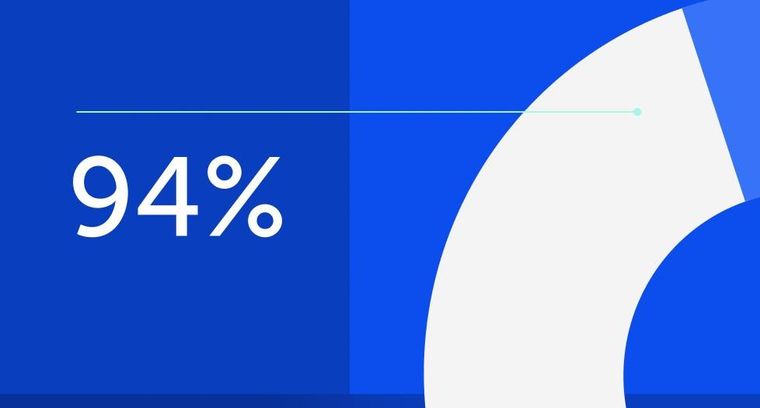
94% of researchers rate our articles as excellent or good
Learn more about the work of our research integrity team to safeguard the quality of each article we publish.
Find out more
ORIGINAL RESEARCH article
Front. Earth Sci., 16 December 2021
Sec. Hydrosphere
Volume 9 - 2021 | https://doi.org/10.3389/feart.2021.762703
Establishing worldwide sustainable and phosphorus efficient cropping systems is urgently needed because the supply of suitable phosphate rock is limited, and excess phosphorus in streams causes eutrophication. One of the impediments in the developing world for sustainable P practices is the lack of studies on P transport and its eventual disposition in the environment. One of these regions with few studies is the Ethiopian Highlands, with permeable volcanic soils. The objective was to establish baseline data on P watershed export in the (sub)humid highlands. Two contrasting watersheds were selected near Lake Tana. For 2 years, stream discharge and sediment, total P, dissolved P, and bioavailable particulate P concentrations were determined at the watershed outlet. The first watershed is the 57 km2 Dangishta, with lava intrusion dikes, forcing subsurface flow through faults to the surface and preventing gully formation. Subsurface flow was half of the 1745 mm annual precipitation, and surface runoff and erosion were minimal. The second watershed is the 9 km2 Robit Bata with 1,420 mm precipitation. The banks of several river banks were slumping. The upper part of the watershed generates saturation excess runoff. A hillslope aquifer in the lower part provided interflow. The average sediment concentrations of 10.5 g L−1 in the stream in Robit Bata (11 times that in Dangishta) reflected the sediments from banks slipping in the stream. The hydrology and the soil loss directly affected the phosphorus export. In Dangishta, the total P concentration averaged 0.5 mg L−1 at the outlet. In Robit Bata, the average total P concentration was 2 mg L−1. The bioavailable particulate P concentration was only twice the concentration in the runoff water. The low phosphorus content of the subsoil slipping in Robit Bata moderated biologically available particulate P at the outlet. Average dissolved P concentrations for both watersheds were around 0.1 mg L−1 in the low range found in temperate climates. It reflects the difference in length of time that phosphorus fertilizers have been applied. Our research concludes that commonly implemented practices such as strengthening river banks and stabilizing gully might not lead to improved water quality in Lake Tana.
As a result of the increasing population and intensification of agriculture, anthropogenic phosphorus pollution is reaching dangerously high in freshwater basins worldwide (Bennett et al., 2001; Mekonnen and Hoekstra, 2018). It leads to eutrophication resulting in seasonal hypoxia (Rabalais et al., 2010), harmful algal blooms, and production losses in aquatic ecosystems (Turner et al., 2006; Li et al., 2014; Watson et al., 2016). Moreover, the supply of the most suitable phosphate rock is limited, and more efficient use of phosphorus improves the life of the P reserves and prevents lake eutrophication.
While the phosphorus loads in water bodies have decreased in rural settings in the USA and Europe (Nyenje et al., 2010), the opposite occurred in major lakes in sub-Saharan Africa. For example, Lake Victoria has become eutrophic (Gikuma-Njuru et al., 2005). In Lake Tana, the largest Lake in Ethiopia, P concentrations have exceeded 0.08 mg L- and are the main driver behind the recent water hyacinth expansion (Dersseh et al., 2019). Best management practices need to be developed to prevent further water quality degradation in the many African Lakes. The design of best practices in the humid monsoon climate with over 1 m precipitation in 4 months requires understanding how the complex hydrogeology interacts with the P transport. One of these regions is the Ethiopian Highlands, where sediment loss and P concentration in rivers and lakes are increasing, despite governmental sponsored projects constructing best management practices on agricultural land.
In humid tropical Ethiopian Highlands, where infiltration rates are generally greater than the rainfall intensities, runoff is produced by saturation excess from periodically saturated areas (Guzman et al., 2013; Tilahun et al., 2013). As soils saturate during the monsoon, the groundwater tables intersect with the soil surface. Overland flow may occur on the degraded hillsides where perched water tables, in soils with a hardpan, reach the surface during the storm. In areas with little vegetation cover, this can lead to significant erosion, especially when the saturated areas are prone to gulley formation (Guzman et al., 2013; Steenhuis et al., 2013; Tilahun et al., 2013; Zegeye et al., 2016; Dagnew et al., 2017; Zegeye et al., 2018; Mhiret et al., 2019). In addition, hydrology in Ethiopia’s volcanic landscape is affected by cracks, fissures, faults, and pipes. Watersheds in the headwaters usually cannot close their water budget. Thirty to seventy percent of effective rain (rainfall – evaporation) leaves the watershed through faults, not the outlet. For example, east of Lake Tana, 60% of the effective rain flows to the Takeze watershed in the Rib watershed (Adem et al., 2020). Another example is the drinking water in Bahir Dar that comes from a spring in the lower Gilgel Abay. These springs have the same outflow pattern as rivers, with most flows during the rain and decreased flows during the dry phases (Nigate et al., 2016).
Sediment concentrations in watersheds in the Ethiopian highlands were the greatest at the start of the rain phase due to the formation of rills in the plowed cropland (Guzman et al., 2013; Steenhuis et al., 2013; Tilahun et al., 2013; Zegeye et al., 2016; Dagnew et al., 2017; Zegeye et al., 2018). Later in the rain phase, expansion of the valley bottom gullies (up to 10 m deep!) became the primary source of the sediment (Tebebu et al., 2010; Zegeye et al., 2016). Basin-wide estimated soil loss ranged from 25 to 37 Mg ha⁻1 a⁻1 for watersheds with gullies (Lemann et al., 2016; Zimale et al., 2016; Haregeweyn et al., 2017; Zimale et al., 2018). At the same time, watersheds without gullies generally had losses of less than 10 Mg ha⁻1 a⁻1 (Moges et al., 2016; Adem et al., 2020).
Phosphorus can be lost in the dissolved and particulate form. The portion of the sediment-associated P can be biologically available (bio-available particulate phosphorus or BAPP) (Uusitalo et al., 2001; Ellison and Brett 2006; Bender et al., 2018). In lakes, an equilibrium exists between the sediment-associated BAPP and dissolved P (Reynolds and Davies 2001; Ruttenberg and Sulak 2011). Catchment properties control the transport of the dissolved and sediment-associated P forms, including land use and management, topography, hydrological and erosion-sedimentation processes (Bowes et al., 2005; Donn et al., 2012; Chow et al., 2017). Up to 92% of the annual total P load can be lost during storm events (Gao et al., 2010).
Throughout the landscape, features such as agricultural land, gullies, flood plains, wetlands can act as a source or a sink depending on their location and P form (Moges et al., 2016; Macintosh et al., 2018; Ezzati et al., 2020). Riparian buffer strips, vegetated valley bottomlands act as a sink for particulate P but a source for dissolved P (Heathwaite 2010; Collins et al., 2013). Moges et al. (2016a) and Guzman et al. (2017) noted that dissolved P in the stream during baseflow was equal to the groundwater P concentration in the saturated valley bottoms. The seasonally or inter-storm relationships of concentration-discharge (C-Q) could be investigated by plotting the P concentration as a function of the discharge (Thomas et al., 2016; Moatar et al., 2017; Heathwaite and Bieroza 2021).
Despite the recent publications on hydrology and sediment transport process and dynamics in the Ethiopian highlands (e.g., Guzman et al., 2013; Zegeye et al., 2018), our understanding of P export remains limited. In the Science Citation Index, we found three studies with limited P export data for watersheds by Moges et al. (2016) and Guzman et al. (2017) and Alemu et al. (2017). In addition, two plot studies were published that report on P export from various land uses by Grum et al. (2017) and Mulualem et al. (2021). Dersseh et al. (2019) and Kebedew et al. (2020) measured phosphorus concentration and the degree of eutrophication. Thus, the objective is twofold. 1) to establish a baseline for phosphorus losses from a watershed and 2) to quantify the effect of runoff mechanisms on dissolved, particulate, and bioavailable P. A better understanding of the bioavailable phosphorus and dissolved phosphorus fractions is essential for adaptive management of terrestrial and aquatic ecosystems (Scavia et al., 2014).
Dangishta (57 km2) and Robit Bata (9 km2) watersheds are found in the northern Ethiopian Highlands, within the Lake Tana basin (Figure 1). Dangishta is located within in upper reaches of the Gilgel Abay that drains to Lake Tana. The climate is a moist sub-humid with an annual temperature variation between 10°C and 25°C and a yearly average rainfall of 1745 mm during the last 10 years at the National metrological Agency (NMA) weather station 5.6 km from the watershed outlet. The rainfall distribution is a unimodal type with 90% that occurs between May to October. Robit-Bata, at the south-eastern edge of Lake Tana, drains direct to Lake Tana. It has a sub-humid climate with temperatures between 11°C and 27°C. The annual rainfall is 1,420 mm, of which 85% falls between May and September.
FIGURE 1. Dangishta and Robit Bata watersheds in Lake Tana basin. (A) Ethiopia with the location of the two watersheds and Lake Tana. (B) The topographic map of Dangishta and (C) the topographic map of Robit Bata. The coordinates are in UTM.
The topographic characteristics of both watersheds vary. Dangishta’s elevations range from 2036 to 2,400 m (Figure 1). The stream length is 34 km. More than 78% of the area is flat to undulating, with slopes less than 8% (Table 1). Compared with Dangishta, Robit Bata is at a lower altitude with elevations that range from 1828 m near the outlet to 2030 m at the upper part of the watershed. The stream length is 6.1 km. Only 55% of the area in Robit Bata is flat to undulating.
The geology of both watersheds is characterized by massive basalt bedrock overlaid by different soil types (Walker and Yimam 2018; Tilahun et al., 2020). The primary soil types in Dangishta are Regosols, Alisols, Nitosols, and Vertisols (Yimam et al., 2018). The soil layer over bedrock thickness increases from the bottom to the upper part of the watershed (David Walker et al., 2018). In Robit Bata, Vertisols are predominantly found in the lowland areas, and Eutric Nitosols in the upper part of the watershed (Abidela Hussein et al., 2019). Deep soils are located around the middle slope and decline in depth downslope and upslope. The upper half of the watershed is severely degraded with shallow soils, and the hillsides are characterized by hillslope aquifers (Tilahun et al., 2020).
Both watersheds have highly conductive surface soils. The infiltration rate is only exceeded by rainfall intensity 2.5% of the time (Yimam et al., 2018; Tilahun et al., 2020). Otherwise, the watersheds are hydrologically very different. In Dangishta, after the rain infiltrates, excess water flows laterally through faults in the volcanic rock. It appears as springs downstream in the watershed where the flow is blocked by volcanic dikes, creating a water table close to the surface during the rain phase (Figures 2A,B). Like other seepage areas, the lateral conductivity is high (Mendoza and Steenhuis 2002; Zhao et al., 2020). Eucalyptus or grassland covering approximately 32% of the watershed (Table 2) are found on these seasonally saturated soils. The volcanic dikes (Figure 2A) which are across the stream also prevent gully formation.
FIGURE 2. Photos depicting features in the Dangishta and Robit Bata watersheds: (A) grassland with volcanic dike indicated by the rocks area in Dangishta valley bottom. The grassland is saturated during the rain phase. (B) Spring in the grassland in Dangishta; the light blue oily shine on the water is from the reduced iron at the location where the water surfaces; the brown deposit is the oxidized iron. (C) Degraded upland in Robit Bata; (D,E) the gully and banks that slip when saturated during a rainstorm upstream of the weir at the outlet in the Robit Bata. All photos were made during the 2021 rain phase, except for photo (C) taken early in the 2014 dry phase.
In Robit Bata, the degraded upslope soils with thin soil (Figure 2C) become rapidly saturated during a rainfall event resulting in saturation excess runoff (Tilahun et al., 2020) and, therefore, a much quicker response time to runoff generation than Dangishta. Volcanic dikes are absent in Robit Bata. Hence an active gully is located upstream of the flume at the watershed outlet (Figures 2D,E). In Robit Bata, which has few seasonally saturated areas, the combined acreage of grassland and eucalyptus is under 10%.
In both watersheds, crop-livestock farming is dominant. Approximately 65% in Dangishta and 74% in Robit Bata of land is cultivated during the rain phase (Table 2; Figure 3). Rainfed cereal crops are “tef” (Eragrostis tef), maize (Zea mays), finger millet (Eleusine coracana), and barley (Hordeum vulgare). Soils are prepared at the onset of the rainy season in June and July using oxen-drawn plows. Fertilizers for rainfed crops are applied in the form of DAP (di-ammonium phosphate) and blended fertilizer NPSZnB (nitrogen, phosphorus, sulfur, zinc and boron), manure, and compost in split doses in July and mid-August. According to the local Bureau of Agriculture, the average applied P fertilizers in Robit Bata watershed was 21 kg P ha−1, and in Dangishta, 20 kg P ha−1.
In Robit Bata, small-scale irrigation in the dry season (October to December) using shallow groundwater is practiced on 117 ha or 13% of the land (Table 2; Figure 3). The main irrigated crop is khat, a mild narcotic perineal small bush, tomatoes, green pepper, and onions are grown as well with irrigation. The applied fertilizer dose for vegetables and khat varies among farmers, but the average application is 6 kg ha−1 P. Irrigated acreage in Dangishta was negligible.
Discharge, suspended sediment concentration, total and bioavailable particulate and dissolved phosphorus were measured at the outlet of both watersheds in 2017–2018. In addition, the physical and chemical soil properties and precipitation were measured.
In Dangishta, the rainfall was recorded both years with a tipping bucket (Figure 1). The number of tips was recorded every 10 min. Missing precipitation data were obtained by linear regressing the locally measured data with the rainfall recorded at the National Meteorological Agency (NMA) weather station located at a 5.3 km distance (Supplementary Figure S1). The coefficient of determination R2 was 0.83.
In 2017, precipitation data for Robit Bata were obtained from the National Meteorological Agency (NMA) Zenzelima weather located at a 3.4 km distance. In 2018, precipitation was measured on-site using a tipping bucket which recorded rainfall at 5-min intervals.
Stream discharge was measured by manually recording stage height at the outlet of the watersheds with paid local observers (Figure 1). In Robit Bata, the gauging station was at the concrete culvert under the highway from Bahir Dar to Gondar. In Dangishta, the gauging station was situated in the natural stream channel where the bedrock was at the surface. During storm events, water flow depth was recorded at 10-min intervals starting when the streamflow became turbid. Measurements were halted when the flow depth returned to base flow levels. Baseflow was measured twice a day at 6:00 AM and 6:00 PM. Each stage reading was accompanied by a surface flow velocity measurement using the float method over a distance of 10 m. By taking the 90% of surface velocity, the mean velocity was obtained. Discharge was calculated as the product of wetted cross-sectional area and mean velocity. The stage-discharge curve was obtained by plotting the discharge obtained from the surface velocity against flow depths (Supplementary Figures S2, S3).
Samples of 34 events in Dangishta and 47 events in Robit Bata were collected over the 2-year study period. Depending on the rainfall magnitude, rainfall-runoff response and falling limb, between 4 and 15 water samples were collected per storm event. The water samples were taken with an interval of 30 min for Dangishta and 10 min for Robit Bata as the rainfall response and lag time differed between both watersheds. The samples were filtered using 32-cm diameter Whatman filter paper with a pore size of 2.5 µm. The filter paper and soil were oven-dried at 105 °C for 24 h and weighed to determine the sediment mass captured per liter of discharge.
Phosphorus concentration of the various fractions was analyzed using 500 ml water samples collected simultaneously as sediment determination. The total P was determined by acid digestion of the unfiltered sample according to the method in (Baird et al., 2017). The dissolved P was measured in water filtered through Whatman filter paper with 0.45 µm pore size. The bioavailable particulate phosphorus was determined by NaOH chemical extraction using 180 ml of the unfiltered sample. The detail of the method is described in Sharpley et al. (1992). The BAPP concentration is the difference between extracted NaOH P concentration and the DP concentration. All P forms were measured using the standard molybdenum blue method and detected by UV/Vis spectrophotometer lambda 32 at 880 nm (Murphy and Riley 1962).
Soil sampling was conducted on May 18, 2017 for Dangishta and June 5, 2017 for Robit Bata. Five composite soil samples were collected from the topsoil at 0–10 cm depth under rainfed cultivated land, eucalyptus tree and grassland in each watershed. A total of 30 composite soil samples, 15 from each watershed, were analyzed. Air-dried samples were sieved through a 2-mm sieve and analyzed to determine organic matter using Walkley-Black method (Walkley and Black 1934) and available P (Pca) by Bray I method (Bray and Kurtz 1945). Soil texture was determined using the hydrometer method (Beretta et al., 2014).
A hydrograph separation was performed to evaluate potential differences in rainfall-runoff responses in both watersheds. The discharge hydrograph was separated into surface runoff components (storm event flow), baseflow, and interflow. The Parameter Efficient Distributed (PED) hydrological model was employed for the flow separation.
The PED (Parameter Efficient Distributed) model is a semi-distributed hydrological model developed for the sub-humid Ethiopian highlands. The model has been widely applied in the highlands of northern Ethiopian (Collick et al., 2009; Steenhuis et al., 2009; Tessema et al., 2010; Zimale et al., 2017; Moges et al., 2017; Guzman et al., 2017a,b; Tilahun et al., 2013, 2015, 2020; Ochoa-Tocachi et al., 2018). The landscape is divided into four units, three of which contribute flow to the outlet and are simulated by the PED model. The PED is run at a daily time step. It updates the water balances for the three zones, calculates groundwater tables for the three zones and predicts discharge at the outlet consisting of interflow, baseflow and direct runoff at a daily time step (Tilahun et al., 2013a, 2015). More information about the model is provided in the Supplementary Material Section S1.
Monthly loads of sediment and P fractions were calculated based on the observed concentrations in the baseflow measured once a day and 34 storm events in Dangishta, and 47 storm events in Robit Bata. The monthly flow weighted average concentrations,
where n is the number of measurements in the mth month. The monthly load
where for the mth month,
Sediment and phosphorus concentrations were plotted as a function of the discharge, similar to Guzman et al. (2013). Like Liu et al. (2008) and Guzman et al. (2013), the scatter in the data was reduced by dividing the rainy monsoon phase into several groups. Grouping was based on cumulative effective precipitation, Rce defined as precipitation minus the reference evapotranspiration (P-ET). Groups of storms in the “early” period occurred when cumulative effective rainfall, Rce was less than 150 mm (Rce < 150) and in between 150 and 300 mm (150 < Rce < 300). Another group of storms occurring between 300 and 450 mm (300 < Rce < 450) and between 450 and 800 mm, delineated as the middle part of the rainy season. Finally, the storms occurring between 800 and 1,200 mm (800 < Rce < 1,200) or greater than 1,200 mm (Rce > 1,200) are defined as the late part of the rainy season.
For the hysteresis loops in the concentration discharge relationship, storm events were selected with a minimum of the two concentrations measurements on each rising and falling limb. Eighteen storms in Dangishta and 27 in Robit Bata fulfilled this requirement. The sediment, total P, and biological available P hysteresis loops were characterized according to the methodology proposed by Lawler et al. (2006) and later modified by Lloyd et al. (2016). The direction of the hysteresis loop can be either clockwise, counter clockwise, or mixed.
One-way ANOVA multiple mean comparison test was used to compare the mean scores of soil properties in two watersheds for different groups of land uses (eucalyptus trees rainfed cropland and grassland). The non-parametric Kruskal-Wallis test was employed to investigate the link between sediment-associated bioavailable phosphorus (BAPP) in the storm event samples and soil available P under three different land uses. R version 4.0.2 was used for data analysis and visualization, and SigmaPlot 12.5 for statistical analysis.
The soil texture of the topsoil in both watersheds was similar and could be classified texture-wise either as clay loam or loam. Soils in Dangishta had a clay content of 32%, which was slightly greater than Robit Bata at 25% (Table 3). Soils are of volcanic origin, with soils in Dangishta being more acidic than in Robit Bata. Cropland in Robit Bata had the lowest organic matter content. In Dangishta, the organic matter was also less than for eucalyptus trees and grasslands. The soil available phosphorus was significantly greater in Dangishta than in Robit Bata (Table 3). Due to fertilizer applications, the soil available P in the cropland was significantly greater than in either the grassland or eucalyptus plantations in both watersheds.
TABLE 3. Statistical summary of soil physical and chemical properties of top 10 cm for the dominant land uses in Dangishta and Robit Bata watersheds.
The daily rainfall of Dangishta and Robit Bata in the 2 years is shown in Figure 4. The highest recorded daily event was 123 mm on 7/21/2017 (Robit Bata) and 60.5 mm on 8/28/2017 (Dangishta). The intensity of the rainfall ranged between 0.02 and 1.4 mm min⁻1 in Dangishta and between 0.05 and 1.8 mm min⁻1 in Robit Bata (Supplementary Table S1). Generally, these rainfall intensities were less than the soil infiltration rates except in saturated areas (Tilahun et al., 2020).
FIGURE 4. The hydrograph shows baseflow and interflow separated from the total discharge using the PED model for (A) Dangishta and (B) Robit Bata watershed.
The PED model was used for hydrograph separation by simulating the subsurface and direct runoff at the outlet. Five parameters were calibrated: the portion of the watershed with a shallow restrictive layer, periodically saturated and located near the stream, deep soils that contained the aquifer. The aquifer in Dangishta was simulated as a first-order reservoir with a halflife of 15 days and Robit Bata as a zero-order reservoir with a travel time of 80 days from the groundwater divide to the stream. The calibrated values of the parameters are discussed below and formally shown in Table S1.1 in Section 1 of the supplementary material.
In both watersheds, approximately half of the rainfall became recharge. However, the rainfall-runoff response was distinctly different. In Dangishta, the water balance closed, and the precipitation minus the evaporation was nearly equal to the discharge at the outlet over the 2 years. As expected, the greater rainfall in Dangishta resulted in greater discharge (Figure 4; Table 4). In Robit Bata, the water balance did not close. Analysis with the PED model showed that only 80% of the precipitation could be accounted for as discharge on an annual basis after subtracting the evaporation. In Dangishta, the subsurface flow was blocked by volcanic dikes and surfaced as springs before the outlet. In Robit Bata, flow through the faults was not blocked and therefore could pass by the watershed outlet.
TABLE 4. Summary of rainfall and measured discharge and the PED predicted flow components (surface runoff, subsurface flow) (mm a⁻1) over the study period of 2017–2018 for the Dangishta watershed.
The contribution of surface runoff and subsurface flow (both interflow and baseflow) to the total discharge is shown in Figure 4. The hydrograph separation with the PED model showed that almost all discharge at the outlet in the Dangishta was subsurface flow. Only a small portion (less than 5%) was direct runoff (Supplementary Table S1.1). It is not uncommon that most water leaves the watershed as interflow and baseflow in volcanic tropical watersheds (Toohey et al., 2018).
Although the subsurface flow contribution in Robit Bata was around 67%, it is much lower than in Dangishta (Table 4). A part of the simulated subsurface flow, the interflow originated from approximately 10% of the area near the river and had a short travel time as described in (Supplementary Table S1.1). The remaining subsurface flow from 50% of the watershed area had a long travel time. The river flow disappeared within about 4 months after the rain phase ended (Figure 4B). The direct runoff generated on the shallow soils over bedrock (Tilahun et al., 2020) was in addition to the subsurface flow. The direct runoff per unit area was 14–18%, much greater than in Dangishta. The greatest storm discharge was also recorded in Robit Bata. Approximately one-fifth of the surficial watershed did not contribute flow to the outlet. Fewer springs were observed in Robit Bata than in Dangishta and reflected less interflow contribution.
Representative examples of concentration of the suspended sediment and P in the discharge at the Dangishta and Robit Bata outlet during individual storms are shown in Figure 5. Differences in flow paths between the catchments resulted in different sediment and P removal rates. In Dangishta, except for the two events (Figures 5D,E), sharply increasing peak concentrations of particulate-associated P fractions were absent because interflow and baseflow were dominant at the outlet. The suspended sediment and associated particulate P are negligible compared with the concentration in the surface runoff (Steenhuis et al., 2009). The event hydrographs (grey shaded) in Robit Bata are characterized by surface runoff and resulted in shorter duration, higher and sharper discharge peaks than Dangishta, mainly due to subsurface flow (Figure 5). In Robit Bata, the sediment and total P (TP and BAPP) similar to the discharge exhibited high peaks. The peaks occurred predominantly before the peak discharge (Figures 5H,J–L).
FIGURE 5. Representative hydrographs with concentrations of suspended sediment (SS) and total (TP), particulate bioavailable (BAPP), and dissolved (DP) phosphorus during the rain phase of the 2017 and 2018 study period. (A–F) Dangishta; (G–L) and Robit Bata.
In Robit Bata, most sediment at the outlet originated from the channel section with unstable banks slipping in the channel (Figures 2D,E). The sediment peak concentrations in Robit Bata ranged from 20 g L−1 or 2% (Figure 5L) to 60 g L−1 or 6% (Figures 5H,J). These high sediment concentrations have been observed in watersheds with actively eroding gullies in Ethiopia (Zegeye et al., 2018; Akale et al., 2017).
The DP concentration in the discharge at the outlet behaved differently compared to the particulate P and sediment concentrations (Figure 5). Overall, DP concentrations varied little throughout the events in Dangishta. In Robit Bata, the greatest DP concentrations were generally observed during the recession flow (i.e., the falling limb). In the discussion, we will examine the differences in sediment and P concentrations and loads in the two watersheds.
Over the entire 2017–2018 study period, on average, the concentrations in the discharge at the outlet in Robit Bata compared to Dangishta were 11 times greater for sediment, four times for TP, a factor of two for BAPP, and in the same order for DP (Figure 6). Precipitation (and therefore discharge) starts in Dangishta earlier than in Robit Bata, and thus Dangishta reports a concentration in May, while in Robit Bata, the creek was still dry.
FIGURE 6. Mean monthly concentrations for suspended sediment and P fractions over a period (May–November) in 2017 and 2018 for Dangishta and Robit Bata watersheds. (A) sediment concentration (SS); (B) Biological active particulate phosphorus concentration (BAPP); (C) total phosphorus concentration (TP); and (D) dissolved phosphorus concentration (DP).
Despite the higher recorded discharge in Dangishta, sediment and associated total P loss were much lower than in Robit Bata because the sediment concentrations were much smaller (Table 5). The Dangishta on average annual sediment load at the outlet is fifteen times lower than in Robit Bata (Table 5). The TP load was three times lower.
TABLE 5. Monthly discharge, sediment and total P (TP), biological available particulate P (BAPP), and dissolved P (DP) losses during the Dangishta and Robit Bata watersheds during the rain phase and first part of the dry phase in 2017 and 2018 The fraction of biological available particulate P and the fraction of dissolved P of the sum of all phosphorus losses (TP) from the water.
The BAPP flux was found to be over the 2 years in Robit Bata was 1.4 times that observed in Dangishta (Table 5). However, the proportion of potential bioavailability of particulate P of all P (sum of TP and DP) was greater for Dangishta. The BAPP made up 30% of the P lost in Dangishta but only 15% in Robit Bata (Table 5). The DP contributed only 14 and 4% (quotient of the export of DP and all P lost). The DP lost in the discharge in Robit Bata was less than half of Dangishta (Table 5).
Two representative watersheds in the upper Blue Nile basin are compared. Dangishta has many lava intrusion dikes, while in Robit Bata, volcanic dikes were absent. The streamflow was separated into surface and subsurface flow by using the PED model. In Dangishta, the PED model predicted direct runoff from only below 5% of the total discharge at the outlet (Supplementary Table S1.1; Figure 4A). The rest of the flow was baseflow through faults and fissures in the volcanic bedrock but surfaced before reaching the stream. The lava dikes forced the baseflow to the surface. In Robit Bata, the direct flow was one-third of the total discharge originating from approximately 20% of the watershed (Supplementary Table S2). The upper part of the watershed generated saturated excess from the shallow soil profile during the rainstorm. The Robit Bata PED subsurface flows were dominated by interflow, which is similar to reports by Tilahun et al. (2020). The results also agree with Guzman et al. (2017) and Tilahun et al. (2015).
The dikes also prevent the formation of gullies in Dangishta. Surface erosion was, therefore, minimal. The average sediment concentration at the outlet was 0.55 g L−1, equivalent to less than 3 Mg ha⁻1. In Robit Bata, sections of the main channel were unstable, with gully banks slumping in the river. The upper part of the watershed generated saturated excess from the shallow soil profile saturated during the rainstorm. The lower watershed portion had deep soils, and a hillslope aquifer provided interflow through faults. The average sediment concentrations of 10.5 g L−1 in the Robit Bata stream reflected the sediment from riverbank erosion in unstable portions of the stream channel and gully formation. These sediment losses of 70 Mg ha−1a−1 are higher and are common in many watersheds with humid climates (Amare et al., 2019). Gully initiation starts after clearing the forests and continues until the landscape finds a new equilibrium between transport of the excess rainfall to the outlet (Smolska 2007; Nyssen et al., 2008; Tebebu et al., 2010).
In Dangishta, total P in the streamflow at the outlet was on the average of 0.5 mg L−1, commonly seen in developing countries with temperate climates (Sharpley et al., 1992; Boardman et al., 2019). In Robit Bata, the average total P loss was four times greater than Dangishta at almost 2 mg L−1. This ratio is less than the 11 fold difference in sediment concentrations, but like other studies where gully erosion increases the total P load (e.g., (Fox et al., 2016)). The biologically available particulate P (BAPP) concentration in Dangishta was 0.17 and 0.30 mg L−1 in Robit Bata. Thus only different by a factor of two in concentration in the runoff water.
On average, the dissolved P concentration for both watersheds was around 0.1 mg L−1. These concentrations are in the low range of agricultural watersheds in temperate climates worldwide surveyed by Sharley et al. (1992) and similar to the average of 280 watersheds in Minnesota, USA, with an average crop cover 72% crops across sites (Dolph et al., 2019). The relatively low DP concentrations reflect the difference in time that phosphorus fertilizers have been applied between the developing and developed countries. Only during the last 15 years, the Ethiopian government has made phosphorus fertilizers available to farmers to boost agricultural production. Dissolved P concentration during high flow events was near 1 mg L⁻1 when the runoff of fertilized agricultural fields contributed to the discharge at the outlet.
The following sections discuss how the different hydrology and soil loss affect the export of the various forms of phosphorus out of the watershed.
The biological available particulate phosphorus (BAPP) is an important factor in the eutrophication of the shallow Lake Tana, where the wave action suspends the bottom sediment. Consequently, it is of interest to compare available particulate P in the soil (Pca, Table 3) in the watershed with that at the outlet. The biologically available phosphate concentration on the sediment can be calculated as the quotient of the BAPP concentration and the sediment concentration in the water (BAPP/SS).
Comparing watersheds, Figure 7 shows that despite the two times greater BAPP concentration recorded in Robit Bata than in Dangishta (Figure 6), the sediment biological available P (BAPP/SS) was lower than in Dangishta. Averaged over the 2 years, the BAPP/SS was 34 mg kg⁻1 in Dangishta and significantly greater than the 4 mg kg⁻1 in Robit Bata.
FIGURE 7. Boxplots showing the ratio of biological available particulate phosphorus (BAPP) concentration to the suspended sediment (SS) concentration measured during storm events and the soil available P measured in rainfed cropland, eucalyptus and grassland in (A) Dangishta and (B) Robit Bata. BAPP/SS boxplot is based on all storm events during the study period. The land use boxplot is based on 15 soil samples. The box plots with a different letter indicate significant differences following the Kruskal–Wallis one-way analysis at a significance level of 0.05.
The available soil P concentration (Pca, Table 3) is compared with the sediment biological available P (BAPP/SS) in Figure 7A). Available soil P was measured with the Bray method and BAPP with 0.1 M NaOH during storm events. In the USA, the soil data of Kleinman et al. (2001) shows, after removing the outliers, that the 0.1 M NaOH extracted 1.6 times as much P as the Bray method. Within the scatter of the data and taking the conversion factor account of the two extraction methods, the sediment available P concentration (BAPP/SS) in Dangishta was less on the average but in the same order as the grassland and the eucalyptus trees in the valley bottom (Figure 7). It is consistent with the subsurface flow path of the water, which does not transport the fertilized upland soils to the outlet. Hence the sediment and soil concentrations are similar (Huijun et al., 2010). Although in the same order, the sediment concentrations are less than the valley bottom soils because of phosphorus desorption in the interflow with a low P (Weihrauch and Weber 2021).
In Robit Bata, in contrast to Dangishta, the biological available particulate P in the sediment (BAPP/SS) was many times lower than soil available P, Pca, measured for all three land uses (Figure 7B). It is caused by pickup of subsoil low in P by the stream in the gully, offsetting the sediment high in P from the upper part of the watershed. The subsoil in the highlands is still low in P, unlike in developed countries where soil eroded from the bank might contain legacy phosphorus (Dolph et al., 2019).
The difference in source and transport pathways for the sediment and the phosphorus during runoff events can be understood by studying the hysteresis relationship between discharge and concentration of SS, TP, BAPP and DP (Tananaev 2015; Thomas et al., 2016; Moatar et al., 2017; Mhiret et al., 2019; Heathwaite et al., 2021). For the two watersheds, 45 runoff events with a minimum of two observations discharge and concentrations pairs of SS, TP BAPP, DP on the rising limb and two on the falling limb were analyzed. Three types of hysteresis loops were identified: clockwise, counterclockwise, and mixed. Examples are given in Figure 8, and all loops are summarized in the excel files in the supplementary material. The clockwise loops occur when peak concentration arrives before the peak discharge. The counterclockwise arise when the concentration peak occurs after the discharge peak. Mixed loops occur when the concentration peaks are found both before and after the discharge peak (Bowes et al., 2005; Lefrançois et al., 2007; Zegeye et al., 2018; Vale and Dymond 2020).
FIGURE 8. A representative sub-set of storm events hysteresis loops observed for suspended sediment, total, particulate bioavailable, and dissolved phosphorus concentration for the events in rainy season in 2017–2018 study period for the (A) Dangishta and (B) Robit Bata.
There was a significant difference among the watersheds. In Dangishta, 18 loops were analyzed, 75% of the sediment SS-Q loops were clockwise, and 20–30% were counterclockwise (Figure 9A). Although the stream channel is stable, sediment is deposited between storms and settled in the stream. When the discharge increased, the sediment was remobilized, but since sediment was limited, the concentrations were lower during the falling limb as the sediment source has run out. Mixed loops were not observed in Dangishta. In humid-topics of Brazil, Gellis (2013) reported a similar finding that the previous storm events supply sediment to the channel. In-channel sediment became the source of sediment on the rising limb of the next event.
FIGURE 9. cumulative number of hysteresis loops of sediment, total particulate phosphorus, particulate bioavailable phosphorus and dissolved phosphorus concentrations plotted as a function of cumulative effective rainfall for (A–C) Dangishta; (D–F) Robit Bata.
In Robit Bata, 27 storms were analysed. In 2017, 60% of the suspended sediment concentrations and discharge (SS-Q) loops were clockwise loops, and 40% were mixed (Figure 8B). In 2018, 65% had clockwise loops, and the remainder was mixed with one counterclockwise (Figure 9D). The clockwise loops are expected when the sediment source is close to the outlet (Bender et al., 2018). Zegeye et al. (2016) observed the same pattern of clockwise and mixed hysteresis loops at the outlet in a gully with unstable and slipping banks. Since most of the sediment originates from unconsolidated sediment of the slipping bank in the gully, any sediment signal from the upper part is obscured.
In Robit Bata, 70% of the TP-Q loops over the 2 years were clockwise and thus behaved similarly to the sediment SS-Q loops, although mixed SS-Q loops became clockwise TP-Q loops (Figure 9E). The phosphorus (TP-Q) loops were clockwise, such as on August 23, 2017 (Figure 8B). The concentration of P is greater before the peak, and the sediment concentration was both after and before. It means that TP concentration per Gram of sediment increases before the peak of the discharge. During falling limb in mixed loops, the sediment originated from gully with unstable and slipping banks and has a lower TP concentration per Gram of sediment (Figure 7). In Dangishta, the type of phosphorus loops diverged from the kind of sediment loops in several cases. Figure 9B shows in 2017, 60% of TP loops are counterclockwise, and in 2018, 50% of the loops are either counterclockwise or mixed. Less than half of the loops are clockwise. In the cases where the SS-Q loops were clockwise and the phosphorus (TP-Q) were counterclockwise. It indicates that TP concentration per Gram of sediment increases after the peak of the discharge. The sediment is initially high because it is the sediment that was deposited in the steam between storms when the DP concentration was low (Figure 5). The sediment after the peak originates from the land surface with a greater TP concentration per Gram of sediment.
The dissolved P (DP-Q) loops behaved the same in both watersheds but differed from the SS, TP and BAPP (Figure 8). In Dangishta, 65% of the dissolved phosphorus (DP-Q loops) are counterclockwise in the 2 years (Figure 9C). In Robit Bata, counterclockwise loops increased above that for sediment and TP (Figure 9F). The DP is a mobile fraction and can be transported via surface runoff, interflow, and baseflow (Evans and Davies 1998). At the onset of the rain phase, the DP followed a clockwise response predominantly similar to the other P fractions. However, as the season progressed, a perched groundwater table developed and the upland became connected with the downhill area. The contribution of interflow and baseflow to streamflow became the major portion of the discharge at the outlet (Figure 4). Higher DP contributions that entered streamflow via sub-surface processes resulted in counterclockwise loops. Fortesa et al. (2020) noted that when the pathways in the watershed become connected, the hysteresis loops become counterclockwise.
Similar to an earlier study (Guzman et al., 2013), the rainy monsoon phase was divided into intervals of cumulative effective rainfall to assess the transport of sediment, particulate and dissolved fractions of P as a function of discharge (Figure 10). Daily concentrations of sediment and P were plotted as a function of average daily discharge. Although some trends were the same (i.e., high sediment concentration at low flows at the beginning of the rainy period), the regression coefficients were very low, unlike Guzman et al. (2013) (Figures 10A,E). In the watersheds analyzed by Guzman et al. (2013), the runoff was generated by overland flow, and gully erosion was minor. In this study, the decreasing rate of rill erosion during the rain phase was responsible for the decreasing sediment concentrations at the outlet. In Dangishta, surface runoff was minimal, and the daily concentration decreased with the increase in interflow and baseflow as the rain phase progressed (Figure 4). In Robit Bata, dilution by interflow occurred for the smaller storms. Still, the maximum sediment concentration remained the same during the growing season due to the pickup of sediment from the gully (Figure 10E).
FIGURE 10. Daily flow weighted mean concentration of SS, TP, BAPP, and DP during a rain phase of 2017 and 2018, as a function of discharge for increments of cumulative effective of rainfall (Rce). Panels (A–D) depict the relationships for Dangishta, and Panels (E–H) are for Robit Bata. The regression lines have the same color as the symbols.
The daily total P concentration behaved similarly to the sediment concentration (Figures 10B,F). With the progression of the rain phase, the TP concentrations decreased for the same amount of discharge. However, the R2 coefficients for the relationship between the total P concentration and discharge were poor similar to the sediment. Curious was that the BAPP concentration and discharge had a decent R2 for cumulative effective rainfall intervals of 450–800 mm in the Robit Bata watershed (Figure 10G).
Remarkably, the dissolved P concentration and discharge were linearly related in the Robit Bata watershed with R2 > 0.55 for 300 m or more cumulative effective rainfall (Figure 10G). In Dangishta, the relationship was linear for the cumulative rainfall interval between 450 and 800 mm (Figure 10D). Theoretically, this linear relationship can be obtained by assuming that the interflow concentration is small compared to the storm flow and the interflow is constant during each cumulative rainfall interval. These are reasonable assumptions since the DP concentrations are the same as the groundwater in the valley bottom, and as Figures 10D,G show, the intercept with the x-axis is small.
Appropriate landscape measures need to be implemented to reduce P export in these watersheds to improve downstream water quality, such as Lake Tana, where water hyacinths have rapidly expanded. Measures are proposed to decrease sediment loss to improve the water quality of the lake (Ayele et al., 2016; Bartley et al., 2020). However, this research shows that reducing sediment loss, actually might decrease lake water quality. The reasoning is as follows. Recent investigations in the relatively shallow Lake Tana found a positive correlation between the BAPP sediment concentration and the dissolved P concentration in the lake water (Kebedew et al., 2020). This study shows that in watersheds with gullies (e.g., Robit Bata), the BAPP concentration per unit sediment exported is much lower than watersheds without gullies (e.g., Dangishta). Thus, stopping the gully sediment from entering the lake will increase the BAPP sediment concentration of the deposited soil on the lake bottom. Hence, the mixing increases the dissolved phosphorus concentration in the lake water, promoting water hyacinth growth.
Another management practice advocated in the USA and other developed countries consists of buffer strips near the stream to remove P from the runoff water before entering the stream (Georgakakos et al., 2018; Young et al., 2019). In the (sub) humid Ethiopian highlands, the near stream area is saturated after the middle of the rain phase. They are, therefore, unsuitable for growing crops and are used for grazing animals and eucalyptus and thereby act as a natural buffer. So near stream buffer strips exist already. Conversely, drainage of these periodically saturated near stream areas for crop cultivation is expected to enhance the transport of P to the stream significantly.
Finally, a practice implemented in the Ethiopian Highlands to stop soil loss and associated particulate P are 50–60 cm deep infiltration furrows with bunds (Dagnew et al., 2017). It has not been successful in (sub) humid highlands due to the lack of maintenance (Gebregergs et al., 2021; Mhiret et al., 2021). The current trend of replacing cropland with eucalyptus trees might effectively decrease P loads since increased acreage of eucalyptus trees reduced the runoff volume by delaying the start of runoff after the dry phase (Mhiret et al., 2019).
Consequently, designing landscape interventions in the humid tropics will require further study since the results of our research lead to the conclusion that commonly implemented practices need further adaptation for the (sub)humid highlands.
One of the impediments in reducing P loss in developing countries is the lack of experimental studies on P transport. This study was conducted in two contrasting watersheds (Dangishta and Robit Bata) in the Ethiopian highland. The first watershed was the 57 km2 Dangishta, with many lava intrusion dikes, forcing interflow to the surface and preventing the formation of gullies. The average annual rainfall is 1745 mm, and 65% of the area was cropped. The second watershed was the 9 km2 Robit Bata with 1,420 mm average annual precipitation and 87% of the land cropped. In Robit Bata, sections of the mainstream were unstable, with banks slumping in the river.
Three factors in these tropical (sub) humid highland watersheds affected the phosphorus loss compared with temperate watersheds: climate, geology and past application of fertilizers. The annual rainfall in (sub) humid climates is over 1,200 mm, of which around 80% falls in the 4 months. The high rainfall greatly enhanced the transport of P from the watershed compared to the most temperate climates, where annual rainfall often does not exceed 1,200 mm and is spread throughout the year. In addition, underlying volcanic-derived geology greatly affected the P losses. It varies among the watersheds. In Dangishta, 95% of the estimated stream discharge reached the stream through faults in the volcanic bedrock, surfacing at the lava dikes. Consequently, in Dangishta, the total erosion and particulate P losses were relatively small and comparable to watersheds in temperate regions with less rainfall. In the Robit Bata with active gullies in the main channel, the sediment losses were 11 times and total P losses four times greater than in Dangishta. Only under exceptional circumstances, these concentrations and loads occur in temperate climates. Dissolved P losses in both watersheds were in the low range found in western countries with a temperate climate because phosphorus fertilizers have been applied recently. In the past, shifting cultivation was used to maintain fertility, but the increasing population density made it unfeasible.
Based on findings that legacy P negatively affects the stream water quality in temperate climates where agricultural land has been cultivated for centuries, phosphorus export is expected to increase in these tropical watersheds where fertilizer application has just started. Finding ways to reduce phosphorus exports is therefore critical to reverse the water quality deterioration in Lake Tana. Our study aids in prioritizing watersheds for treatment. Further study is required on what practices will be most effective.
The raw data supporting the conclusion of this article will be made available by the authors, without undue reservation.
All authors participated fully in the research. FS and AB collected the data in the field and analyzed the samples. FS wrote the first draft, and the subsequent versions were a cooperative effort of FS, PS, ST and TS. PS and ST obtained funding.
The study was made possible through funds provided by the U.S. Agency for International Development under the Feed the Future Evaluation of the Relationship between Sustainably Intensified Production Systems and Farm Family Nutrition (SIPS-IN) project (AID-OAA-L-14-00006) with additional support from the Feed the Future Innovation Lab for Small Scale Irrigation (contract no. AID-OAA-A-13-0055). Furthermore, the authors received funding from the CGIAR Research Program on Water, Land and Ecosystems (WLE) supported by CGIAR Fund Donors. The paper contents are the authors’ responsibility and do not necessarily reflect the views of USAID or the United States government.
The authors declare that the research was conducted in the absence of any commercial or financial relationships that could be construed as a potential conflict of interest.
All claims expressed in this article are solely those of the authors and do not necessarily represent those of their affiliated organizations, or those of the publisher, the editors, and the reviewers. Any product that may be evaluated in this article, or claim that may be made by its manufacturer, is not guaranteed or endorsed by the publisher.
This paper is dedicated to Ato Girma, who was in charge of the Dangishta data collection since its start in 2014. He passed away in 2021. This research would not have been completed without his dedicated work. He is greatly missed.
The Supplementary Material for this article can be found online at: https://www.frontiersin.org/articles/10.3389/feart.2021.762703/full#supplementary-material
Abidela Hussein, M., Muche, H., Schmitter, P., Nakawuka, P., Tilahun, S. A., Langan, S., et al. (2019). Deep Tillage Improves Degraded Soils in the (Sub) Humid Ethiopian Highlands. Land 8 (11), 159. doi:10.3390/land8110159
Adem, A. A., Addis, G. G., Aynalem, D. W., Tilahun, S. A., Mekuria, W., Azeze, M., et al. (2020). Hydrogeology of Volcanic Highlands Affects Prioritization of Land Management Practices. Water 12 (10), 2702. doi:10.3390/w12102702
Alemu, M. L., Geset, M., Mosa, H. M., Zemale, F. A., Moges, M. A., Giri, S. K., et al. (2017). Spatial and Temporal Trends of Recent Dissolved Phosphorus Concentrations in Lake Tana and its Four Main Tributaries. Land. Degrad. Dev. 28 (5), 1742–1751. doi:10.1002/ldr.2705
Akale, A. T., Dagnew, D. C., Belete, M. A., Tilahun, S. A., Mekuria, W., and Steenhuis, T. S. (2017). Impact of Soil Depth and Topography on the Effectiveness of Conservation Practices on Discharge and Soil Loss in the Ethiopian Highlands. Land 6 (4), 78.
Amare, D., Wondie, M., Mekuria, W., and Darr, D. (2019). Agroforestry of Smallholder Farmers in Ethiopia: Practices and Benefits. Small-scale For. 18 (1), 39–56.
Ayele, G. K., Gessess, A. A., Addisie, M. B., Tilahun, S. A., Tebebu, T. Y., Tenessa, D. B., et al. (2016). A Biophysical and Economic Assessment of a Community-Based Rehabilitated Gully in the Ethiopian Highlands. Land Degrad. Develop. 27, 270–280. doi:10.1002/ldr.2425
Baird, R. B., Eaton, A. D., Rice, E. W., and Bridgewater, L. (2017). Standard Methods for the Examination of Water and Wastewater. Washington, DC: American Public Health Association.
Bartley, R., Poesen, J., Wilkinson, S., and Vanmaercke, M. (2020). A Review of the Magnitude and Response Times for Sediment Yield Reductions Following the Rehabilitation of Gullied Landscapes. Earth Surf. Process. Landforms 45, 3250–3279. doi:10.1002/esp.4963
Bender, M. A., dos Santos, D. R., Tiecher, T., Minella, J. P. G., de Barros, C. A. P., and Ramon, R. (2018). Phosphorus Dynamics during Storm Events in a Subtropical Rural Catchment in Southern Brazil. Agric. Ecosyst. Environ. 261, 93–102. doi:10.1016/j.agee.2018.04.004
Bennett, E. M., Carpenter, S. R., and Caraco, N. F. (2001). Human Impact on Erodable Phosphorus and Eutrophication: A Global Perspective. Bioscience 51 (3), 227–234. doi:10.1641/0006-3568(2001)051
Beretta, A. N., Silbermann, A. V., Paladino, L., Torres, D., Bassahun, D., Musselli, R., et al. (2014). Soil Texture Analyses Using a Hydrometer: Modification of the Bouyoucos Method. Cienc. Inv. Agr. 41 (2), 25–26. doi:10.4067/S0718-16202014000200013
Boardman, E., Danesh-Yazdi, M., Foufoula-Georgiou, E., Dolph, C. L., and Finlay, J. C. (2019). Fertilizer, Landscape Features and Climate Regulate Phosphorus Retention and River export in Diverse Midwestern Watersheds. Biogeochemistry 146 (3), 293–309. doi:10.1007/s10533-019-00623-z
Bowes, M. J., House, W. A., Hodgkinson, R. A., and Leach, D. V. (2005). Phosphorus-discharge Hysteresis during Storm Events along a River Catchment: the River Swale, UK. Water Res. 39 (5), 751–762. doi:10.1016/j.watres.2004.11.027
Bray, R. H., and Kurtz, L. T. (1945). Determination of Total, Organic, and Available Forms of Phosphorus in Soils. Soil Sci. 59 (1), 39–46. doi:10.1097/00010694-194501000-00006
Chow, M., Huang, J.-C., and Shiah, F.-K. (2017). Phosphorus Dynamics along River Continuum during Typhoon Storm Events. Water 9 (7), 537. doi:10.3390/w9070537
Collick, A. S., EastonAshagrie, Z. M. T., Biruk, B., Tilahun, S. A., Adgo, E., Awulachew, S. B., et al. (2009). A Simple Semi-distributed Water Balance Model for the Ethiopian highlands. Hydrological Process. 23, 3718–3727. doi:10.1002/hyp.7517
Collins, A. L., Zhang, Y. S., Duethmann, D., Walling, D. E., and Black, K. S. (2013). Using a Novel Tracing-Tracking Framework to Source fine-grained Sediment Loss to Watercourses at Sub-catchment Scale. Hydrol. Process. 27 (6), 959–974. doi:10.1002/hyp.9652
Dagnew, D. C., Guzman, C. D., Akale, A. T., Tebebu, T. Y., Zegeye, A. D., Mekuria, W., et al. (2017). Effects of Land Use on Catchment Runoff and Soil Loss in the Sub-humid Ethiopian highlands. Ecohydrology & Hydrobiology 17 (4), 274–282. doi:10.1016/j.ecohyd.2017.07.004
Dersseh, M. G., Kibret, A. A., Tilahun, S. A., Worqlul, A. W., Moges, M. A., Dagnew, D. C., et al. (2019). Potential of Water Hyacinth Infestation on lake Tana, ethiopia: A Prediction Using a Gis-Based Multi-Criteria Technique. Water 11 (9), 1921. doi:10.3390/w11091921
Dolph, C. L., Boardman, E., Danesh‐Yazdi, M., Finlay, J. C., Hansen, A. T., Baker, A. C., et al. (2019). Phosphorus Transport in Intensively Managed Watersheds. Water Resour. Res. 55 (11), 9148–9172. doi:10.1029/2018WR024009
Donn, M. J., Barron, O. V., and Barr, A. D. (2012). Identification of Phosphorus export from Low-Runoff Yielding Areas Using Combined Application of High Frequency Water Quality Data and MODHMS Modelling. Sci. Total Environ. 426, 264–271. doi:10.1016/j.scitotenv.2012.03.021
Ellison, M. E., and Brett, M. T. (2006). Particulate Phosphorus Bioavailability as a Function of Stream Flow and Land Cover. Water Res. 40 (6), 1258–1268. doi:10.1016/j.watres.2006.01.016
Ezzati, G., Fenton, O., Healy, M. G., Christianson, L., Feyereisen, G. W., Thornton, S., et al. (2020). Impact of P Inputs on Source-Sink P Dynamics of Sediment along an Agricultural Ditch Network. J. Environ. Manage. 257, 109988. doi:10.1016/j.jenvman.2019.109988
Fortesa, J., Latron, J., García-Comendador, J., Company, J., and Estrany, J. (2020). Runoff and Soil Moisture as Driving Factors in Suspended Sediment Transport of a Small Mid-mountain Mediterranean Catchment. Geomorphology 368, 107349. doi:10.1016/j.geomorph.2020.107349
Fox, G. A., Purvis, R. A., and Penn, C. J. (2016). Streambanks: A Net Source of Sediment and Phosphorus to Streams and Rivers. J. Environ. Manage. 181, 602–614. doi:10.1016/j.jenvman.2016.06.071
Gao, Y., Zhu, B., Wang, T., Tang, J.-l., Zhou, P., and Miao, C.-y. (2010). Bioavailable Phosphorus Transport from a Hillslope Cropland of Purple Soil under Natural and Simulated Rainfall. Environ. Monit. Assess. 171 (1-4), 539–550. doi:10.1007/s10661-009-1298-6
Gebregergs, T., Teka, K., Taye, G., Gidey, E., and Dikinya, O. (2021). Status and Challenges of Integrated Watershed Management Practices After-Project Phased-Out in Eastern Tigray, Ethiopia. Model. Earth Syst. Environ. 7, 1–7. doi:10.1007/s40808-021-01108-5
Gellis, A. C. (2013). Factors Influencing Storm-Generated Suspended-Sediment Concentrations and Loads in Four Basins of Contrasting Land Use, Humid-Tropical Puerto Rico. Catena 104: 39–57. doi:10.1016/j.catena.2012.10.018
Georgakakos, C. B., Morris, C. K., and Walter, M. T. (2018). Challenges and Opportunities with On-Farm Research: Total and Soluble Reactive Stream Phosphorus before and after Implementation of a Cattle-Exclusion, Riparian Buffer. Front. Environ. Sci. 6, 1–12. doi:10.3389/fenvs.2018.00071
Gikuma-Njuru, P., Rutagemwa, D., Mugidde, R., Hecky, R., Mwebaza-Ndawula, L., Mwirigi, P., et al. (2005). Eutrophication of the Lake Victoria Ecosystem. Available at: http://195.202.82.11:8080/jspui/bitstream/123456789/167/1/_Lake%20Victoria%20Environme.pdf (August 5, 2021).
Grum, B., Assefa, D., Hessel, R., Woldearegay, K., Kessler, A., Ritsema, C., et al. (2017). Effect of In Situ Water Harvesting Techniques on Soil and Nutrient Losses in Semi-Arid Northern Ethiopia. Land. Degrad. Dev. 28 (3), 1016–1027.
Guzman, C. D., Tilahun, S. A., Dagnew, D. C., Zegeye, A. D., Tebebu, T. Y., Yitaferu, B., et al. (2017a). Modeling Sediment Concentration and Discharge Variations in a Small Ethiopian Watershed with Contributions from an Unpaved Road. J. Hydrol. Hydromechanics. J. Hydrol. Hydromechanics 65 (1), 1–17. doi:10.1515/johh-2016-0051
Guzman, C. D., Tilahun, S. A., Zegeye, A. D., and Steenhuis, T. S. (2013). Suspended Sediment Concentration-Discharge Relationships in the (Sub-) Humid Ethiopian highlands. Hydrol. Earth Syst. Sci. 17 (3), 1067–1077. doi:10.5194/hess-17-1067-2013
Guzman, C. D., Zimale, F. A., Tebebu, T. Y., Bayabil, H. K., Tilahun, S. A., Yitaferu, B., et al. (2017b). Modeling Discharge and Sediment Concentrations after Landscape Interventions in a Humid Monsoon Climate: The Anjeni Watershed in the highlands of Ethiopia. Hydrol. Process. 31, 1239–1257. doi:10.1002/hyp.11092
Haregeweyn, N., Tsunekawa, A., Poesen, J., Tsubo, M., Meshesha, D. T., Fenta, A. A., et al. (2017). Comprehensive Assessment of Soil Erosion Risk for Better Land Use Planning in River Basins: Case Study of the Upper Blue Nile River. Sci. Total Environ. 574, 95–108. doi:10.1016/j.scitotenv.2016.09.019
Heathwaite, A. L., and Bieroza, M. (2021). Fingerprinting Hydrological and Biogeochemical Drivers of Freshwater Quality. Hydrological Process. 35 (1), e13973. doi:10.1002/hyp.13973
Heathwaite, A. L. (2010). Multiple Stressors on Water Availability at Global to Catchment Scales: Understanding Human Impact on Nutrient Cycles to Protect Water Quality and Water Availability in the Long Term. Freshwat. Biol. 55 (s1), 241–257. doi:10.1111/j.1365-2427.2009.02368.x
Huijun, H., Zhigang, Y., Qingzheng, Y., Hongtao, C., and Tiezhu, M. (2010). The Hydrological Regime and Particulate Size Control Phosphorus Form in the Suspended Solid Fraction in the Dammed Huanghe (Yellow River). Hydrobiologia 638 (1), 203–211. doi:10.1007/s10750-009-0041-1
Kebedew, M. G., Kibret, A. A., Tilahun, S. A., Belete, M. A., Zimale, F. A., and Steenhuis, T. S. (2020). The Relationship of Lake Morphometry and Phosphorus Dynamics of a Tropical Highland Lake: Lake Tana, Ethiopia. Water 12, 2243. doi:10.3390/w12082243
Lawler, D. M., Petts, G. E., Foster, I. D. L., and Harper, S. (2006). Turbidity Dynamics during spring Storm Events in an Urban Headwater River System: The Upper Tame, West Midlands, UK. Sci. Total Environ. 360 (1-3), 109–126. doi:10.1016/j.scitotenv.2005.08.032
Lefrançois, J., Grimaldi, C., Gascuel-Odoux, C., and Gilliet, N. (2007). Suspended Sediment and Discharge Relationships to Identify Bank Degradation as a Main Sediment Source on Small Agricultural Catchments. Hydrol. Process. 21 (21), 2923–2933. doi:10.1002/hyp.6509
Lemann, T., Zeleke, G., Amsler, C., Giovanoli, L., Suter, H., and Roth, V. (2016). Modelling the Effect of Soil and Water Conservation on Discharge and Sediment Yield in the Upper Blue Nile basin, Ethiopia. Appl. Geogr. 73, 89–101. doi:10.1016/j.apgeog.2016.06.008
Li, H.-M., Tang, H.-J., Shi, X.-Y., Zhang, C.-S., and Wang, X.-L. (2014). Increased Nutrient Loads from the Changjiang (Yangtze) River Have Led to Increased Harmful Algal Blooms. Harmful Algae 39, 92–101. doi:10.1016/j.hal.2014.07.002
Liu, B. M., Collick, A. S., Zeleke, G., Adgo, E., Easton, Z. M., and Steenhuis, T. S. (2008). Rainfall-Discharge Relationships for a Monsoonal Climate in the Ethiopian Highlands. Hydrol. Process. 22 (7), 1059–1067.
Lloyd, C. E. M., Freer, J. E., Johnes, P. J., and Collins, A. L. (2016). Technical Note: Testing an Improved index for Analysing Storm Discharge-Concentration Hysteresis. Hydrol. Earth Syst. Sci. 20 (2), 625–632. doi:10.5194/hess-20-625-2016
Macintosh, K. A., Mayer, B. K., McDowell, R. W., Powers, S. M., Baker, L. A., Boyer, T. H., et al. (2018). Managing Diffuse Phosphorus at the Source versus at the Sink. Environ. Sci. Technol. 52 (21), 11995–12009. doi:10.1021/acs.est.8b01143
Mekonnen, M. M., and Hoekstra, A. Y. (2018). Global Anthropogenic Phosphorus Loads to Freshwater and Associated Grey Water Footprints and Water Pollution Levels: A High‐Resolution Global Study. Water Resour. Res. 54 (1), 345–358. doi:10.1002/2017WR020448
Mendoza, G., and Steenhuis, T. S. (2002). Determination of Hydraulic Behavior of Hillsides with a Hillslope Infiltrometer. Soil Sci. Soc. Am. J. 66 (5), 1501–1504. doi:10.2136/sssaj2002.1501
Mhiret, D. A., Dagnew, D. C., Alemie, T. C., Guzman, C. D., Tilahun, S. A., Zaitchik, B. F., et al. (2019). Impact of Soil Conservation and Eucalyptus on Hydrology and Soil Loss in the Ethiopian highlands. Water 11 (11), 2299. doi:10.3390/w11112299
Mhiret, D. A., Dagnew, D. C., Guzman, C. D., Alemie, T. C., Zegeye, A. D., Tebebu, T. Y., et al. (2020). A Nine-Year Study on the Benefits and Risks of Soil and Water Conservation Practices in the Humid highlands of Ethiopia: The Debre Mawi Watershed. J. Environ. Manage. 270, 110885. doi:10.1016/j.jenvman.2020.110885
Moatar, F., Abbott, B. W., Minaudo, C., Curie, F., and Pinay, G. (2017). Elemental Properties, Hydrology, and Biology Interact to Shape Concentration‐discharge Curves for Carbon, Nutrients, Sediment, and Major Ions. Water Resour. Res. 53 (2), 1270–1287. doi:10.1002/2016WR019635
Moges, M. A., Schmitter, P., Tilahun, S. A., Langan, S., Dagnew, D. C., Akale, A. T., et al. (2017). Suitability of Watershed Models to Predict Distributed Hydrologic Response in the Awramba Watershed in Lake Tana Basin. Land Degrad. Develop. 28 (4), 1386–1397. doi:10.1002/ldr.2608
Moges, M. A., Tilahun, S. A., Ayana, E. K., Moges, M. M., Gabye, N., Giri, S., et al. (2016). Non-Point Source Pollution of Dissolved Phosphorus in the Ethiopian Highlands: The Awramba Watershed Near Lake Tana. Clean. Soil Air Water 44, 703–709. doi:10.1002/clen.201500131
Moges, M. A., Zemale, F. A., Alemu, M. L., Ayele, G. K., Dagnew, D. C., Tilahun, S. A., et al. (2016). Sediment Concentration Rating Curves for a Monsoonal Climate: Upper Blue Nile. Soil 2 (3), 337–349. doi:10.5194/soil-2-337-2016
Mulualem, T., Adgo, E., Meshesha, D. T., Tsunekawa, A., Haregeweyn, N., Tsubo, M., et al. (2021). Exploring the Variability of Soil Nutrient Outflows as Influenced by Land Use and Management Practices in Contrasting Agro-Ecological Environments. Sci. Total Environ. 786, 147450.
Murphy, J., and Riley, J. P. (1962). A Modified Single Solution Method for the Determination of Phosphate in Natural Waters. Analytica Chim. Acta 27, 31–36. doi:10.1016/S0003-2670(00)88444-5
Nigate, F., Van Camp, M., Kebede, S., and Walraevens, K. (2016). Hydrologic Interconnection between the Volcanic Aquifer and Springs, Lake Tana basin on the Upper Blue Nile. J. Afr. Earth Sci. 121, 154–167. doi:10.1016/j.jafrearsci.2016.05.015
Nyenje, P. M., Foppen, J. W., Uhlenbrook, S., Kulabako, R., and Muwanga, A. (2010). Eutrophication and Nutrient Release in Urban Areas of Sub-saharan Africa - A Review. Sci. Total Environ. 408 (3), 447–455. doi:10.1016/j.scitotenv.2009.10.020
Nyssen, J., Poesen, J., Moeyersons, J., Haile, M., and Deckers, J. (2008). Dynamics of Soil Erosion Rates and Controlling Factors in the Northern Ethiopian Highlands - towards a Sediment Budget. Earth Surf. Process. Landforms 33 (5), 695–711. doi:10.1002/esp.1569
Ochoa-Tocachi, B. F., Alemie, T. C., Guzman, C. D., Tilahun, S. A., Zimale, F. A., Buytaert, W., et al. (2018). Sensitivity Analysis of the Parameter-Efficient Distributed (PED) Model for Discharge and Sediment Concentration Estimation in Degraded Humid Landscapes. Land Degrad. Dev. 30, 151–165. doi:10.1002/ldr.3202
Rabalais, N. N., Díaz, R. J., Levin, L. A., Turner, R. E., Gilbert, D., and Zhang, J. (2010). Dynamics and Distribution of Natural and Human-Caused Hypoxia. Biogeosciences 7(2): 585–619. doi:10.5194/bg-7-585-2010
Reynolds, C. S., and Davies, P. S. (2001). Sources and Bioavailability of Phosphorus Fractions in Freshwaters: a British Perspective. Biol. Rev. 76(1): 27–64. doi:10.1017/S1464793100005625
Ruttenberg, K. C., and Sulak, D. J. (2011). Sorption and Desorption of Dissolved Organic Phosphorus onto Iron (Oxyhydr)oxides in Seawater. Geochimica et Cosmochimica Acta 75 (15), 4095–4112. doi:10.1016/j.gca.2010.10.033
Scavia, D., David Allan, J., Arend, K. K., Bartell, S., Beletsky, D., Bosch, N. S., et al. (2014). Assessing and Addressing the Re-eutrophication of Lake Erie: Central basin Hypoxia. J. Great Lakes Res. 40 (2), 226–246. doi:10.1016/j.jglr.2014.02.004
Sharpley, A. N., Smith, S. J., Jones, O. R., Berg, W. A., and Coleman, G. A. (1992). The Transport of Bioavailable Phosphorus in Agricultural Runoff. J. Environ. Qual. 21 (1), 30–35. doi:10.2134/jeq1992.00472425002100010003x
Smolska, E. (2007). Development of Gullies and Sediment Fans in Last-Glacial Areas on the Example of the Suwałki Lakeland (NE Poland). Catena 71 (1), 122–131. doi:10.1016/j.catena.2006.10.009
Steenhuis, T. S., Collick, A. S., Easton, Z. M., Leggesse, E. S., Bayabil, H. K., White, E. D., et al. (2009). Predicting Discharge and Sediment for the Abay (Blue Nile) with a Simple Model. Hydrol. Process. 23 (26), a–n. doi:10.1002/hyp.7513
Steenhuis, T. S., Tilahun, S. A., Elkamil, M., Atanaw, F., Worqlul, A., Ayana, E. K., et al. (2013). “Simulating Discharge and Sediment Concentrations in the Increasingly Degrading Blue Nile Basin,” in Proceedings conference on science and technology towards the Development of East Africa, Bahir Dar, Ethiopia, July 2013.
Tananaev, N. I. (2015). Hysteresis Effects of Suspended Sediment Transport in Relation to Geomorphic Conditions and Dominant Sediment Sources in Medium and Large Rivers of the Russian Arctic. Nord. Hydrol. 46 (2), 232–243. doi:10.2166/nh.2013.199
Tebebu, T. Y., Abiy, A. Z., Zegeye, A. D., Dahlke, H. E., Easton, Z. M., Tilahun, S. A., et al. (2010). Surface and Subsurface Flow Effect on Permanent Gully Formation and upland Erosion Near Lake Tana in the Northern highlands of Ethiopia. Hydrol. Earth Syst. Sci. 14 (11), 2207–2217. doi:10.5194/hess-14-2207-2010
Tesemma, Z. K., Mohamed, Y. A., and Steenhuis, T. S. (2010). Trends in Rainfall and Runoff in the Blue Nile Basin: 1964-2003. Hydrol. Process. 24, 3747–3758. doi:10.1002/hyp.7893
Thomas, Z., Abbott, B. W., Troccaz, O., Baudry, J., and Pinay, G. (2016). Proximate and Ultimate Controls on Carbon and Nutrient Dynamics of Small Agricultural Catchments. Biogeosciences 13 (6), 1863–1875. doi:10.5194/bg-13-1863-2016
Seifu Admassu Tilahun, S. A., Rajith Mukundan, R., Bezawit A. Demisse, B. A., Tegenu A. Engda, T. A., Christian D. Guzman, C. D., Birara C. Tarakegn, B. C., et al. (2013). A Saturation Excess Erosion Model. Trans. ASABE 56 (2), 681–695. doi:10.13031/2013.42675
Tilahun, S. A., Guzman, C. D., Zegeye, A. D., Dagnew, D. C., Collick, A. S., Yitaferu, B., et al. (2015). Distributed Discharge and Sediment Concentration Predictions in the Sub-humid Ethiopian highlands: the Debre Mawi Watershed. Hydrol. Process. 29 (7), 1817–1828. doi:10.1002/hyp.10298
Tilahun, S. A., Yilak, D. L., Schmitter, P., Zimale, F. A., Langan, S., Barron, J., et al. (2020). Establishing Irrigation Potential of a hillside Aquifer in the African highlands. Hydrological Process. 34 (8), 1741–1753. doi:10.1002/hyp.13659
Toohey, R. C., Boll, J., Brooks, E. S., and Jones, J. R. (2018). Effects of Land Use on Soil Properties and Hydrological Processes at the point, Plot, and Catchment Scale in Volcanic Soils Near Turrialba, Costa Rica. Geoderma 315, 138–148. doi:10.1016/j.geoderma.2017.11.044
Turner, R. E., Rabalais, N. N., and Justic, D. (2006). Predicting Summer Hypoxia in the Northern Gulf of Mexico: Riverine N, P, and Si Loading. Mar. Pollut. Bull. 52 (2), 139–148. doi:10.1016/j.marpolbul.2005.08.012
Uusitalo, R., Turtola, E., Kauppila, T., and Lilja, T. (2001). Particulate Phosphorus and Sediment in Surface Runoff and Drainflow from Clayey Soils. J. Environ. Qual. 30 (2), 589–595. doi:10.2134/jeq2001.302589x
Vale, S. S., and Dymond, J. R. (2020). Interpreting Nested Storm Event Suspended Sediment‐discharge Hysteresis Relationships at Large Catchment Scales. Hydrological Process. 34 (2), 420–440. doi:10.1002/hyp.13595
Walker, D., Parkin, G., Schmitter, P., Gowing, J., Haile, A. T., and Yimam, A. Y. 2018). Insights from a Multi-Method Recharge Estimation Comparison Study. Groundwater, 57, 245, 258. doi:10.1111/gwat.12801
Walkley, A., and Black, I. A. (1934). An Examination of the Degtjareff Method for Determining Soil Organic Matter, and a Proposed Modification of the Chromic Acid Titration Method. Soil Sci. 37 (1), 29–38. doi:10.1097/00010694-193401000-00003
Watson, S. B., Miller, C., Arhonditsis, G., Boyer, G. L., Carmichael, W., Charlton, M. N., et al. (2016). The Re-eutrophication of Lake Erie: Harmful Algal Blooms and Hypoxia. Harmful Algae 56, 44–66. doi:10.1016/j.hal.2016.04.010
Weihrauch, C., and Weber, C. J. (2021). The Enrichment of Phosphorus in Floodplain Subsoils - A Case Study from the Antrift Catchment (Hesse, Germany). Geoderma 385, 114853. doi:10.1016/j.geoderma.2020.114853
Yimam, A. Y., Bekele, A. M., Nakawuka, P., Schmitter, P., and Tilahun, S. A. (2019). “Rainfall-Runoff Process and Groundwater Recharge in the Upper Blue Nile Basin: The Case of Dangishta Watershed,” in International Conference on Advances of Science and Technology, Bahir Dar, Ethiopia, 08 March 2019 (Berlin/Heidelberg, Germany: Springer), 536–549. doi:10.1007/978-3-030-15357-1_43
Young, E. O., Ross, D. S., and Jaynes, D. B. (2019). Editorial: Riparian Buffer Nutrient Dynamics and Water Quality. Front. Environ. Sci. 7, 1–2. doi:10.3389/fenvs.2019.00076
Zegeye, A. D., Langendoen, E. J., Stoof, C. R., Tilahun, S. A., Dagnew, D. C., Zimale, F. A., et al. (2016). Morphological Dynamics of Gully Systems in the Subhumid Ethiopian Highlands: the Debre Mawi Watershed. SOIL 2 (3): 443–458. doi:10.5194/soil-2-443-2016
Zegeye, A. D., Langendoen, E. J., Guzman, C. D., Dagnew, D. C., Amare, S. D., Tilahun, S. A., et al. (2018). Gullies, a Critical Link in Landscape Soil Loss: A Case Study in the Subhumid highlands of Ethiopia. Land Degrad. Dev. 29 (4), 1222–1232. doi:10.1002/ldr.2875
Zhao, Z., Shen, Y., Jiang, R., and Wang, Q. (2020). Rock Outcrops Change Infiltrability and Water Flow Behavior in a Karst Soil. Vadose Zone J. 19 (1), e20002. doi:10.1002/vzj2.20002
Zimale, F. A., Moges, M. A., Alemu, M. L., Ayana, E. K., Demissie, S. S., Tilahun, S. A., et al. (2018). Budgeting Suspended Sediment Fluxes in Tropical Monsoonal Watersheds with Limited Data: the Lake Tana basin. J. Hydrol. Hydromech. 66(1), 65–78. doi:10.1515/johh-2017-0039
Keywords: baseflow separation, highlands, interflow, phosphorus, runoff, soil loss, Sub-Saharan Africa, watershed
Citation: Sishu FK, Bekele AM, Schmitter P, Tilahun SA and Steenhuis TS (2021) Phosphorus Export From Two Contrasting Rural Watersheds in the (Sub) Humid Ethiopian Highlands. Front. Earth Sci. 9:762703. doi: 10.3389/feart.2021.762703
Received: 22 August 2021; Accepted: 19 November 2021;
Published: 16 December 2021.
Edited by:
Jun Niu, China Agricultural University, ChinaReviewed by:
Pingping Luo, Chang’an University, ChinaCopyright © 2021 Sishu, Bekele, Schmitter, Tilahun and Steenhuis. This is an open-access article distributed under the terms of the Creative Commons Attribution License (CC BY). The use, distribution or reproduction in other forums is permitted, provided the original author(s) and the copyright owner(s) are credited and that the original publication in this journal is cited, in accordance with accepted academic practice. No use, distribution or reproduction is permitted which does not comply with these terms.
*Correspondence: Tammo S. Steenhuis, dHNzMUBjb3JuZWxsLmVkdQ==
Disclaimer: All claims expressed in this article are solely those of the authors and do not necessarily represent those of their affiliated organizations, or those of the publisher, the editors and the reviewers. Any product that may be evaluated in this article or claim that may be made by its manufacturer is not guaranteed or endorsed by the publisher.
Research integrity at Frontiers
Learn more about the work of our research integrity team to safeguard the quality of each article we publish.