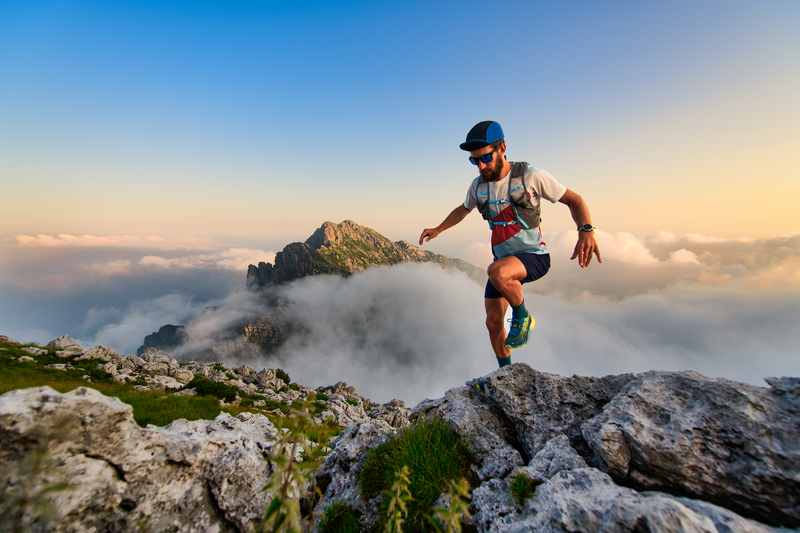
94% of researchers rate our articles as excellent or good
Learn more about the work of our research integrity team to safeguard the quality of each article we publish.
Find out more
ORIGINAL RESEARCH article
Front. Earth Sci. , 11 October 2021
Sec. Economic Geology
Volume 9 - 2021 | https://doi.org/10.3389/feart.2021.766011
This article is part of the Research Topic Unconventional Natural Gas Geoscience View all 24 articles
To reveal the evolution law of coal skeleton deformation during the process of CO2 flooding and displacing CH4 in coal seam, a fluid-solid coupling mathematical model of CO2 injection enhanced CH4 drainage was established based on Fick’s law, Darcy’s law, ideal gas state equation, and Langmuir equation. Meanwhile, numerical simulations were carried out by implementing the mathematical model in the COMSOL Multiphysics. Results show that the CH4 content of both regular gas drainage and CO2 enhanced gas drainage gradually decreases with time, and the decreasing rate is high between 10 and 60 days. Compared with regular gas drainage, the efficiency of CO2 enhanced gas drainage is more obvious with greater amount of CH4 extracted out. When coal seam gas is extracted for 10, 60, 120, and 180 days, CH4 content in coal seam is reduced by 5.2, 17.2, 23.6, and 26.7%, respectively. For regular gas drainage, the deformation of coal skeleton is dominated by the shrink of coal matrix induced by gas desorption, and the strain curve shows a continuous downward trend. For CO2 enhanced gas drainage, the strain curve of coal skeleton showed a decrease—rapid increase—slow increase trend. The evolution of permeability is opposite to the evolution of coal skeleton strain. Higher gas injection pressure will lead to a greater coal skeleton strain. The pumping pressure affects the deformation of coal skeleton slightly compared with that of initial water saturation and initial temperature. Greater initial water saturation leads to larger deformation of coal skeleton in the early stage. The strain value of coal skeleton gradually tends to be consistent as gas injection prolongs. Higher initial temperature leads to greater reduction in coal skeleton strain when the gas injection continues. Research achievements provide a basis for the field application of CO2 injection enhanced CH4 drainage in underground coal mines.
Coal seam gas is a by-product of coal mining, mainly composed of CH4 (Fan et al., 2017). Coal seam gas is a clean energy source. However, coal and gas outbursts and gas explosions often occur with coal mining, which severely restricts the safe and efficient production of coal mines (Huo et al., 2019). With the increase of mining depth, the permeability of coal seam gradually decreases, resulting in the increase of difficulty of gas drainage. Therefore, improving the efficiency of coal seam gas drainage is a key technology for preventing and controlling mine gas disasters, as well as the development and utilization of gas resources (Guo et al., 2020).
Scholars have explored the method of replacing CH4 by injecting waste gas into the coal seam. Generally, the injected gas includes CO2, N2, and flue gas (Wu et al., 2019). The adsorption capacity of coal for CO2, N2, and CH4 is CO2 > CH4 > N2 through a series of studies, which provides a certain theoretical basis for coal seam gas injection (Song et al., 2019, Fan et al., 2019a, Yi et al., 2013). N2 mainly displaces coal bed methane by changing the pressure gradient in the coal-rock fractures (Lin et al., 2018), and the competitive adsorption effect is small. CO2 can not only displace the free CH4 in fractures, but also compete with the gas on the adsorption site to replace it (Liu et al., 2017; Fang et al., 2019a). Busch et al. (2003) carried out CO2 and CH4 mixed binary gas adsorption and desorption experiments on different coal ranks under the same conditions, and found that the adsorption capacity of CO2 is stronger than that of CH4 (Liu et al., 2019; Wang et al., 2018). Reznik et al. (1984) conducted experiments by injecting CO2 into bituminous coal containing CH4 and bituminous coal containing water under high pressure, and proved that CO2 injection can increase the recovery rate of CH4 by 2–3 times and obtained higher gas injection pressure can lead to higher recovery rate of CH4 (Chattaraj et al., 2016, Lin et al., 2017). Baran et al. (2014) used the volumetric method to conduct adsorption experiments under lower and higher pressures, and proved that CO2 is the best gas that can penetrate into the internal structure of coal. The above experiments show that CO2 injection can flood or displace coal seam CH4 and improve the efficiency of CH4 drainage. To explore the mechanism of CO2 injection enhanced CH4 recovery, scholars from various countries have carried out numerical simulation on the basis of physical experiments. Vishal et al. (2015) studied the effect of adsorption time on CO2 enhanced coal bed methane recovery (CO2-ECBM). Zhou et al. (2012) and Fang et al. (2019b) used COMSOL Multiphysics software to analyze the evolution of permeability in the CO2-ECBM process, and the results show that the effective stress changes, matrix shrinkage, and swelling caused by pumping pressure of drainage and CO2 injection pressure are the key factors affecting permeability. Gas injection pressure and temperature are the two key factors that affect the efficiency of CH4 extraction (Fang et al., 2019c). Fan et al. (2018) considered non-isothermal adsorption and analyzed the effects of different injection pressures and initial temperatures on CO2-ECBM. Additionally, Pan et al. (2019) studied the evolution of its microstructural changes under high-pressure methane adsorption/desorption. Wang et al. (2020) revealed the specific process of coal macromolecular rearrangement caused by CO2 injection through molecular dynamics.
However, predecessors have seldom studied the deformation law of coal skeleton in the process of gas injection to displace coal seam CH4. In this article the coal mass is considered a dual pore structure composed of pores and fractures, and a fluid-solid coupling model for gas injection enhanced methane drainage is established. The COMSOL Multiphysics software is used to study the coal skeleton strain law in the process of CO2 injection to displace coal seam CH4 on the background of Zhangcun coal mine in Shanxi Provence. The results will provide a reference for improving the CH4 drainage from coal seams during underground mining.
According to the occurrence characteristics of gas in coal reservoirs, the following assumptions are made (Fan et al., 2016; Li et al., 2016; Ren et al., 2017; Fan et al., 2019b): 1) The coal mass is a porous medium with double pores composed of pores and fracture; 2) The gas migration in the matrix satisfies Fick’s law of diffusion, and the gas migration process in the fracture satisfies Darcy’s law; 3) CH4 and CO2 are regarded as ideal gases; 4) Water only migrates in the fractures; 5) The adsorption and desorption of CH4 and CO2 in coal mass are carried out under constant temperature conditions.
Assume that the coal seam is a dual-porosity unidirectional permeable medium composed of a matrix, as shown in Figure 1. Matrix pores are the main storage space for CH4 and CO2, and the change of fractures affects the evolution of permeability. Therefore, the changes of pores and fractures are the key factors in the process of CO2 down whole injection enhanced CH4 drainage by effects of flooding and displacement.Where a0 is the initial matrix width, m; q is the initial fracture width, m.
FIGURE 1. Physical model of coal mass: (A) actual coal surface, (B) coal structure model, and (C) representative element volume (REV).
The coal matrix porosity model can be expressed as (Fan et al., 2016):
where
Expression of matrix swelling strain caused by gas adsorbed on coal (Cao et al., 2019):
where ρc is the density of coal, kg/m3; R is gas molar constant, J/(mol·K); T is the temperature in the coal seam, K; ai is the limit adsorption capacity of gas component i, m3/kg; bi is the adsorption equilibrium constant of gas component i, MPa−1; pmgi is pressure of gas component i in the matrix, Pa; Vm is the molar volume of gas, L/mol.
Considering the influence of stress and seepage effects, the fracture porosity model can be obtained as (Fan et al., 2019b):
where φf0 is the initial fracture porosity;
According to the cubic law, the relationship between porosity and permeability is:
where k0 is the initial permeability of the coal seam, m2.
Substituting Eq. 3 into Eq. 4 can obtain the dynamic evolution equation of permeability:
The relative permeability model of gas-water two-phase flow is (Xu et al., 2014):
where krg0 is the endpoint relative permeability of the gas; sw is the saturation of water; swr is the irreducible water saturation; sgr is the residual gas saturation fraction; krw0 is the endpoint relative permeability of water.
According to the ideal gas state equation, the density of each component gas under standard conditions is:
where pa is standard atmospheric pressure, kPa.
Generalized Langmuir equation for binary gas adsorption equilibrium:
where cpi is the content of gas component i in coal, kg/m3; ρgi is the density of gas component i under standard conditions, kg/m3; Mgi is the molar mass of gas component i, g/mol.
The gas content in the coal matrix per unit volume equals the sum of the free gas content and the adsorbed gas content, which is obtained from the Langmuir equation and the ideal gas equation of state:
Owing to the influence of gas injection and drainage, the original equilibrium state of gas in the coal seam is broken. Forced by the concentration gradient, the gas in the coal matrix migrates into the fractures by diffusion. According to Fick’s law of diffusion, the gas mass in the matrix can be conserved. The equation is (Ren et al., 2017):
where pfgi is the pressure of gas component i in the fracture, MPa; τi is the desorption time of gas component i, d.
Substituting Eqs 7–9 into Eq. 10, the gas transport equation in the matrix can be obtained as:
Considering the gas slippage effect and the generalized Darcy law of gas-water two-phase flow, the transport flows of gas and water are gained respectively (Fan et al., 2019c):
where b is the Klinkenberg factor, MPa; μgi is the dynamic viscosity of gas component i, MPa·s; μw is the dynamic viscosity of water, MPa·s.
Then, the mass conservation equation for gas migration in the fractures is:
where sg is the gas saturation in fracture, sg+ sw= 1.
The water seepage controlling equation is:
Considering that the total strain of coal mass is the sum of strain caused by stress, fluid pressure in matrix pores and fractures, and coal matrix swelling resulted from CH4 and CO2 adsorption. The stress field controlling equation is (Li et al., 2016):
where
Taking the 2,606 roadway of Zhangcun Coal Mine in Shanxi Provence as the background, the feasibility of CO2 injection to increase gas drainage was studied in terms of its high gas and enrich water combined condition. COMSOL Multiphysics software is adopted to numerically solve the established fluid-solid coupling mathematical model. The 2,606 roadway is buried in a depth of 537 m, the coal seam temperature is 298.15 K, and the gas content during advancing is 8.5–10.0 m3/t. As shown in Figure 2, a two-dimensional physical geometry model with 6 m × 16 m in size was built for the simplification of coal wall on 2,606 roadway. There are five boreholes (two for gas injection and three for drainage) arranged along the center line of the roadway with borehole spacing of 2.5 m. Line A-B is set as the observation reference of simulate results, as well as the point C (9.25 m, 3 m), point D (11.75 m, 3 m), point E (14.5 m, 3 m). Both the sides of the model are set as roller boundary condition. The overburden loading of 14.85 MPa is applied on the upper side. The extern sides of the model are set as impermeable boundaries, indicating that no gas flows at these boundaries. The bottom side is set as a fix boundary. The borehole wall of the borehole is set as the pressure boundary condition with a pumping pressure of 20 kPa and an injection pressure of 1.0 MPa. The other used parameters are shown in Table 1. These parameters are mainly recovered from field tests and laboratory experiments, as well as recorded in other articles (Fan et al., 2016; Fan et al., 2019a; Fan et al., 2019b; Fang et al., 2019b; Fang et al., 2019c).
Figure 3 shows the contour map of coal bed gas pressure at 10, 60, 120, and 180 days of regular gas drainage and CO2 enhanced gas drainage. In Figure 3A, without CO2 injection, the gas pressure continues to decrease with the increase of extraction time. The vertical direction decreases faster than the horizontal direction leading by the supplemental gas sources in the horizontal direction. The vertical direction is closer to the coal seam boundary and there is no supplementary gas source.
FIGURE 3. Coal bed gas pressure distribution of both regular and CO2 enhanced gas drainage at different times. (A) Regular gas drainage. (B) CO2 enhanced gas drainage.
As CO2 is continuously injected into coal seam, the gas pressure shows a downward tendency in whole, but the changes near the drainage borehole and the gas injection borehole are different, as illustrated in Figure 3B. The pressure around the gas injection borehole is also gradually decreasing. The pressure around the gas injection borehole drops slower than that near the extraction borehole. And the drop rate in the vertical direction is slower than that in the horizontal direction. This is because the vicinity of the injection borehole is largely affected by CO2 flows. Although the CH4 pressure decreases with the effects of extraction, the CO2 pressure is rising, resulting in slowly drops of pressure. The gas pressure in the vertical direction of injection borehole is less affected by the pumping pressure of the drainage.
Figure 4 presents the contour of CH4 content at 10, 60, 120, and 180 days of both regular and CO2 enhanced gas drainage in coal seam. Figure 4A shows the variation of CH4 content after regular gas drainage. The CH4 content reduction area expands from the center of drainage borehole, but the reduction region and rate are relatively slow. On the contrary, the reduction region and rate of CH4 content at the same duration for CO2 injection enhanced CH4 drainage have been significantly improved. This is caused by the combined action of CO2 injection and CH4 drainage. The potential pressure gradient in the coal seam drives the seepage of CO2 and displacement of free CH4 in the fractures. When most of the free CH4 in the fractures is driven out, the injected CO2 will compete with the CH4 adsorbed inside and on the surface of the coal, and then replace the adsorbed CH4. This accelerates the desorption rate of CH4. In Figure 4B, CH4 presents the trend of change.
FIGURE 4. Contour of CH4 content of both regular and CO2 enhanced gas drainage at different times. (A) Regular gas drainage. (B) CO2 enhanced gas drainage.
Figure 5 presents the evolution of the CH4 content on the observation line A-B with time. In Figure 5A CH4 content of both regular gas drainage and CO2 enhanced gas drainage gradually decreases with time, and the decreasing rate is highest between 10 and 60 days. CH4 pressure in coal seam gradually decreases (pressure gradient between the drainage borehole and coal seam) as the extraction time prolongs. In other words, the pressure gradient and the CH4 flow rate gradually decrease, slowing down the decreasing rate of CH4 content. Compared with the regular gas drainage, the effect of CO2 enhanced gas drainage in coal seam is more obvious with greater amount of CH4 extracted out. The reason is that CO2 drives and competitively adsorbs with CH4, which enhances the efficiency CH4 drainage.
FIGURE 5. Evolution of CH4 content on the reference line AB with both regular and CO2 enhanced gas drainage. (A) Regular gas drainage. (B) CO2 enhanced gas drainage.
The peak CH4 content between the extraction borehole and the gas injection borehole for 10, 60, 120, and 180 days of regular gas drainage are 8.62, 6.87, 5.55, and 4.57 m3/t, respectively. The peak CH4 content of CO2 enhanced gas drainage are 8.17, 5.69, 4.24, and 3.35 m3/t respectively, which reduced by 5.2, 17.2, 23.6, and 26.7% compared with that of regular gas drainage, respectively. The drainage efficiency has been significantly improved.
Figure 6 shows the strain and permeability of coal skeleton adsorbed gas during regular gas drainage and CO2 enhanced gas drainage. From Figure 6A, the strain value of coal skeleton behaves a gradual downward trend on the reference points (C, D, E) for the regular gas drainage. This is because the free CH4 in fractures flows out driven by the pressure gradient, and the decrease of gas pressure in fractures triggers desorption of adsorbed gas in coals, as a result that coal matrix will shrink and deform. The strain at point E is slightly smaller than that at other points at the same time due to the influence of a single drainage hole. There is a short period of plateau on the coal permeability curve at the beginning followed by gradual increase. The change in permeability is mainly affected by effective stress and matrix shrinkage. On the one hand, with the pumping pressure of drainage, the free CH4 in fractures is discharged, and the adsorbed CH4 begins to desorb. As a result, CH4 pressure in coal seam drops. The effective stress increases, to compress the seepage channel in coal mass, and subsequently coal permeability decreases. On the other hand, the permeability increases caused by the CH4 desorption induced shrinkage of coal matrix. The two opposite aspects work together to decide the change of coal seam permeability. In early stage, the seepage effect dominates, and the desorption rate of CH4 is slow, leading to the infinitesimal change in coal permeability. When the free CH4 is gradually discharged, the desorption rate begins to increase, and the permeability begins to rise.
FIGURE 6. Changes in strain and permeability of coal skeleton on the reference points (C, D, E) during regular and CO2 enhanced gas drainage. (A) Regular gas drainage. (B) CO2 enhanced gas drainage.
In Figure 6B, the strain on reference points C and D shows a slight decrease—rapid increase—slow increase trend in the process of CO2 enhanced gas drainage. CH4 desorption under the combined action of CO2 displacement and pumping pressure of drainage at the initial stage plays a leading role. Then, free CH4 in the coal fractures decreases and CO2 increases. CO2 competes with the CH4 in matrix for adsorption, and the strain value of coal skeleton increases as the coal matrix undergoes swelling deformation caused by the stronger adsorption affinity of CO2 than that of CH4. Finally, CO2 gradually reaches adsorption equilibrium, and the strain curve gradually slows down. Point E is mainly affected by the drainage hole, and its strain value continues to decrease. Figure 6B shows that the evolution curve of permeability is opposite to that of coal skeleton strain.
The CH4 desorption in coal matrix contracts leading to the decrease of coal skeleton strain. Then, as the free CH4 in the coal fractures decreases, CO2 increases and CO2 competes with the CH4 in the matrix to adsorb. The coal matrix expands and deforms, and the strain value of the coal skeleton rapidly increases. Finally, the competitive adsorption of CO2 gradually tends to balance since most of the adsorbed CH4 in the matrix is replaced. The competitive adsorption of CO2 gradually tends to balance. The CO2 adsorption rate slows down, and the strain value of coal skeleton slowly increases.
We will explore different factors affecting the deformation of coal skeleton during CO2 injection to enhanced CH4 drainage from coal seam. The controlled single variable method was used to analyze the influence of different gas injection pressures, pumping pressures, initial water saturations, and coal seam temperatures on coal skeleton deformation. The simulation schemes of different influencing factors are shown in Table 2.
Figure 7A presents the strain curve on the reference point D under different gas injection pressures during CO2 enhanced gas drainage. In general, higher gas injection pressure will lead to greater strain of coal skeleton. Higher injection pressure under the same condition will lead to greater amount of adsorbed CO2, as well as greater swelling deformation of coal matrix. If the injection pressure is low, the matrix contraction in the early stage is great, and the curve is easy to slow down. When the initial injection pressure is higher than CH4 pressure in coal seam, CO2 cannot transport from high pressure to low pressure area. At this time, the coal skeleton strain is mainly affected by the desorption of CH4. CH4 pressure in coal seam gradually decreases as the action of pumping pressure. When CH4 pressure drops below the injection pressure, CO2 can flow under the action of the pressure gradient. The lower the injection pressure of CO2, the smaller the pressure gradient formed. As a result, the adsorption equilibrium reaches earlier.
FIGURE 7. Evolution of coal skeleton strain on point D under different parameters during CO2 enhanced gas drainage. (A) Different gas injection pressures. (B) Different pumping pressures. (C) Different initial water saturations. (D) Different initial temperatures.
In Figure 7B, the change of pumping pressure of drainage affects the deformation of coal skeleton slightly.
Figure 7C shows the strain curve of coal skeleton on the reference point D at different initial water saturations. Greater initial water saturation will lead to greater deformation of the coal skeleton in the early stage, and the strain value of coal skeleton gradually tends to be consistent as the operation time of gas injection prolongs. The reason is the water in fractures flow out will carry out part of the free CH4 and CO2 in the coal. As a result, the desorption rate of CH4 is accelerated and the adsorption rate of CO2 is slowed down. The shrinkage scale of the coal matrix becomes larger. As most of the water flows out of the borehole, the water saturation of coal seams with different water saturations tends to be consistent, and the deformation of coal skeleton also tends to be consistent.
Figure 7D shows the coal skeleton strain on the reference point D at different initial temperatures. The higher initial temperature will lead to greater reduction in the coal skeleton strain value, particularly when the operation time of gas injection prolongs.
1) A fluid-solid coupling mathematical model of CO2 injection enhanced CH4 drainage in coal seam was established based on Fick’s law, Darcy’s law, ideal gas state equation, and Langmuir equation. Meanwhile, numerical simulations on CO2 injection enhanced CH4 drainage during underground mining were carried out using the established model.
2) The CH4 content of both regular and CO2 enhanced gas drainage gradually decreases with time, and the decreasing rate is high between 10 and 60 days. Compared with regular gas drainage, CO2 enhanced gas drainage effect is more obvious with greater amount of CH4 extracted out. When the CH4 in coal seam is extracted for 10, 60, 120, and 180 days, the CH4 content in coal seam is reduced by 5.2, 17.2, 23.6, and 26.7%, respectively.
3) For regular gas drainage, the deformation of the coal skeleton is dominated by the contraction of coal matrix induced by gas desorption, and the strain curve shows a continuous downward trend. In the process of CO2 enhanced gas drainage, the strain curve of coal skeleton showed a slight decrease— rapid increase—slow increase trend. The evolution curve of permeability is opposite to that of coal skeleton strain.
4) Higher gas injection pressure will lead to greater coal skeleton strain. The pumping pressure affects the deformation of coal skeleton slightly compared with the initial water saturation and initial temperature. Greater initial water saturation will lead to larger deformation of coal skeleton in the early stage, and the strain value of coal skeleton gradually tends to be consistent as the operation time of gas injection prolongs. Higher initial temperature leads to greater reduction in coal skeleton strain, particularly when the gas injection prolongs continuously.
The raw data supporting the conclusion of this article will be made available by the authors, without undue reservation.
CF write and financially support this paper; LY design the research scheme and write this paper; GW financially support and correct this paper; QH derive the equations of this paper; HW carry out simulations.
This research was financially supported by the National Natural Science Foundation of China (Grant Nos. 52174117, 52004117, and 51874159), the Research Fund of Key Laboratory of Mining Disaster Prevention and Control (Grant No. MDPC202008), the Basic Research Project of Key Laboratory of Liaoning Provincial Education Department (Grant No. LJ2020JCL005), and the Project supported by the Postdoctoral Science Foundation of China (Grant Nos. 2021T140290, 2020M680975), the Project supported by discipline innovation team of Liaoning Technical University (Grant no. LNTU20TD-30).
The authors declare that the research was conducted in the absence of any commercial or financial relationships that could be construed as a potential conflict of interest.
All claims expressed in this article are solely those of the authors and do not necessarily represent those of their affiliated organizations, or those of the publisher, the editors and the reviewers. Any product that may be evaluated in this article, or claim that may be made by its manufacturer, is not guaranteed or endorsed by the publisher.
The author(s) would like to thank all editors and reviewers for their comments and suggestions.
Baran, P., Zarębska, K., Krzystolik, P., Hadro, J., and Nunn, A. (2014). CO2-ECBM and CO2 Sequestration in Polish Coal Seam - Experimental Study. J. Sustainable Min. 13 (2), 22–29. doi:10.7424/jsm140204
Busch, A., Krooss, B. M., Gensterblum, Y., van Bergen, F., and Pagnier, H. J. M. (2003). High-Pressure Adsorption of Methane, Carbon Dioxideand Their Mixtures on Coals with a Special Focus on the Preferential Sorption Behaviour. J. Geochem. Explor. 78-79, 671–674. doi:10.1016/s0375-6742(03)00122-5
Cao, Z., Lin, B., and Liu, T. (2019). The Impact of Depositional Environment and Tectonic Evolution on Coalbed Methane Occurrence in West Henan, China. Int. J. Min. Sci. Technol. 29 (2), 297–305. doi:10.1016/j.ijmst.2019.01.006
Chattaraj, S., Mohanty, D., Kumar, T., and Halder, G. (2016). Thermodynamics, Kinetics and Modeling of Sorption Behaviour of Coalbed Methane - A Review. J. Unconv. Oil Gas Resour. 16, 14–33. doi:10.1016/j.juogr.2016.09.001
Fan, C. J., Li, S., Luo, M. K., Yang, Z. H., Zhang, H. H., and Wang, S. (2016). Numerical Simulation of Deep Coalbed Methane Extraction Based on Fluid-Solid-thermal Coupling. J. China Coal Society 41 (12), 3076–3085. (in Chinese with English abstract).
Fan, C., Li, S., Luo, M., Du, W., and Yang, Z. (2017). Coal and Gas Outburst Dynamic System. Int. J. Min. Sci. Technol. 27 (01), 49–55. doi:10.1016/j.ijmst.2016.11.003
Fan, Y., Deng, C., Zhang, X., Li, F., Wang, X., and Qiao, L. (2018). Numerical Study of CO2-enhanced Coalbed Methane Recovery. Int. J. Greenhouse Gas Control. 76, 12–23. doi:10.1016/j.ijggc.2018.06.016
Fan, C., Elsworth, D., Li, S., Zhou, L., Yang, Z., and Song, Y. (2019). Thermo-hydro-mechanical-chemical Couplings Controlling CH4 Production and CO2 Sequestration in Enhanced Coalbed Methane Recovery. Energy 173, 1054–1077. doi:10.1016/j.energy.2019.02.126
Fan, C., Elsworth, D., Li, S., Chen, Z., Luo, M., Song, Y., et al. (2019). Modelling and Optimization of Enhanced Coalbed Methane Recovery Using CO2/N2 Mixtures. Fuel 253, 1114–1129. doi:10.1016/j.fuel.2019.04.158
Fan, C., Li, S., Luo, M., Yang, Z., and Lan, T. (2019). Numerical Simulation of Hydraulic Fracturing in Coal Seam for Enhancing Underground Gas Drainage. Energy Explor. Exploit. 37 (1), 166–193. doi:10.1177/0144598718785998
Fang, H. H., Sang, S. X., and Liu, S. Q. (2019). The Coupling Mechanism of the thermal-hydraulic- Mechanical fields in CH4-Bearing Coal and its Application in the CO2-enhanced Coalbed Methane Recovery. J. Petrol. Sci. Eng. 181, 106177. doi:10.1016/j.petrol.2019.06.041
Fang, H., Sang, S., and Liu, S. (2019). Establishment of Dynamic Permeability Model of Coal Reservoir and its Numerical Simulation during the CO2-ECBM Process. J. Pet. Sci. Eng. 179, 885–898. doi:10.1016/j.petrol.2019.04.095
Fang, H.-H., Sang, S.-X., and Liu, S.-Q. (2019). Numerical Simulation of Enhancing Coalbed Methane Recovery by Injecting CO2 with Heat Injection. Pet. Sci. 16 (1), 32–43. doi:10.1007/s12182-018-0291-5
Guo, D., Lv, P., Zhao, J., and Zhang, C. (2020). Research Progress on Permeability Improvement Mechanisms and Technologies of Coalbed Deep-Hole Cumulative Blasting. Int. J. Coal Sci. Technol. 7 (2), 329–336. doi:10.1007/s40789-020-00320-5
Huo, B., Jing, X., Fan, C., and Han, Y. (2019). Numerical Investigation of Flue Gas Injection Enhanced Underground Coal Seam Gas Drainage. Energy Sci. Eng. 7 (6), 3204–3219. doi:10.1002/ese3.491
Li, S., Fan, C., Han, J., Luo, M., Yang, Z., and Bi, H. (2016). A Fully Coupled thermal-hydraulic-mechanical Model with Two-phase Flow for Coalbed Methane Extraction. J. Nat. Gas Sci. Eng. 33, 324–336. doi:10.1016/j.jngse.2016.05.032
Lin, J., Ren, T., Wang, G., Booth, P., and Nemcik, J. (2017). Experimental Study of the Adsorption-Induced Coal Matrix Swelling and its Impact on ECBM. J. Earth Sci. 28 (5), 917–925. doi:10.1007/s12583-017-0778-9
Lin, J., Ren, T., Wang, G., Booth, P., and Nemcik, J. (2018). Experimental Investigation of N2 Injection to Enhance Gas Drainage in CO2-rich Low Permeable Seam. Fuel 215, 665–674. doi:10.1016/j.fuel.2017.11.129
Liu, J., Yao, Y., Liu, D., and Elsworth, D. (2017). Experimental Evaluation of CO2 Enhanced Recovery of Adsorbed-Gas from Shale. Int. J. Coal Geol. 179, 211–218. doi:10.1016/j.coal.2017.06.006
Liu, J., Xie, L., Elsworth, D., and Gan, Q. (2019). CO2/CH4 Competitive Adsorption in Shale: Implications for Enhancement in Gas Production and Reduction in Carbon Emissions. Environ. Sci. Technol. 53 (15), 9328–9336. doi:10.1021/acs.est.9b02432
Pan, J., Lv, M., Hou, Q., Han, Y., and Wang, K. (2019). Coal Microcrystalline Structural Changes Related to Methane Adsorption/desorption. Fuel 239, 13–23. doi:10.1016/j.fuel.2018.10.155
Ren, T., Wang, G., Cheng, Y., and Qi, Q. (2017). Model Development and Simulation Study of the Feasibility of Enhancing Gas Drainage Efficiency through Nitrogen Injection. Fuel 194, 406–422. doi:10.1016/j.fuel.2017.01.029
Reznik, A. A., Singh, P. K., and Foley, W. L. (1984). An Analysis of the Effect of CO2 Injection on the Recovery of In-Situ Methane from Bituminous Coal: An Experimental Simulation. Soc. Pet. Eng. J. 24 (05), 521–528. doi:10.2118/10822-PA
Song, Y., Jang, B., and Lan, F. J. (2019). Competitive Adsorption of CO2/N2/CH4 onto Coal Vitrinite Macromolecular: Effects of Electrostatic Interactions and Oxygen Functionalities. Fuel 235, 23–38./doi:10.1016/j.fuel.2018.07.087
Vishal, V., Singh, T. N., and Ranjith, P. G. (2015). Influence of Sorption Time in CO2 -ECBM Process in Indian Coals Using Coupled Numerical Simulation. Fuel 139, 51–58. doi:10.1016/j.fuel.2014.08.009
Wang, T., Tian, S., Li, G., Sheng, M., Ren, W., Liu, Q., et al. (2018). Molecular Simulation of CO2/CH4 Competitive Adsorption on Shale Kerogen for CO2 Sequestration and Enhanced Gas Recovery. J. Phys. Chem. C 122 (30), 17009–17018. doi:10.1021/acs.jpcc.8b02061
Wang, K., Pan, J., Wang, E., Hou, Q., Yang, Y., and Wang, X. (2020). Potential Impact of CO2 Injection into Coal Matrix in Molecular Terms. Chem. Eng. J. 401, 126071. doi:10.1016/j.cej.2020.126071
Wu, S., Deng, C., and Wang, X. (2019). Molecular Simulation of Flue Gas and CH4 Competitive Adsorption in Dry and Wet Coal. J. Nat. Gas Sci. Eng. 71, 102980. doi:10.1016/j.jngse.2019.102980
Xu, H., Tang, D. Z., Tang, S. H., Zhao, J. L., Meng, Y. J., and Tao, S. (2014). A Dynamic Prediction Model for Gas-Water Effective Permeability Based on Coalbed Methane Production Data. Int. J. Coal Geology 121, 44–52. doi:10.1016/j.coal.2013.11.008
Yi, H., Li, F., Ning, P., Tang, X., Peng, J., Li, Y., et al. (2013). Adsorption Separation of CO2, CH4, and N2 on Microwave Activated Carbon. Chem. Eng. J. 215-216, 635–642. doi:10.1016/j.cej.2012.11.050
Keywords: coal seam, CO2 injection enhanced CH4 drainage, coal skeleton deformation, numerical simulation, fluid-solid coupling model
Citation: Fan C, Yang L, Wang G, Huang Q, Fu X and Wen H (2021) Investigation on Coal Skeleton Deformation in CO2 Injection Enhanced CH4 Drainage From Underground Coal Seam. Front. Earth Sci. 9:766011. doi: 10.3389/feart.2021.766011
Received: 28 August 2021; Accepted: 15 September 2021;
Published: 11 October 2021.
Edited by:
Jienan Pan, Henan Polytechnic University, ChinaReviewed by:
Ting Liu, China University of Mining and Technology, ChinaCopyright © 2021 Fan, Yang, Wang, Huang, Fu and Wen. This is an open-access article distributed under the terms of the Creative Commons Attribution License (CC BY). The use, distribution or reproduction in other forums is permitted, provided the original author(s) and the copyright owner(s) are credited and that the original publication in this journal is cited, in accordance with accepted academic practice. No use, distribution or reproduction is permitted which does not comply with these terms.
*Correspondence: Chaojun Fan, Y2hhb2p1bmZhbkAxMzkuY29t; Gang Wang, Z2FuZy53YW5nQHNkdXN0LmVkdS5jbg==
Disclaimer: All claims expressed in this article are solely those of the authors and do not necessarily represent those of their affiliated organizations, or those of the publisher, the editors and the reviewers. Any product that may be evaluated in this article or claim that may be made by its manufacturer is not guaranteed or endorsed by the publisher.
Research integrity at Frontiers
Learn more about the work of our research integrity team to safeguard the quality of each article we publish.