- Department of Geology and Jewelry, Lanzhou Resourses and Environment Voc-Tech College, Lanzhou, China
Understanding property changes in soil improvement using a new technique is critical for enhancing engineering activity. However, little is known about nano-SiO2 pile-induced changes in soil properties due to its excellent properties as an alternative new additive material. This study aims to examine the changes in properties of loess stabilized with nano-SiO2 pile after curing for 28 days. Using samples taken from four desired radii (i.e., 5, 10 15, and 20 cm), we tested their mineralogical, structural, physicochemical, and index properties and analyzed the relationship of these properties to strengthen the link between microscopic characteristics and macroscopic behaviors. We then discussed the mechanism of the changes in the treated loess properties. The test results show that nano-SiO2 pile induced a physical structure modification in the treated loess and, consequently, an isotropic process with coarser particles due to crystallinity. This resulted in a solidification process in loess with nano-SiO2 pile, causing a decrease in water content and void ratio as well as an increase in natural density, thereby improving its mechanical strength. Meanwhile, the specific surface area (SSA)and cation exchange capacity (CEC) of treated loess were smaller than those of original loess, and there were slight changes in chemical properties. The disintegration rate of treated loess decrease compared with the original loess. The interactional relationship of the microscopic and macroscopic observation was facilitated to better understand the mechanism of changes in nano-SiO2 pile-treated loess properties. This finding reveals that nano-SiO2 pile has the potential as an alternative stabilized technique in loess improvement due to its obvious performance improvement and slight chemical changes.
Introduction
A large number of highways and railways have been constructed in China’s loess area. Meanwhile, urbanization promotes the significant scale of land creation projects by cutting hilltops and then filling valleys (Zhang and Wang, 2018). However, loess also easily collapses and experiences subsidence through wetting and loading. Hence, it is important to improve its performance in engineering projects (Zhang et al., 2018a; Kong et al., 2018).
Considerable research has explored ways to improve loess performance using various stabilizing treatment techniques, but much of this effort has focused on changes in the properties of the treated loess by mixing different chemical additives (Zia and Fox, 2000; Tchakalova and Todorov, 2008; Sariosseiri and Muhunthan, 2009; Arrua et al., 2010; Metelková et al., 2012; Zhang et al., 2017; Zhang et al., 2018a; Haeri et al., 2019). Generally, these chemical additives, such as lime, fly ash, and cement, are added into the loess in order to modify its physical, chemical, structural, and mineral properties, thereby improving its geotechnical performance. Efforts have also sought to use alternative new materials, such as nano-SiO2, to replace the generally used chemical additives in loess performance improvement (Ren and Hu, 2014; Kong et al., 2018; Tabarsa et al., 2018). Such research demonstrated that the nano-SiO2 particle has potential as a cost-effective and eco-friendly stabilized agent for loess improvement. In addition, a few studies have examined the pile treatment technique for loess improvement (Dolgikh, 1966; Tystovich et al., 1971; Pei et al., 2015). The still widely used agents of stabilized piles are chemical additives, such as lime and fly ash (Tystovich et al., 1971; Pei et al., 2015). In developed sites, pile treatment techniques are more practical than mixing treatment methods (Pei et al., 2015). Hence, using a stabilization treatment technique that can be easily applied in various contexts is important for soil improvement. Another area of research interest is seeking alternative new materials to replace the traditional chemical additives for eco-friendly and cost-effective purposes.
Pile treatment techniques have been used to improve the slope, Earth embankment, and clay soils (Rogers and Glendinning, 1997; Rajasekaran and Narasimha Rao, 2002; Larsson et al., 2005; Barker et al., 2006, 2007; Gallagher et al., 2007; Saoudi et al., 2013; Abiodun and Nalbantoglu, 2014; Helle et al., 2017; Selvakumar and Soundara, 2019). According to the nature of the stabilizing agent, pile treatment techniques can be divided into two different types. The first type is for chemical piles, which are generally filled by lime, fly ash, cement, and salt additives (Rogers and Glendinning, 1997; Larsson et al., 2005; Barker et al., 2006, 2007; Pei et al., 2015; Quang and Chai, 2015; Helle et al., 2017). The improvement mechanism of chemical pile-treated soils is mainly attributed to ion migration, which generates a chemical reaction with the surrounding soil. The second type is for physical piles, which are currently made up of stone or colloidal silica grout without a chemical reaction with neighboring soil (Gallagher et al., 2007; Saoudi et al., 2013). The reinforced effect of the stone pile comes from its own mechanical properties Saoudi et al. (2013), Castro (2017), while stabilization from colloidal silica can also be attributed to silica nanoparticle migration (Gallagher et al., 2007). On the whole, chemical piles are still more generally practiced in soil improvement, but the interest in physical piles such as silica nanoparticle has increased due to its eco-friendly characteristics and effectively stabilized effects.
The purpose of this study is to examine the changes in properties of loess stabilized with nano-SiO2 pile. We treated loess with nano-SiO2 pile and cured it for 28 days. The treated loess was subsequently sampled at four radial distances from the pile (i.e., 5, 10, 15, and 20 cm). We examined their mineralogical, structural, physicochemical, disintegrated, and index properties, and then analyzed the relationship of these properties to define the link between microscopic characteristics and macroscopic behaviors. Ultimately, we discussed the mechanism of the changes in the treated loess properties.
Materials and Methods
Tested Samples
The sample used in this study was taken from the Malan loess in Lanzhou City, Gansu Province, China. Nano-SiO2 used as the treated pile is commercially available with a mean aggregate size of 20 μm. Their basic properties are provided in Table 1.
Sample Preparation
We used Pei et al.‘s Pei et al. (2015) procedure for sample preparation. First, using a plastic hammer, large clods and aggregations were broken into homogeneously sized particles that could pass through a sieve with a 1 mm aperture. Second, to achieve the original water content of 15%, distilled water was added to oven-dried loess and thoroughly mixed by hand. The wet loess was then sealed and stored for 24 h at room temperature before packing. The more uniformly distributed loess was placed in the test tank (45 cm × 45 cm × 30 cm) with a polyvinyl chloride (PVC) tube (5 cm internal diameter) using the moist tamping method. To get a consistent density, the samples were tamped with 10 layers, and each layer was designed to have 1.73 g/cm3 of wet density.
Distilled water was added to the dry nano-SiO2 to achieve 15% water content by weight. To minimize the aggregation as much as possible, a glass stirring rod was used to stir in the mixture. The mixture was quickly poured into the pre-positioned PVC tube to form the stabilized nano-SiO2 pile (measuring 5 cm in diameter and 10 cm in length). The PVC tube was gradually pulled out as each layer was tamped.
The loess with the nano-SiO2 pile in the middle of was cured for 28 days at room temperature. After curing, the samples were collected using a unique cutting ring (2.8 cm diameter and 1.5 cm height) at the desired radius (i.e., 5, 10, 15, and 20 cm) from the periphery of the nano-SiO2 pile (Figure 1). The physicochemical and structural properties of these samples were then defined. Meanwhile, the mechanical test was conducted on the desired radii at another depth. In the limited test tank, the radial sampling pattern facilitated examination of the nano-SiO2 migration-induced changes in the properties of the samples, as observed in the loess with lime and fly ash piles (Pei et al., 2015). It should be noted that the sampling has not been conducted in vertical profile, because the limited size of test tank is difficult to take multiple undisturbed samples in the depth of 10 cm.
Sample Tests
Mineralogical Tests
To examine the mineralogical changes after the curing, X-ray diffraction (XRD) techniques were performed on the original and treated loess, which was collected from the specified radial point. All samples were scanned using a Philips PW 3710 X-ray diffractometer with Cu-Kα radiation. Data were recorded from 5 to 45 at a rate of 0.05°2θ/s per step. Meanwhile, a thermogravimetric analysis, including mass loss (TG) and its derivative (DTG), was performed up to 1,000°C in oven-dried samples, at a ramp of 10°C/min on a Perkin-Elmer Instrument, Waltham, MA. In this thermogravimetric test, 10 mg of sample was filled into the instrument.
Structural Tests
The macro-texture and micro-structure of the original and treated loess were examined. The macroscopic particle size distributions of these samples were determined using the sieve and sedimentation methods (ASTMD422-63, 2007). The micro-morphology and micro-size were observed on the representative samples after metallization with gold powder using a JSM-5600 LV Scanning Electron Microscope (SEM).
The slake test has been recommended to assess the aggregation or cementation of soil treated by stabilization agents Pei et al. (2015), due to directly visual structure stability. The slake test was performed on each desired radius. Cylindrical samples were prepared after the oven-dry test. The four samples on each desired radius were simultaneously soaked in the plate, and water completely covered them. Their stability was recorded accompaning the time by camera. Complete disintegration was indicated by no further breakage of samples. The disintegration rate was calculated by dry weigt of the samples divided by the completely disintegrated time.
Index Property Tests
Hardness value was determined using an Equotip hardness tester (Piccolo). Previous research has sought to assess the strength of loess stabilized with lime and fly ash piles (Pei et al., 2015). This study found the Equotip to be a convenient quantitative tool for assessing the changes in strength and stiffness of tread soils due to its ease of use and rapid outcomes. This study used the Equotip hardness test on a newly planed soil surface after obtaining the cutting ring samples, which allowed for rapid and multiple measurements at the desired radius. The hardness value was determined as the average value of 30 measurements along each desired radius from the nano-Sio2 pile edge.
Density and water contents are two of the most commonly determined properties in characterizing the engineering behavior of soil. The preferred test method for the laboratory determination of the moisture content is the oven drying method. Density and water contents are determined as the reduction in the mass of the test specimen after oven drying. The specimens, cut using a circular ring of iron, were 2.8 cm in diameter and 1.5 cm in height at each desired radial point. All specimens were weighed, recorded, and then oven dried at 105°C for 24 h. The mass of the dry sample was recorded. The density and water contents were subsequently calculated, and the average value was used in the analysis.
Liquid and plastic limits were measured using the fall cone test following the Chinese standard procedures GB/T50123 (1999), which define the liquid and plastic limits for penetration as 17 and 2 mm, respectively. The tested samples were taken along each desired radius.
Physicochemical Tests
The total SSA and CEC of the samples were measured using a methylene blue spot test method. The test procedure for determining the total SSA of soil was described by Santamarina et al. (2002), and its CEC was calculated using the formula suggested by Çokça and Birand (1993). Chemical properties were measured using a water quality monitor, including pH, total dissolved solids (TDS), electrical conductivity (CE), and oxidation-reduction potential (ORP) of extracts from 1:5 soil to water.
Results and Analyses
Changes in Mineralogical Composition
XRD Diffractograms
The results of the XRD of the original loess and loess treated with nano-SiO2 pile at different radial distances are shown in Figure 2. Based on the XRD diffractograms, there is no new reflection; only intensity is enhanced. Sharp changes occurred in intensity and full width in all treated loess, and the crystalline structure seemed to be quite insensitive after nano-SiO2 mitigation into loess around the pile. Generally, the changes in reflection shape can be related to a structural adjustment that fabric tends to become more isotropic due to the increase in crystallinity (Choquette et al., 1987; Souli et al., 2013). Thus, only physical structure modification exists while lacking the chemical reaction (Kong et al., 2018). Meanwhile, a slight difference occurred in the mixture of nano-SiO2 added into the loess; the intensity and full width indicated only slight enhancement in XRD diffractograms (Kong et al., 2018). As a whole, the physical structure modification shows that an isotropic process occurred due to the increase of crystallinity.
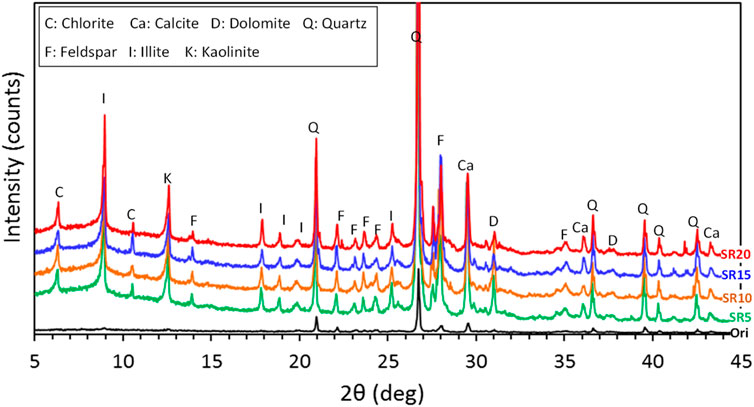
FIGURE 2. X-ray diffractions of original loess and treated loess at different radial distances from the nano-SiO2 pile.
TG-DTA Curves
The TG-DTA results of this study are presented in Figure 3. Among these results, the TG-DTA curves indicated two noticeable changes in two temperature domains. The first significant drop finished at about 150°C, corresponding to the loss of absorbed water in treated the loess. The second striking change occurred in the temperature range from 600°C to 780°C. The samples influenced by nano-SiO2 migration showed relatively lower and narrower peaks with lesser mass loss, and the turning point of the curves shifted toward a slightly lower temperature. In the first domain, the loss of the absorbed water of original loess is related to the absorption of migrated nano-SiO2 due to its large surface area and high activity. Menwhile, it is striking compared to the changes in the second domain. Previous studies have found that more hydration products causing denser structure can produce taller and wider peaks (Wu et al., 2016), and the shift in the turning point toward a lower temperature is derived from a more stable stage for materials (Galindo et al., 2015). Hence, the apparent changes in the second domain contributed to the generation of a more stable structure. Meanwhile, a looser structure existed in the sample with a more significant distance from the nano-SiO2 pile. These findings also support the XRD results.
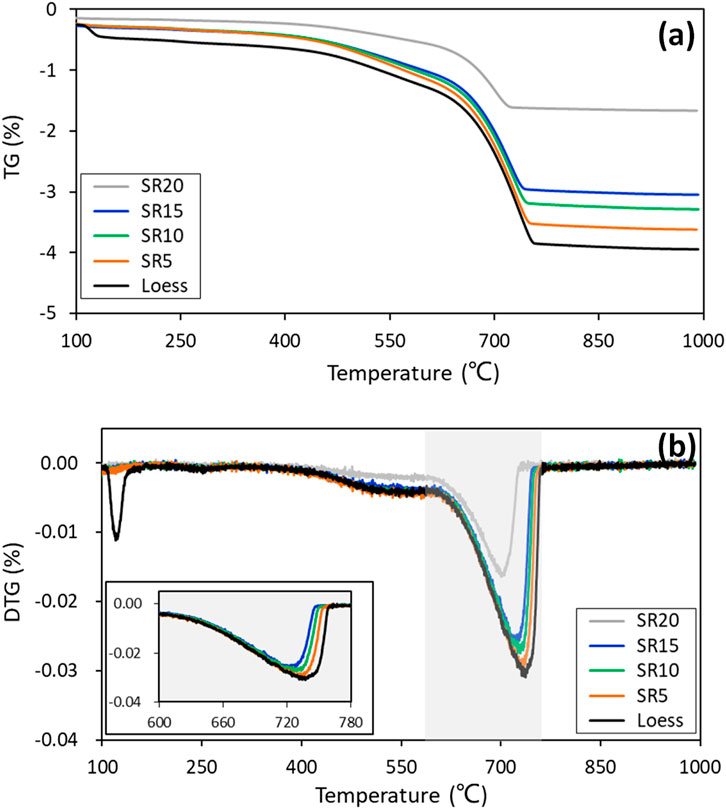
FIGURE 3. TG-DTG curves of original loess and treated loess at different radial distances from the nano-SiO2 pile.
Changes in Texture and Structure
Macro-Texture From Particle Size Curves
The particle size curves of original loess and loess treated with nano-SiO2 pile are shown in Figure 4. The macrostructure changes suggest a slight increase in the coarse fraction in the treated loess, with samples farther from the nano-SiO2 pile becoming coarser. As a whole, the loess influenced by the nano-SiO2 pile has a higher uniformity coefficient than that of the original loess. This finding also supports the idea that the loess involves an isotropic process with coarser particles due to crystallinity.
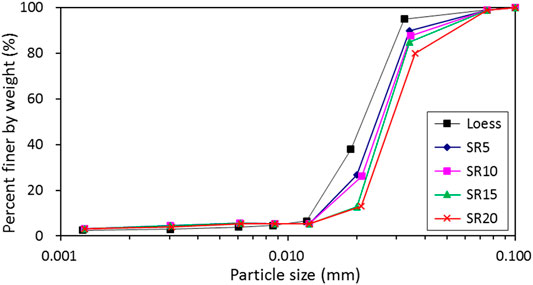
FIGURE 4. Particle size curves of original loess and treated loess at different radial distances from the nano-SiO2 pile.
Micro-structure From SEM Micrographs
The SEM micrographs of the loess at 5 and 20 cm radial distances from the nano-SiO2 pile are shown in Figure 5. The microstructure of the samples closer to the nano-SiO2 pile is denser and more filling, with a more homogeneous state (Figure 5A), whereas that of the farther example is a looser and larger void space, with greater aggregation and more contact (Figure 5B). This result can be attributed to the degree of nano-SiO2 migration into the loess. When the loess is close to the nano-SiO2 pile, the degree of its migration is high; as a result, the nano-SiO2 filled the pores and adhered to the soil particles (Figure 5A). Meanwhile, low nano-SiO2 migration occurred in the loess farther from the pile. The few nano-SiO2 particles mainly adhered to the surface of the soil particles, strengthening the bonding, while also filling the pores between particles. These created a bridge effect to link together soil particles as greater aggregates with larger void space (Figure 5B). The observation from microstructures effectively explains the changes in the particle size curve of the samples at a different radial distance from the nano-SiO2 pile.
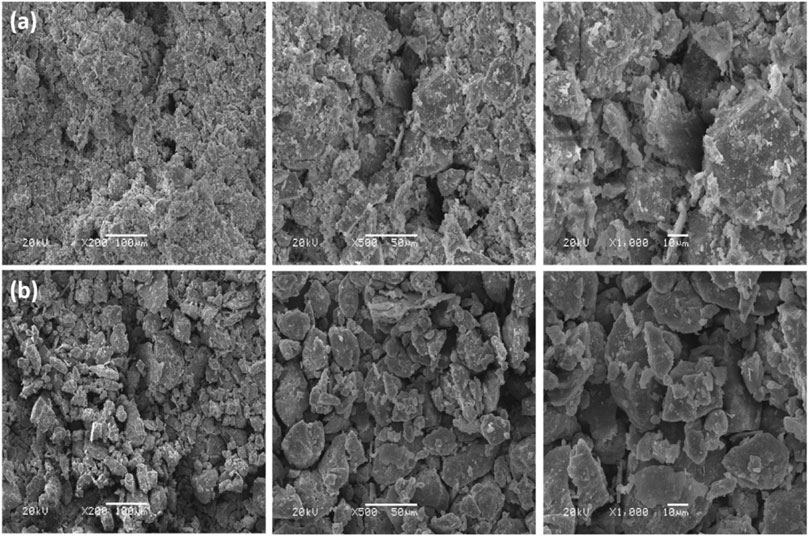
FIGURE 5. SEM images of treated loess with a nano-SiO2 pile at different amplification factors: (A) 5 cm radius from the pile; (B) 20 cm radius from the pile.
Aggregate Structure Stability From Disintegration
Figure 6 shows the disintegration characteristics of treated loess at different radial distances from the nano-SiO2 pile with soaking time, and Figure 7 shows disintegration rate of treated loess at different radial distances from the nano-SiO2 pile. It can be seen that the disintegration rate of the treated loess has an obvious decrease acomparing with original loess, and that the disintegration rate of the treated loess increased with with increasing radius from the piles (Figures 6, 7). These trends are matched with the denser packing from micro-structure observation, as shown in Figure 5. These results reveal that that the treated loess with the nano-SiO2 pile have stronger cementation of aggregates, leading to more stable structure between aggregates.
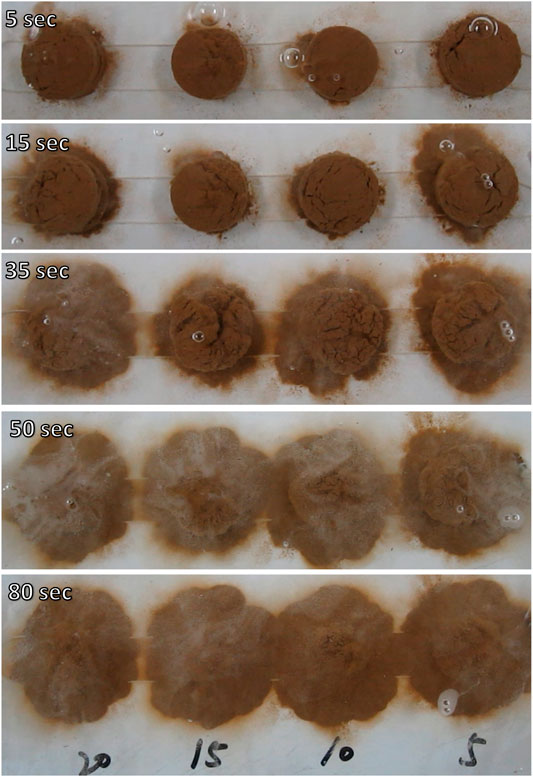
FIGURE 6. Disintegration characteristics of treated loess at different radial distances from the nano-SiO2 pile.
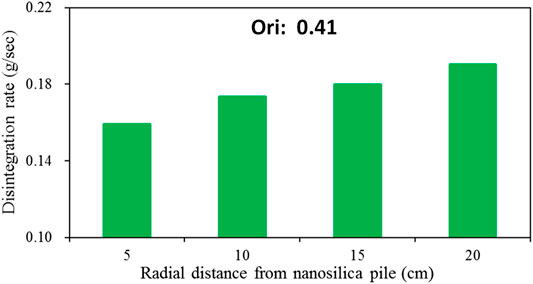
FIGURE 7. Disintegration rate of treated loess at different radial distances from the nano-SiO2 pile.
Changes in Index Properties
Basic State From Water Content, Density, and Void Ratio
The changes in water content, natural density, and void ratio of treated loess with different radial distances from the nano-SiO2 pile are shown in Figure 8. As indicated, the water content of loess treated with the nano-SiO2 pile is lower than that of the original loess (Figure 8A), while the natural density of the treated loess is noticeably greater than the original one (Figure 8B). As a result, the void ratio of the treated loess is strikingly low compared to that of the original loess (Figure 8C). On the other hand, as shown in Figure 8, when the treated loess is closer to the nano-SiO2 pile, the decrease in water content and the void ratio are stronger, and the increase in natural density tapers off as radial distance from the pile increases. Consequently, the loess treated with the nano-SiO2 pile becomes dryer and denser, essentially producing a solidification process—namely, the water is removed and the densification also occurs in the treated loess (Zhang et al., 2018a). These state changes in the treated loess can attribute to the nano-SiO2 migration, which has dual roles during the progress. Two direct effects involve adsorption and filling due to the special properties of nano-SiO2, and one indirect derivative effect is the generation of tighter bonding between aggregates or particles. Meanwhile, the stronger effects in the treated loess are closer to the nano-SiO2 pile.
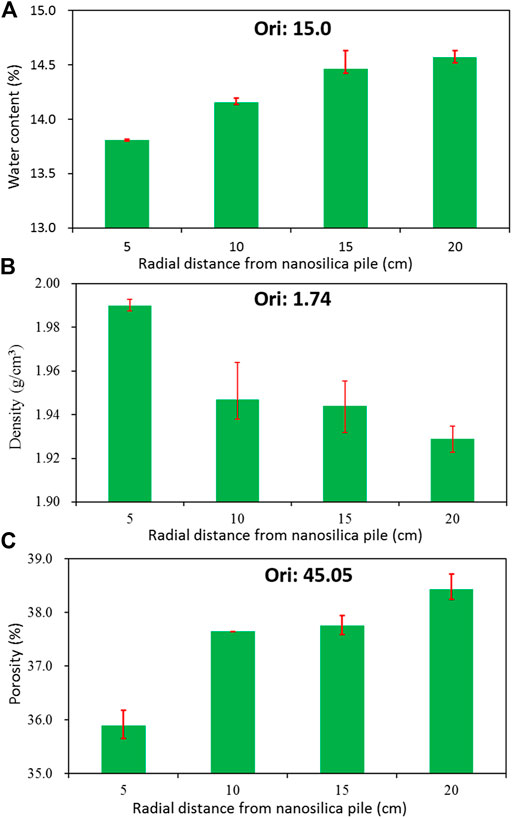
FIGURE 8. Basic state of treated loess at different radial distances from the nano-SiO2 pile: (A) water content; (B) wet density; (C) porosity.
The changes in the Atterberg limit of treated loess at different radial distances from the nano-SiO2 pile are shown in Figure 9. As shown, the nano-SiO2 migration has almost no effects on liquid limit, plastic limit, or plasticity index. This may be because the treated loess has no essential change in composition, with modification of only its structure and state. The results indirectly support that this is lacking for chemical reactions.
Mechanical Strength From Hardness Value
The changes in the hardness of treated loess at different radial distances from the nano-SiO2 pile are shown in Figure 10. The hardness of the treated loess decreases as the radius from the nano-SiO2 pile increases, but the hardness values is greater than that of loess treated with the lime and fly ash piles (Pei et al., 2015). In addition, no similar radial cracks occurred in the loess stabilized with the lime pile. This means that there should have a greater mechanical strength in the loess stabilized with the nano-SiO2 pile than in the untreated loess. The increase in the mechanical strength is related to the nano-SiO2 migration, which results in structure and state modification of the treated loess. Hence, the mechanical improvement is attributed to the coarsening effect and solidification process of the treated loess (i.e., large aggregate formation) as well as the dryer and denser state with more contact, smaller void space and more stable structure (as shown in Figures 4–8). Furthermore, the weakening strength improvement depends on the degree of nano-SiO2 migration as the radius from the pile increases.
Changes in Physicochemical Properties
Particle or Aggregate Surface Properties From SSA and CEC
The changes in SSA and CEC of treated loess at different radial distances from the nano-SiO2 pile are shown in Figure 11. Both SSA and CEC increase as the radial distance from the nano-SiO2 pile increases, but all measurements are smaller than the original loess ones. The decrease in SSA and CEC is due to the formation of large aggregates due to the nano-SiO2 migration. Nevertheless, their increasing trends seem to contradict the results of the particle size distribution (Figure 4). However, the increase in SSA and CEC with increasing radial distance from the nano-SiO2 pile is a reasonable expectation. The total SSA and CEC depend not only on the particle size of a soil, but also on the structure of the soil (Zhang et al., 2018b). Previous research has found that the formation of larger aggregate clusters can result in a larger void space (Zhang et al., 2013). As observed in microstructural images (Figure 5), the treated loess farther from the nano-SiO2 pile showed a larger void space in the greater aggregates. Hence, the higher SSA and CEC of the farther treated loess could be attributed to a larger surface contact area due to its structure modification.
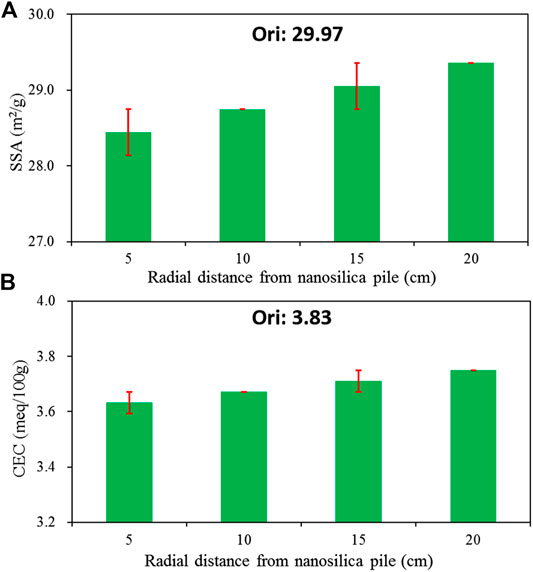
FIGURE 11. Specific surface area (SSA) and cation exchange capacity (CEC) of treated loess at different radial distances from the nano-SiO2 pile.
Soil Chemical Properties From EC, TDS, pH, and ORP
The changes in chemical properties (i.e., EC, TDS, pH, and ORP) of treated loess at different radial distances from the nano-SiO2 pile are shown in Figure 12. As a whole, the EC and TDS of the treated loess with radial distance from the nano-SiO2 pile, and their pH and ORP, accordingly decrease with increasing radius. It should be noted that the EC and TDS of the treated loess at radii of 5 and 10 cm are very slightly lower compared to the original loess, and the values at radii of 15 and 20 cm are also slightly higher compared to the original loess. The pH and ORP of the treated loess are overall lower than those of the original loess. This trend clearly shows the effect of nano-SiO2 migration into loess, causing the changes in chemical properties due to the high activity and small size of the nano-SiO2 particle. The very slight changes in chemical properties illustrate that the loess treated with nano-SiO2 can keep an almost constant chemical environment. Thus, the nano-SiO2 particle has the potential to act as an eco-friendly stabilized additive in loess improvement.
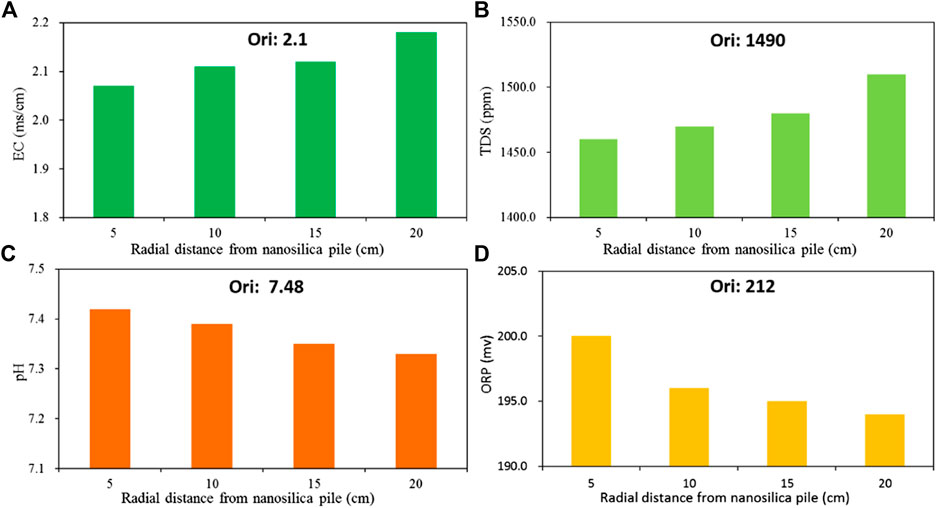
FIGURE 12. Chemical properties of treated loess at different radial distances from the nano-SiO2 pile: (A) EC; (B) TDS; (C) pH; (D) ORP.
Discussion
Nano-SiO2 Migration-Induced Changes in Loess Properties
The results presented herein confirm that the nano-SiO2 migration modifies the properties of the nano-SiO2 pile treated loess. The structural, mechanical, physicochemical, and index properties all change, but the mineral composition experiences no noticeable change. In the previous studies, these have also no observable compositon modification to different nano-particles treated clay, silt and sands, even longer curing periods (Taha and Taha, 2012; Choo et al., 2015; Kong et al., 2018). Meanwhile, the very slight changes in EC and TDS can support the unobservable compositon modification. This is due to the fact that the chemical properties have close links to the mineral compositions. However, the particle coating, cemention between aggregates, more uniform aggregate formation have contributed to the physcial structure modification, which responsible for the changes in mechanical, physicochemical, and index properties (Kong et al., 2018; Kong et al., 2019). Hence, the changes in the treated loess properties are related to the physical structure modification rather than a chemical reaction.
It is interesting to note that the same initial water content existed between the loess and nano-SiO2, which prevented the generation of the pore water pressure gradient. Here should mainly be adsorption force and capillary force. The nano-SiO2 migration would be attributed to its dispersion because many previous studies have proved that nanoparticles are easily dispersed in various media (Li et al., 2004; Yao et al., 2005; Gallagher et al., 2007; Hendraningrat and Torsæter, 2015). Research using nano-SiO2-treated concrete also found that large contents can seriously inhibit the uniform dispersion of nanoparticles (Li, 2004). Meanwhile, the dispersion can contributed to the special particle features, i.e. extremely small particle size and extremely great surface area, and correspondingly strong surface energy. It is should to noted that the density and water content of nano-SiO2 migrated loess are different in that of nano-SiO2 mixed loess. Theos treated loess by mixing nano-SiO2 has slight decrese in water content and density (Kong et al., 2018). This may be relative with their reaction progress, but there still has a need to do a further study. As the difference of the stabilizing treatment techniques has important influence on its practial appilcation and availability. Aa a whole, a small amount of nano-SiO2 particles migrating from the pile into loess may facilitate improving loess performance, and thus it has potential as a new treatment technique for soil improvement.
Link Between Microscopic Characteristics and Macroscopic Behaviors
The observation of macroscopic behaviors and microscopic features identified a vital link. Nano-SiO2 particle migration results in an isotropic aggregate in the treated loess, causing a denser and dryer state (Figures 4–8). Based the observation of SEM photograph and necessary state (Figure 5 and Figure 8), an increase in micro-pores occurs at the expense of the macropores, with a significant decrease in the total pore volume of the treated loess. Meanwhile, the agglomerated effect causes the formation of coarser aggregates and the denser packing accompanying the consumption of water (Figures 4–8), which generates greater mechanical strength (Figure 10) and smaller SSA and CEC in the treated loess (Figure 11). The chemical properties indicate slight changes in CE, TDS, pH, and ORP, without significant modification of the treated loess (Figure 12). Thus, the nano-SiO2 has the potential to be used as an eco-friendly additive in soil improvement. On the other hand, no new XRD diffractograms are generated in the treated loess, but SEM observation affords a direct method for changes to the microscopic characteristics.
Conclusion
The microscopic characteristics (such as mineralogical and structural properties) and macroscopic behaviors (such as basic state, mechanical, and physicochemical properties) of loess treated with the nano-SiO2 pile were observed. Based on the test results, the following conclusions can be drawn:
1 The nano-SiO2 migration induced the changes in microscopic characteristics and macroscopic behaviors of the treated loess, producing an interactional relationship among them. The linked relationships facilitate the understanding of the mechanism of changes in nano-SiO2 pile-treated loess properties.
2 No obvious changes occurred in the mineralogical composition of XRD diffractograms, but SEM photographs and slake tests show apparent structural modification in the treated loess with the nano-SiO2 pile. An isotropic process is evident with coarser particles, more filling and denser packing due to crystallinity. As a result, the treated loess with the nano-SiO2 pile have stronger water stability.
3 The loess treated with nano-SiO2 pile produces a solidification process, causing a decrease in water content and void ratio as well as an increase in natural density, thereby improving its mechanical strength.
4 The loess treated with nano-SiO2 pile modifies the physicochemical properties. The SSA and CEC of treated loess are smaller than those of the original loess but include an increasing trend moving farther from the pile, resulting in a slight change in chemical properties.
On the whole, the nano-SiO2 pile migration induced the relevant changes in the treated loess due to the nanoparticle dispersion. The modification of the physical structure and basic state resulted in the improvement of the performance of the treated loess. Meanwhile, nano-SiO2 has the potential to be an eco-friendly stabilized additive in loess performace improvement due to its slight changes in chemical properties.
Data Availability Statement
The original contributions presented in the study are included in the article/supplementary material, further inquiries can be directed to the corresponding author.
Author Contributions
RK performed the tests and analyzed the data, RK wrote the manuscript text. LG, WZ and BZ revised the manuscript, RK and LG sourced the funds for the study.
Funding
This study was partially supported by Gansu University Innovation Fund Project (No. 2021A-255) and Industrial support and guidance project of colleges and universities in Gansu Province in 2019: Research and key technology of coal mine reclamation in Gansu Province (No.:2019C-16).
Conflict of Interest
The authors declare that the research was conducted in the absence of any commercial or financial relationships that could be construed as a potential conflict of interest.
Publisher’s Note
All claims expressed in this article are solely those of the authors and do not necessarily represent those of their affiliated organizations, or those of the publisher, the editors and the reviewers. Any product that may be evaluated in this article, or claim that may be made by its manufacturer, is not guaranteed or endorsed by the publisher.
Acknowledgments
The authors are also grateful to the two reviewers and editor for their valuable comments to improve the quality of this paper.
References
Abiodun, A. A., and Nalbantoglu, Z. (2014). Lime Pile Techniques for the Improvement of clay Soils. Can. Geotechnical J. 52, 760–768. doi:10.1139/cgj-2014-0073
Arrua, P., Aiassa, G., and Eberhardt, M. (2010). Loess Soil Stabilized with Cement for Civil Engineering Applications. Int. J. Earth Sci. Eng. 5, 10–17.
ASTMD422-63 (2007). Standard Test Method for Particle-Size Analysis of Soils. West Conshohocken, PA: ASTM International.
Barker, J. E., Rogers, C. D., and Boardman, D. I. (2006). Physio-chemical Changes in clay Caused by Ion Migration from Lime Piles. J. Mater. Civ. Eng. 18, 182–189. doi:10.1061/(asce)0899-1561(2006)18:2(182)
Barker, J. E., Rogers, C. D. F., and Boardman, D. I. (2007). Ion Migration Associated with Lime Piles: a Review. Proc. Inst. Civil Eng. - Ground Improvement 11, 87–98. doi:10.1680/grim.2007.11.2.87
Choo, H., Larrahondo, J., and Burns, S. E. (2015). Coating Effects of Nano-Sized Particles onto Sand Surfaces: Small Strain Stiffness and Contact Mode of Iron Oxide-Coated Sands. J. Geotech. Geoenviron. Eng. 141, 04014077. doi:10.1061/(asce)gt.1943-5606.0001188
Choquette, M., Bérubé, M., and Locat, J. (1987). Mineralogical and Microtextural Changes Associated with Lime Stabilization of marine Clays from Eastern Canada. Appl. Clay Sci. 2, 215–232. doi:10.1016/0169-1317(87)90032-9
Çokça, E., and Birand, A. A. (1993). Determination of Cation Exchange Capacity of Clayey Soils by the Methylene Blue Test. Geotechnical Test. J. 16, 518–524. doi:10.1520/GTJ10291J
Dolgikh, P. D. (1966). Stabilization of Slumping Loess Soils by Injection of a Lime-Slag Suspension. Soil Mech. Found. Eng. 3, 272–273. doi:10.1007/bf01703524
Galindo, R., López-Delgado, A., Padilla, I., and Yates, M. (2015). Synthesis and Characterisation of Hydrotalcites Produced by an Aluminium Hazardous Waste: A Comparison between the Use of Ammonia and the Use of Triethanolamine. Appl. Clay Sci. 115, 115–123. doi:10.1016/j.clay.2015.07.032
Gallagher, P. M., Pamuk, A., and Abdoun, T. (2007). Stabilization of Liquefiable Soils Using Colloidal Silica Grout. J. Mater. Civ. Eng. 19, 33–40. doi:10.1061/(asce)0899-1561(2007)19:1(33)
GB/T50123 (1999). Standard for Soil Test Method. Ministry of Construction, P.R. China. (in Chinese).
Haeri, S. M., Akbari Garakani, A., Roohparvar, H. R., Desai, C. S., Seyed Ghafouri, S. M. H., and Salemi Kouchesfahani, K. (2019). Testing and Constitutive Modeling of Lime-Stabilized Collapsible Loess. I: Experimental Investigations. Int. J. Geomech. 19, 04019006. doi:10.1061/(asce)gm.1943-5622.0001364
Helle, T. E., Aagaard, P., and Nordal, S. (2017). In Situ improvement of Highly Sensitive Clays by Potassium Chloride Migration. J. Geotech. Geoenviron. Eng. 143, 04017074. doi:10.1061/(asce)gt.1943-5606.0001774
Hendraningrat, L., and Torsæter, O. (2015). A Stabilizer that Enhances the Oil Recovery Process Using Silica-Based Nanofluids. Transp. Porous Med. 108, 679–696. doi:10.1007/s11242-015-0495-8
Kong, R., Yan, B., Xu, J., Shi, Z., Peng, Q., and Lin, X. (2019). Physical Homogenization and Chemical Stability of Nano-SiO2 Treated Loess. Soil Mech. Found. Eng. 56, 336–339. doi:10.1007/s11204-019-09611-9
Kong, R., Zhang, F., Wang, G., and Peng, J. (2018). Stabilization of Loess Using Nano-SiO2. Materials 11, 1014. doi:10.3390/ma11061014
Larsson, S., Stille, H., and Olsson, L. (2005). On Horizontal Variability in Lime-Cement Columns in Deep Mixing. Géotechnique 55, 33–44. doi:10.1680/geot.55.1.33.58586
Li, G. (2004). Properties of High-Volume Fly Ash concrete Incorporating Nano-SiO2. Cement Concrete Res. 34, 1043–1049. doi:10.1016/j.cemconres.2003.11.013
Li, H., Xiao, H.-g., and Ou, J.-p. (2004). A Study on Mechanical and Pressure-Sensitive Properties of Cement Mortar with Nanophase Materials. Cement Concrete Res. 34, 435–438. doi:10.1016/j.cemconres.2003.08.025
Metelková, Z., Boháč, J., Přikryl, R., and Sedlářová, I. (2012). Maturation of Loess Treated with Variable Lime Admixture: Pore Space Textural Evolution and Related Phase Changes. Appl. Clay Sci. 61, 37–43. doi:10.1016/j.clay.2012.03.008
Pei, X., Zhang, F., Wu, W., and Liang, S. (2015). Physicochemical and index Properties of Loess Stabilized with Lime and Fly Ash Piles. Appl. Clay Sci. 114, 77–84. doi:10.1016/j.clay.2015.05.007
Quang, N. D., and Chai, J. C. (2015). Permeability of Lime- and Cement-Treated Clayey Soils. Can. Geotech. J. 52, 1221–1227. doi:10.1139/cgj-2014-0134
Rajasekaran, G., and Narasimha Rao, S. (2002). Permeability Characteristics of Lime Treated marine clay. Ocean Eng. 29, 113–127. doi:10.1016/s0029-8018(01)00017-8
Ren, X., and Hu, K. (2014). Effect of Nanosilica on the Physical and Mechanical Properties of Silty clay. Nanosci. Nanotechnol. Lett. 6, 1010–1013. doi:10.1166/nnl.2014.1857
Rogers, C. D. F., and Glendinning, S. (1997). Improvement of clay Soils In Situ Using Lime Piles in the UK. Eng. Geology 47, 243–257. doi:10.1016/s0013-7952(97)00022-7
Santamarina, J. C., Klein, K. A., Wang, Y. H., and Prencke, E. (2002). Specific Surface: Determination and Relevance. Can. Geotech. J. 39, 233–241. doi:10.1139/t01-077
Saoudi, N. K. S. A., Rahil, F., and Abbawi, Z. (2013). Soft Soil Improved by Stone Columns And/or Ballast Layer. Ground Improvement 168, 179–186.
Sariosseiri, F., and Muhunthan, B. (2009). Effect of Cement Treatment on Geotechnical Properties of Some Washington State Soils. Eng. Geology 104, 119–125. doi:10.1016/j.enggeo.2008.09.003
Selvakumar, S., and Soundara, B. (2019). Swelling Behaviour of Expansive Soils with Recycled Geofoam Granules Column Inclusion. Geotextiles and Geomembranes 47, 1–11. doi:10.1016/j.geotexmem.2018.08.007
Souli, H., Fleureau, J.-M., Trabelsi-ayadi, M., and Taibi, S. (2013). A Multi-Scale Study of the Effect of Zinc and lead on the Hydromechanical Behaviour and Fabric of a Montmorillonite. Géotechnique 63, 880–884. doi:10.1680/geot.13.p.016
Tabarsa, A., Latifi, N., Meehan, C. L., and Manahiloh, K. N. (2018). Laboratory Investigation and Field Evaluation of Loess Improvement Using Nanoclay - A Sustainable Material for Construction. Construction Building Mater. 158, 454–463. doi:10.1016/j.conbuildmat.2017.09.096
Taha, M. R., and Taha, O. M. E. (2012). Influence of Nano-Material on the Expansive and Shrinkage Soil Behavior. J. Nanoparticle Res. 14, 1–13. doi:10.1007/s11051-012-1190-0
Tchakalova, B., and Todorov, K. (2008). Plastic Soil-Cement Mixtures for Isolation Barriers. Geologica Balcanica 37, 91–96.
Tystovich, N., Abelev, M., and Takhirov, I. (1971). “Compacting Saturated Loess Soils by Means of Lime Piles,” in Processings of Fourth Conference on Soil Mechanics and Foundation Engineering. Editor A. Kezdi (Budapest: Akademiai Kiado), 837–842.
Wu, Z., Deng, Y., Liu, S., Liu, Q., Chen, Y., and Zha, F. (2016). Strength and Micro-structure Evolution of Compacted Soils Modified by Admixtures of Cement and Metakaolin. Appl. Clay Sci. 127-128, 44–51. doi:10.1016/j.clay.2016.03.040
Yao, X., Tan, S., Huang, Z., and Jiang, D. (2005). Dispersion of Talc Particles in a Silica Sol. Mater. Lett. 59, 100–104. doi:10.1016/j.matlet.2004.09.025
Zhang, C.-l., Jiang, G.-l., Su, L.-j., and Zhou, G.-d. (2017). Effect of Cement on the Stabilization of Loess. J. Mt. Sci. 14, 2325–2336. doi:10.1007/s11629-017-4365-4
Zhang, F., Kong, R., and Peng, J. (2018b). Effects of Heating on Compositional, Structural, and Physicochemical Properties of Loess under Laboratory Conditions. Appl. Clay Sci. 152, 259–266. doi:10.1016/j.clay.2017.11.022
Zhang, F., Pei, X., and Yan, X. (2018a). Physicochemical and Mechanical Properties of Lime-Treated Loess. Geotech. Geol. Eng. 36, 685–696. doi:10.1007/s10706-017-0341-6
Zhang, F., and Wang, G. (2018). Effect of Irrigation-Induced Densification on the post-failure Behavior of Loess Flowslides Occurring on the Heifangtai Area, Gansu, China. Eng. Geology 236, 111–118. doi:10.1016/j.enggeo.2017.07.010
Zhang, F., Wang, G., Kamai, T., Chen, W., Zhang, D., and Yang, J. (2013). Undrained Shear Behavior of Loess Saturated with Different Concentrations of Sodium Chloride Solution. Eng. Geology 155, 69–79. doi:10.1016/j.enggeo.2012.12.018
Keywords: loess, nano-SiO2 pile, nanoparticle migration, multi-scale analysis, stabilization
Citation: Kong R, Gao L, Zhao W and Zhao B (2021) Multi-Scale Analysis of Geotechnical and Physicochemical Changes in Loess Caused by Nano-SiO2 Pile Migration. Front. Earth Sci. 9:747317. doi: 10.3389/feart.2021.747317
Received: 26 July 2021; Accepted: 27 August 2021;
Published: 15 November 2021.
Edited by:
Gonghui Wang, Kyoto University, JapanReviewed by:
Jin Liu, Hohai University, ChinaYao Jiang, Institute of Mountain Hazards and Environment (CAS), China
Copyright © 2021 Kong, Gao, Zhao and Zhao. This is an open-access article distributed under the terms of the Creative Commons Attribution License (CC BY). The use, distribution or reproduction in other forums is permitted, provided the original author(s) and the copyright owner(s) are credited and that the original publication in this journal is cited, in accordance with accepted academic practice. No use, distribution or reproduction is permitted which does not comply with these terms.
*Correspondence: Ran Kong, a29uZ3JhbjQxM0AxNjMuY29t