi:10.1016/j.jsg.2020.104236
Mitchell, T. M., and Faulkner, D. R. (2009). The Nature and Origin of Off-Fault Damage Surrounding Strike-Slip Fault Zones with a Wide Range of Displacements: A Field Study from the Atacama Fault System, Northern Chile. J. Struct. Geology. 31 (8), 802–816. doi:10.1016/j.jsg.2009.05.002
Mort, K., and Woodcock, N. H. (2008). Quantifying Fault Breccia Geometry: Dent Fault, NW England. J. Struct. Geology. 30 (6), 701–709. doi:10.1016/j.jsg.2008.02.005
Ngia, N. R., Hu, M., and Da, G. (2019). Tectonic and Geothermal Controls on Dolomitization and Dolomitizing Fluid Flows in the Cambrian-Lower Ordovician Carbonate Successions in the Western and central Tarim Basin, NW China. J. Southeast Asian Earth Sci. 172, 359–382. doi:10.1016/j.jseaes.2018.09.020
Panza, E., Agosta, F., Rustichelli, A., Zambrano, M., Tondi, E., Prosser, G., et al. (2016). Fracture Stratigraphy and Fluid Flow Properties of Shallow-Water, Tight Carbonates: The Case Study of the Murge Plateau (Southern Italy). Mar. Pet. Geology. 73, 350–370. doi:10.1016/j.marpetgeo.2016.03.022
Rotevatn, A., Thorsheim, E., Bastesen, E., Fossmark, H. S. S., Torabi, A., and Sælen, G. (2016). Sequential Growth of Deformation Bands in Carbonate Grainstones in the Hangingwall of an Active Growth Fault: Implications for Deformation Mechanisms in Different Tectonic Regimes. J. Struct. Geology. 90, 27–47. doi:10.1016/j.jsg.2016.07.003
Rotevatn, A., Fossmark, H. S., Bastesen, E., Thorsheim, E., and Torabi, A. (2017). Do deformation Bands Matter for Flow? Insights from Permeability Measurements and Flow Simulations in Porous Carbonate Rocks[J]. Pet. Geosci. 23 (1). doi:10.1144/petgeo2016-038
Shipton, Z. K., and Cowie, P. A. (2003). A Conceptual Model for the Origin of Fault Damage Zone Structures in High-Porosity sandstone. J. Struct. Geology. 25 (3), 333–344. doi:10.1016/s0191-8141(02)00037-8
Sibson, R. H. (1977). Fault Rocks and Fault Mechanisms. J. Geol. Soc. 133 (3), 191–213. doi:10.1144/gsjgs.133.3.0191
Środoń, J., Drits, V. A., McCarty, D. K., Hsieh, J., and Eberl, D. D. (2001). Quantitative X-ray Diffraction Analysis of clay-bearing Rocks from Random Preparations[J]. Clays and Clay Minerals 49 (6), 514–528. doi:10.1346/CCMN.2001.0490604
Vermilye, J. M., and Scholz, C. H. (1999). Fault Propagation and Segmentation: Insight from the Microstructural Examination of a Small Fault. J. Struct. Geology. 21 (11), 1623–1636. doi:10.1016/s0191-8141(99)00093-0
Walsh, J. J., Watterson, J., Heath, A. E., and Childs, C. (1998). Representation and Scaling of Faults in Fluid Flow Models. Pet. Geosci. 4 (3), 241–251. doi:10.1144/petgeo.4.3.241
Wenyuan, H., Jiang-hai, L., Xiang-lin, Q., and Zheng, D. (2002). Analysis of Fault Structures in the Kalpin Fault Uplift, Tarim Basin[J]. Geology. China 1, 37–43. doi:10.3969/j.issn.1000-3657.2002.01.007
Wibberley, C. A. J., Yielding, G., and Di Toro, G. (2008). Recent Advances in the Understanding of Fault Zone Internal Structure: a Review. Geol. Soc. Lond. Spec. Publications 299 (1), 5–33. doi:10.1144/sp299.2
Woodcock, N. H., Sayers, N. J., and Dickson, J. A. D. (2008). Fluid Flow History from Damage Zone Cements Near the Dent and Rawthey Faults, NW England. J. Geol. Soc. 165 (4), 829–837. doi:10.1144/0016-76492007-133
Xiaofei, F., Jianhua, X., and Lingdong, M. (2014). Fault Deformation Mechanisms and Internal Structure Characteristics of Fault Zone in Pure Sandstone[J]. J. Jilin Univ. Earth Sci. Edition 44 (1), 25–37. doi:10.13278/j.cnki.jjuese.201401103
Xiaofei, F., Xiaoyu, S., and Lingdong, M. (2013). Internal Structure of Fault Zone and Oil/gas Reservior in Low- Porosity Rock[J]. J. Cent. South Univ. Sci. Tech. Edition 44 (6), 2428–2438.
Yang, D., Tailiang, F., and Zhiqian, G. (2016). Geochemical Characteristics and Their Implications to Diagenetic Environment of Lower-Middle Ordovician Carbonate Rocks, Tarim Basin, China:A Case Study of Bachu Dabantage Outcrop and Aksu Penglaiba Outcrop[J]. Nat. Gas Geoence 27 (8).
Zhiwen, D., Rujun, W., Fangfang, C., Jianping, Y., Zhongqian, Z., Zhimin, S., et al. (2020). Origin, Hydrocarbon Accumulation and Oil-Gas Enrichment of Fault-Karst Carbonate Reservoirs: A Case Study of Ordovician Carbonate Reservoirs in South Tahe Area of Halahatang Oilfield, Tarim Basin[J]. Pet. Exploration Dev. 47 (2), 306–317. doi:10.11698/PED.2020.02.07
Keywords: thrust fault, faulting and fracturing, deformation mechanism, damage zone, carbonate, Tarim basin
Citation: Geng F, Wang H, Hao J and Gao P (2021) Internal Structure Characteristics and Formation Mechanism of Reverse Fault in the Carbonate Rock, A Case Study of Outcrops in Xike’er Area, Tarim Basin, Northwest China. Front. Earth Sci. 9:793016. doi: 10.3389/feart.2021.793016
Received: 11 October 2021; Accepted: 11 November 2021;
Published: 01 December 2021.
Edited by:
Wei Ju, China University of Mining and Technology, ChinaCopyright © 2021 Geng, Wang, Hao and Gao. This is an open-access article distributed under the terms of the Creative Commons Attribution License (CC BY). The use, distribution or reproduction in other forums is permitted, provided the original author(s) and the copyright owner(s) are credited and that the original publication in this journal is cited, in accordance with accepted academic practice. No use, distribution or reproduction is permitted which does not comply with these terms.
*Correspondence: Haixue Wang, d2FuZ2hhaXh1ZTExNkAxNjMuY29t
Disclaimer: All claims expressed in this article are solely those of the authors and do not necessarily represent those of their affiliated organizations, or those of the publisher, the editors and the reviewers. Any product that may be evaluated in this article or claim that may be made by its manufacturer is not guaranteed or endorsed by the publisher.
Research integrity at Frontiers
Learn more about the work of our research integrity team to safeguard the quality of each article we publish.