- 1Department of Geography, The University of Hong Kong, Pokfulam, Hong Kong, SAR China
- 2Centre for Water Technology and Policy, The University of Hong Kong, Pokfulam, Hong Kong, SAR China
High-gradient headwater streams are major participants in the carbon (C) cycle because of their capabilities of emitting a significant amount of carbon dioxide (CO2). Notwithstanding, their CO2 emissions have been largely overlooked in previous studies owing to their small water surface area and are sometimes strenuous to be measured because of their narrow channel widths and strong turbulence. This study examined the spatial and seasonal variabilities of CO2 dynamics of a subtropical steep headwater stream fed by groundwater. Our study found that the pH and dissolved oxygen exhibited a general increasing trend away from the source of the headwater whereas the partial pressure of carbon dioxide (pCO2) showed a downward trend. The stream water pCO2 in the upper reach was found to be higher than the ambient level by 19–114 times, with an average drop of >70% at just 9.2 m from the groundwater source, demonstrating the potentially large emission of CO2 into the atmosphere within this short distance. Additionally, the sampling works conducted further downstream revealed that the CO2 derived from groundwater could almost completely dissipate within approximately half a kilometer downstream of the source. The concentrations of dissolved organic carbon and pCO2 were also lower during the period with lower air temperatures in the headwater stream, indicating temperature-dependent metabolism and decomposition of organic matter in soil might modulate the C dynamics in the headwater stream, although the rapid gas exchange along the stream remained the determinative factor. Our findings reassert that headwater streams are an essential source of CO2 and disregarding them from the studies of greenhouse gas emissions of inland waters would underestimate their potency to influence the global C cycle.
Introduction
There has been a growing number of studies showing that carbon dioxide (CO2) emissions from inland waters into the atmosphere have substantial repercussions on the regional and global carbon (C) cycle. Headwater streams are a crucial component of the water cycle because they dominate the riparian interface in the world and account for approximately 80% of the Earth’s perennial channels (Downing et al., 2012; Schneider et al., 2020). Headwater streams are also important players in the global C cycle as they receive different forms of C from surrounding environments, with running waters as the “vehicles” that transport these C downstream (Duvert et al., 2018). They can also provide an environment for the development of metabolic processes such as respiration that lead to gaseous C emissions, while aquatic sediments do not typically store substantial amounts of terrestrial C (Bernal et al., 2013; Argerich et al., 2016; Schneider et al., 2020). Studies that analyzed rivers and streams worldwide also showed that greenhouse gas (GHG) emissions decrease with increasing Strahler stream orders, and the amount of annual GHG emission from first order headwater streams is the highest, highlighting they are a hotspot for GHG emissions (Ran et al., 2021a; Li et al., 2021). A regional study on the CO2 emissions from inland waters also observed 61% of the flux is attributable to first and second order streams in China (Ran et al., 2021a). Additionally, past research on the partial pressure of CO2 (pCO2) of stream networks in boreal regions revealed a general decreasing trend of pCO2 and CO2 evasion flux with stream order (Crawford et al., 2013; Wallin et al., 2013). It has been suggested that approximately 36% of the global CO2 emitted from lotic systems is sourced from headwaters (Marx et al., 2017). During the high-flow period when the hydrological connectivity is the highest in a year, CO2 evasion from headwater streams can even dominate the aquatic CO2 loss (Gómez-Gener et al., 2016). These studies unequivocally demonstrated that headwater streams have a significant role to contribute to the global C cycle. In addition, when a headwater stream is connected to a CO2-enriched groundwater source, the rapid evasion of CO2 along the stream may also enable it to serve as an important conduit for terrestrially-respired C (Johnson et al., 2008; Duvert et al., 2018). For determining the extent to which a system is affected by groundwater input, measurement of radon-222 concentration has been identified to be one of the promising solutions (Wu et al., 2004; Mullinger et al., 2007).
Despite the prominence of headwater streams in the C cycle, they have largely been overlooked in C cycle studies because of their narrow width and strong turbulence, making them difficult to use traditional methods, including floating chamber or tracer gas, to estimate the GHG emissions. Furthermore, their water surface area is subject to significant seasonal variations which is the underlying cause of the huge uncertainties in documenting the length and area of streams on both regional and global scales (Benstead and Leigh, 2012; Wallin et al., 2013; Horgby et al., 2019b). These systems can have particularly high emission rates due to their common associations with steep slopes, high flow velocities, and rough riverbeds (Schelker et al., 2016; Maurice et al., 2017; Aho and Raymond, 2019; Horgby et al., 2019b; Ulseth et al., 2019). The substantial differences in the CO2 concentration between headwater streams and the ambient air is another factor that elevates the emission rate of CO2. Headwater streams are typically supersaturated in CO2 owing to a number of factors, such as the mobilization of organic C arising from the degradation of organic matters, the input of CO2-enriched groundwater, as well as the influence of carbonate-dominated catchment lithology (Davidson et al., 2010; Marx et al., 2017; Duvert et al., 2018). It has also been postulated previous studies might have underestimated the magnitude of catchment-scale CO2 degassing flux because samples were collected distant from the groundwater source, thereby having a great potential of underestimating the contribution of groundwater inputs and obscuring the impacts of CO2 evasion (Johnson et al., 2008; Duvert et al., 2018).
Although the relatively small water surface area covered by a single headwater stream signifies that the GHG emissions from an individual headwater stream may not be significant, they are still likely to make a substantial contribution to the global C budget due to their sheer numbers on a global scale. It has been estimated that first—third order systems comprise nearly 48% of the surface area of all rivers and streams globally (Li et al., 2021), implying a substantial fraction of GHG emissions from lotic systems are contributed by low-order headwater streams. Furthermore, the CO2 dynamics (i.e., pCO2 and CO2 emission rate) of headwater streams are spatially and temporally heterogeneous, adding to the difficulty in the accurate quantification of their roles in the global C cycle (Crawford et al., 2017). Although the difficulty of this task cannot be understated, given the above factors, there is a genuine need to better characterize and quantify GHG emissions from headwater streams in order to render greater accuracy to relevant studies in the future. When these studies are more accurately reflective of the actual scale of the GHG emissions, a more comprehensive understanding of the factors contributing to climate change may be gained.
In this study, the spatio-temporal patterns of pCO2 of a high-gradient forested headwater stream fed by groundwater in subtropical Hong Kong were analyzed. We paid particular attention to the rate of CO2 degassing from this headwater stream into the atmosphere. The relationships of pCO2 with selected environmental and hydrological variables, such as pH, dissolved oxygen (DO), and dissolved organic carbon (DOC), were also investigated to identify the potential drivers that have regulated the C dynamics in the studied headwater stream.
Materials and Methods
Study Site
This study was conducted in a headwater stream in the Pokfulam catchment (area: 1.7 km2) in subtropical Hong Kong (22°16′00″N—22°16′08″N, 114°08′25″E—114°08′41″E, Figure 1). The bedrock geology of the study area is dominated by early Cretaceous volcanic rocks (Fletcher, 1997). The annual precipitation is approximately 2,400 mm and the climate is classified as Cwa (monsoon-influenced humid subtropical climate) according to the Köppen climate classification (Cheung et al., 2019). The altitude of the groundwater resurgence is 258 m above the sea level (m.a.s.l.) (Figure 1C). It was fed by groundwater and the groundwater resurgence was visible. A total of nine sampling campaigns were conducted at a monthly interval from June 2020 to February 2021 at the beginning of each month and 7 water samples were taken from the first 520 m of the headwater stream at the sampling sites of 0 m (Site A, the groundwater resurgence), 1.3 m (Site B), 3.3 m (Site C), 5.1 m (Site D), 6.7 m (Site E), 9.2 m (Site F), and 520 m (Site G) from the headwater stream respectively during each field campaign (Figures 1C,D). Sites A—F and site G are defined as the upper reach and lower reach of the headwater stream, respectively. The average slope of the stream reach from site A to F and site F to G is 24.7 and 7.8%, respectively. There is a waterfall immediately downstream of site F and site G is located downstream of the waterfall (Figure 1C). The sampling sites were selected to characterize the potential evasion of CO2 along the reach and for illustrating the longitudinal changes in water chemistry and C properties. Based on the climatic characteristics of Hong Kong, June to October is defined as the “wet season” and November to February as the “dry season” in this study. The meteorological data from the Hong Kong Observatory suggested that June to October on average account for >70% of the annual precipitation whereas this figure is only around 5% for November to February (Hong Kong Observatory, 2021).
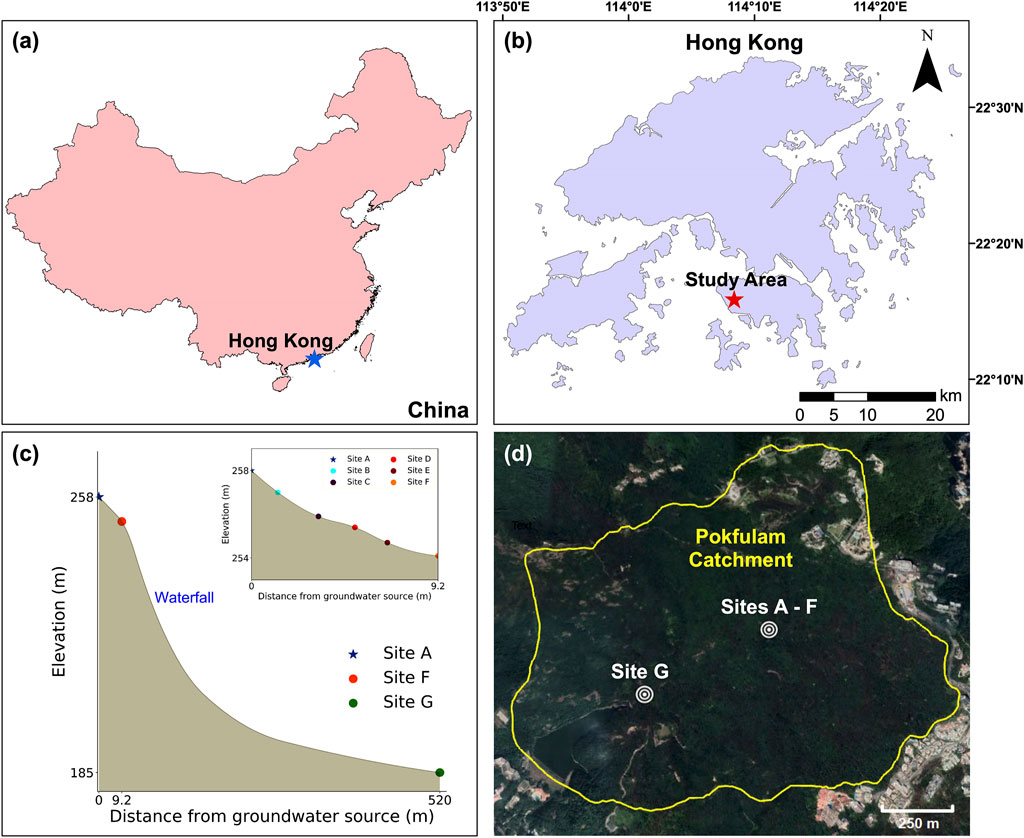
FIGURE 1. Location map of the study area (A,B), the schematic diagram showing the changes in elevation from site A to G (not scaled) (C), and the enlarged satellite image obtained from Google Earth showing the locations of the study sites, i.e., sites A—F and site G and the boundary of the Pokfulam catchment (D).
Sampling Procedures and Laboratory Analysis
At each site, approximately 500 ml of stream water was collected by using a polyethylene bottle. All the polyethylene bottles were thoroughly cleaned and sterilized prior to each field campaign and were filled completely with stream water without headspace. At Sites A and G, an additional 9 L of water was collected without headspace for analyzing the radon-222 concentration in the laboratory.
The basic water quality parameters, including pH, electroconductivity (EC), and DO at each sampling site was measured by using a ProDSS Multiparameter water quality meter (YSI, Yellow Springs, Ohio, United States). The water quality meter was calibrated with two pH buffer solutions (pH = 4.00 and 7.00) prior to each sampling work to ensure the accuracy of pH measurement. The water collected in the polyethylene bottle was filtered using 47 mm GF/C filter paper with a pore size of 1.2 μm (Whatman, Maidstone, United Kingdom), and alkalinity and dissolved organic carbon (DOC) were subsequently measured in the laboratory. 20 ml of sample was titrated with 0.05 M hydrochloric acid (HCl) to determine the alkalinity, using methyl orange as the indicator. A 60 ml subsample was prepared from the filtered water and acidified by concentrated sulfuric acid to pH < 2 for the measurement of DOC concentration. The determination of alkalinity and acidification of the subsample were carried out immediately upon the return of the laboratory and within the same day of the sampling. The acidified subsamples were then preserved in a refrigerator at 4°C until analysis, usually for 1 week. The DOC concentration was assessed by a TOC-L TOC analyzer (Shimadzu, Kyoto, Japan) using high-temperature combustion method with triple injections and an error of <2%. The standard used was 10 mg C L−1 potassium hydrogen phthalate. The stream discharge has been measured at sites A and G by using salt dilution gauging as reported in Moore (2004).
The pCO2 of the stream water at each site was measured by the headspace equilibrium method in-situ immediately after sampling (Campeau et al., 2014; Ran et al., 2021b). Briefly, 400 ml of water sample was collected in a reagent bottle with a capacity of 610 ml, while the remaining space was filled with ambient air, thus giving a water to headspace ratio of 1.91:1. The bottle was subsequently closed with a lid and shaken vigorously for at least 2 min for equilibrating the pCO2 between water and the headspace prior to the connection of a Li-850 CO2 analyzer (LI-COR, Lincoln, Nebraska, United States). The measurements of the headspace pCO2 were done in duplicate with repeatability better than 5%, and the values of original stream water pCO2 were accordingly computed from the headspace ratio, solubility constant of CO2, and temperature (Koschorreck et al., 2021). The calculation of average areal evasion flux of CO2 within the headwater stream was done in two steps. Firstly, the total amount of CO2 loss along the reach was estimated by the differences in the pCO2 between sites A and F and then multiply by the discharge at site A. Secondly, the average areal evasion flux of CO2 was calculated by dividing the total amount of CO2 loss along the reach by the estimated surface area of the reach.
The water collected at sites A and G was analyzed for radon-222 concentration with a RAD7 radon detector (Durridge, Billerica, Massachusetts, United States) in laboratory within 2 h after collection to determine the groundwater signature at sites A and G. It usually took approximately 30–45 min for the reading of radon-222 to reach equilibrium. The gap between the time of water collection and radon-222 measurement was considered unlikely to have a substantial effect on the experimental results because of the relatively short half-life of radon-222, which is about 3.8 days (Dimova et al., 2013).
Statistical Analyses
The statistical analyses were executed by IBM SPSS Statistics (Version 27). Apart from pH, the mean values of the parameters were presented as mean ± standard deviation. The two-sample independent t-test was conducted to test for the differences in the mean of variables between the dry season and wet season. The Pearson correlation coefficient (r) was selected to assess the strength of a linear association between two variables. The threshold of the p-value to be regarded as statistically significant was 0.05.
Results
Patterns of General Water Chemistry
The mean EC ranged from 43.9 to 50.4 μS cm−1, with a mean of 47.2 ± 1.4 μS cm−1 and exhibited a slight downward trend downstream from site A to F (Figure 2A). The mean EC at site F was 2.8 μS cm−1 lower than that of site A. The DO was always undersaturated with a range from 68.4 to 95.1% and a mean of 85.9 ± 6.3%, exhibiting a significant increasing trend downstream (Figure 2B). The mean DO at site F was 14.2% higher than that of site A, and stream water with DO < 80% was observed mainly at sites A and B. The stream water was acidic with pH ranging from 4.53 to 5.63 and a mean of 5.07, exhibiting a significant increasing trend downstream (Figure 2C). The mean pH value at site F was approximately on average 0.32 units higher than site A, and stream water with pH < 5 was mostly found at sites A, B, and C. The alkalinity did not display significant spatial differences along the reach, ranging from 125 to 206 μmol L−1, with a mean of 159 ± 22 μmol L−1 (Figure 2D). The DOC varied between 0.40 and 5.73 mg C L−1 with a mean of 1.38 ± 1.10 mg C L−1. There was a generally decreasing trend in DOC downstream during the dry season, with the mean DOC concentration decreased progressively from 1.15 mg C L−1 at site A to 0.48 mg C L−1 at site F, but this tendency was not observed during the wet season (Figure 2E). The discharge measured at site A ranged from 0.23 to 0.63 L s−1 with a mean of 0.37 ± 0.13 L s−1, and the average stream width was 0.2 m. The mean discharge during the wet and dry seasons was 0.42 ± 0.18 and 0.33 ± 0.04 L s−1, respectively (Figure 2F). Radon-222 concentrations at site A often reached high levels, exceeding 100,000 Bq m−3 in most sampling months with a mean of 101,720 ± 18,880 Bq m−3 (Figure 2G). The mean DOC was significantly lower in the dry season than in the wet season (p < 0.05, Two-sample t-test, Figure 2E), while no significant differences in EC, DO, pH, and alkalinity between the dry and wet seasons were found (p > 0.05, Two-sample t-test, Figures 2A–D).
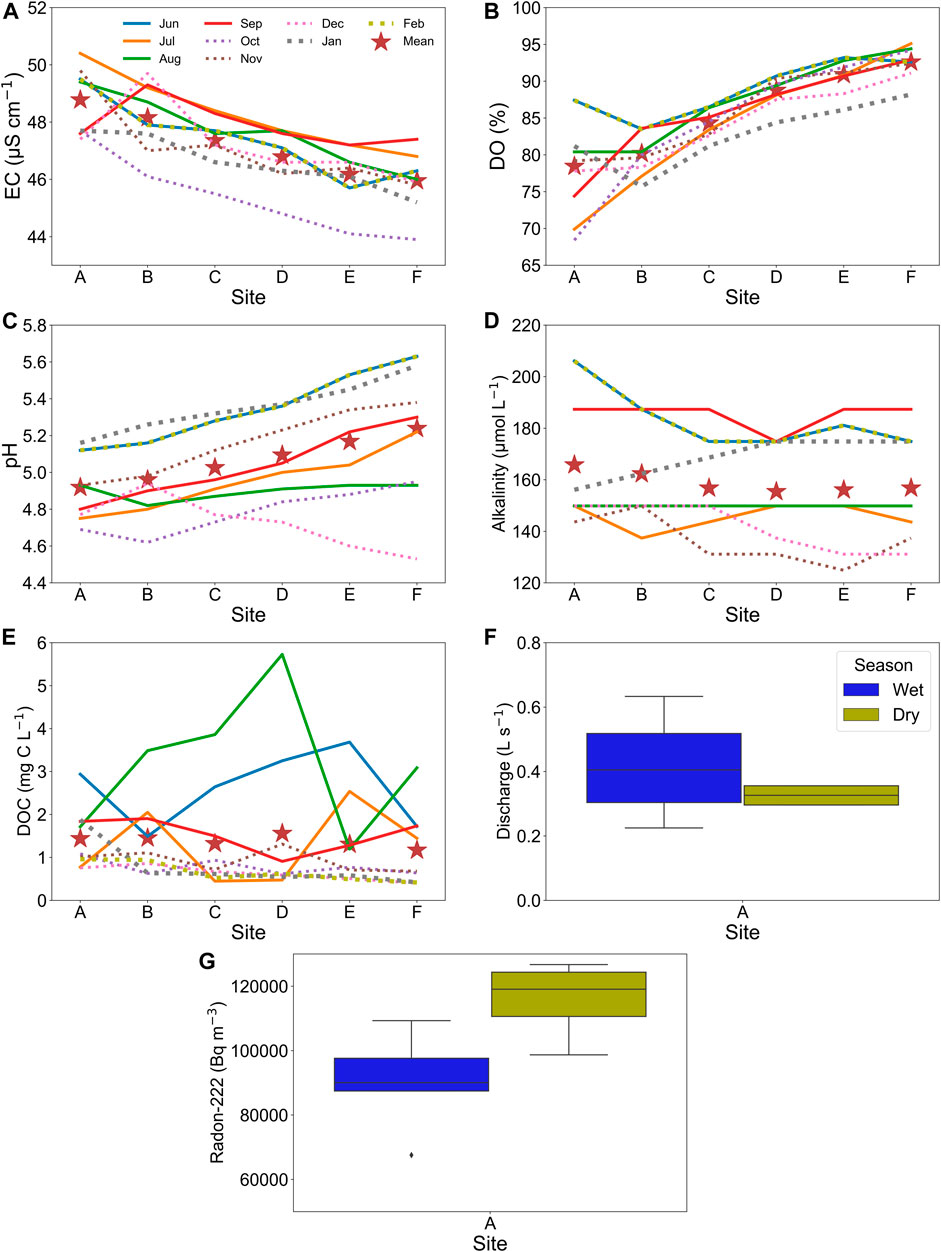
FIGURE 2. Plots summarizing the monthly water quality variables, including EC (A), DO (B), pH (C), alkalinity (D), and DOC (E) of sites A—F, and discharge (F) and radon-222 (G) of site A between wet and dry seasons in the headwater stream.
For site G, the mean EC, DO, pH, alkalinity, and DOC were 92.6 ± 12.9 μS cm−1, 100.5 ± 0.8%, 7.13, 211 ± 21 μmol L−1, and 1.15 ± 0.31 mg C L−1, respectively (Figures 3A–E). DO and DOC were significantly higher in the wet season than the dry season (p < 0.05, Two-sample t-test) while the seasonal differences of the remaining variables were not statistically significant (p > 0.05, Two-sample t-test). The mean EC, DO, pH, and alkalinity at site G were significantly higher than that of sites A—F (p < 0.05, Two-sample t-test). The mean radon-222 concentration at site G was 562 ± 168 Bq m−3.
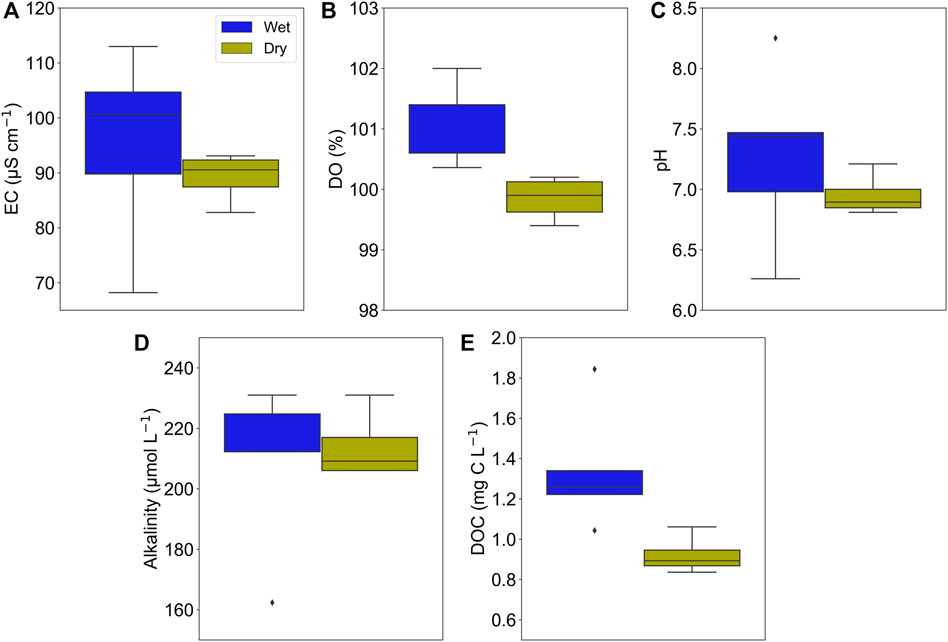
FIGURE 3. Boxplots summarizing the water quality variables (EC, DO, pH, alkalinity, and DOC) of site G between wet and dry season.
Temporal and Spatial Variations of Partial Pressure of Carbon Dioxide
The atmospheric pCO2 was determined to be 410 μatm based on the average value in 2020. The stream water at sites A—F was significantly supersaturated with CO2 with the pCO2 ranging from 7,890 to 46,750 μatm (mean: 22,940 ± 9,840 μatm), which was equal to supersaturation of 19–114 times with respect to atmospheric equilibrium (Figure 4). Concerning the temporal variability of the pCO2, the mean pCO2 during the wet season and dry season were 23,680 ± 9,810 and 22,010 ± 10,000 μatm, respectively, with the highest values in September and the lowest values in January (Figure 4). The mean pCO2 at sites A and F were 38,590 ± 5,780 and 10,820 ± 1,880 μatm, respectively, implying approximately 72% of the stream CO2 has been lost over the 9.2-m distance along the headwater stream (Figure 4). The pCO2 recorded at site G was significantly lower than that of sites A—F with the range of 533–1,590 μatm (mean: 778 ± 317 μatm) (Figure 4). The mean pCO2 at site G during the dry and wet seasons were 674 ± 114 and 861 ± 414 μatm, respectively.
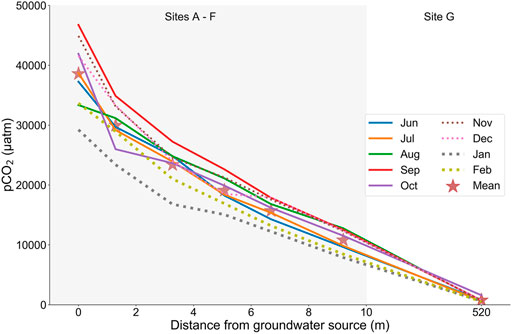
FIGURE 4. The longitudinal changes in pCO2 as a function of distance downstream from the groundwater source.
Relationship of Partial Pressure of Carbon Dioxide With Water Quality Parameters
For sites A—F, the pCO2 always exhibited a negative relationship with DO, and the correlations of pCO2 with DO and pH were significant in six out of 9 months with the magnitude of r higher than 0.9 (Table 1). The relationship of DOC with pCO2 was less prominent and consistent compared to that of DO and pH since the r ranged from −0.27 to 0.94, and the relationship was only significant during the dry season from December to February. The values of r of pCO2 with radon-222 and discharge were 0.33 and 0.01, respectively at site A, and both relationships were not statistically significant. For site G, the pCO2 had a positive significant relationship with discharge (p < 0.05, Table 2) while the relationships with DO, pH, DOC, and radon-222 were not statistically significant (p > 0.05, Table 2).
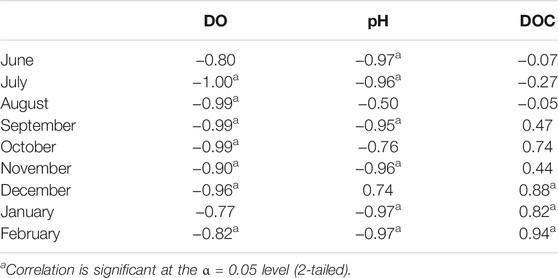
TABLE 1. The monthly values of Pearson correlation coefficient (r) of pCO2 with water quality variables in the headwater stream at sites A—F.
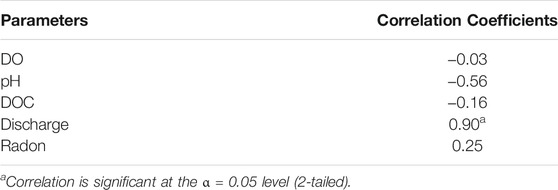
TABLE 2. The values of Pearson correlation coefficient (r) of pCO2 with water quality variables at site G of the headwater stream.
Discussion
General Chemistry of the Stream Water
Our measurements on the water quality parameters suggested that the chemistry of the headwater stream is heavily influenced by groundwater. Since the EC values remained fairly constant and low under all months of sampling, this indicated that the headwater stream had no signs of saltwater intrusion from the surrounding environment.
The general increase in pH along the downstream direction was a manifestation of the decrease in CO2 concentration due to degassing (Johnson et al., 2008). There was an occasion in which the pH at sites C—F remained low with the value lower than 5, and this might be attributable to the introduction of anthropogenic acidic substances into the stream or soil which resulted in a drop in the pH value (Figure 2C). As the concentration of DO also increased downstream owing to intense gas exchange (Dick et al., 2016), this was likely the reason for the significant positive association between pH and DO in the headwater stream. However, there have been a couple of instances with DO showing a slight decrease from site A to site B, and we suggest that the decomposition of OC along this distance may be a possible reason for this trend. Our measurements were consistent with the theoretical correlation between alkalinity and pH since an increase in pH leads to the production of chemically sequestered bicarbonate ions, a component of alkalinity (Piñol and Avila, 1992; Abril et al., 2015). There was also a general decreasing trend in the DOC concentration within sites A—F of the headwater stream during the dry season (Figure 2E), potentially due to in-stream metabolic processes such as mineralization of organic carbon (OC) and by the dilution of DOC-depleted water downstream (Duan et al., 2017). However, this decreasing trend was obscured in the wet season likely because of the capability of heavy rainfall events displacing terrestrially derived OC from the upstream catchment and riparian areas along the reach, which also led to up to more than twofold higher levels of DOC in the headwater stream during the wet season (Figure 2E) (Pumpanen et al., 2014; Panton et al., 2020). The generally higher discharge during the wet season might also reduce the water residence time which had the potential to impact the duration of OC mineralization that occurred along the reach (Wickland et al., 2007). Additionally, the variabilities of DOC were higher in the wet season than in the dry season (Figure 2E), especially at sites A—F, potentially owing to the sporadic and incidental nature of heavy rainfall events that brought about an increase in DOC levels.
Carbon Dioxide Exchange Dynamics
The pCO2 was mostly two orders of magnitude higher than the ambient air at sites A—F along the headwater stream, and it was even >100 times higher at sites immediately adjacent to the groundwater source (Figure 4), indicating the reach was a consistent source of CO2. This observation was consistent with the pCO2 values obtained from headwater stream networks elsewhere in the world (Dawson et al., 2002; Crawford et al., 2013; Winterdahl et al., 2016; Crawford et al., 2017; Li et al., 2021). Because the mean pCO2 value recorded at site A was in good agreement with the mean value (51,900 ± 1,600 ppmv) of Amazonian deep soil for emergent groundwater reported previously (Johnson et al., 2008) and fell within the range of the groundwater pCO2 in Swiss mountain catchments and Ganges River catchment (Horgby et al., 2019b; Manaka et al., 2019), it is of high confidence that the high pCO2 in the headwater stream is contributed by the CO2-enriched groundwater. The elevated pCO2 level in groundwater was likely the result of root respiration as well as the microbial respiration of organic matter and its decomposition in surrounding soils (Crawford et al., 2013; Deirmendjian and Abril, 2018; Horgby et al., 2019a; Manaka et al., 2019). The C dynamics of headwater streams located in upper reaches are more profoundly influenced by terrestrially sourced C when compared with large rivers, and therefore they are less sensitive to in-stream metabolism (Johnson et al., 2008; Hotchkiss et al., 2015; Argerich et al., 2016; Deirmendjian and Abril, 2018; Wang et al., 2021). Furthermore, a larger proportion of stream water was in contact with adjacent soil horizons, allowing a stronger hydrological connection between CO2-rich soils and surface water, contributing to high pCO2 values in groundwater (Crawford et al., 2013; Schneider et al., 2020). Because the study area is dominated by volcanic rocks without extensive coverage of carbonate rocks, the dissolution of carbonate rocks is highly unlikely to occur and thus cannot be the underlying cause of the elevated pCO2 in the groundwater (Duvert et al., 2018; Wang et al., 2021).
The rate of decline in pCO2 along the reach was analogous to the Johnson et al. (2008) study, which developed a model showing a 77% reduction in dissolved CO2 at 9.2 m from the groundwater spring and was even more rapid than a headwater stream in southwestern France, which exhibited a 70% loss of pCO2 at 40 m downstream of the groundwater resurgence (Deirmendjian and Abril, 2018). The median percentage of CO2 loss in the first 100 m from groundwater sources in 15 streams worldwide covering tropical, temperate, and boreal catchments was 76% (Duvert et al., 2018). Our pCO2 measurement unequivocally indicated the evasion of CO2 along the reach was very substantial and occurred immediately upon entry of the groundwater into the surface stream (Pu et al., 2019). This observation was particularly salient because it reflected the presence of very active gas exchange triggered by strong turbulence in this high gradient headwater stream fed by groundwater and illustrated that groundwater with exceptionally high CO2 content had a diminishing effect on stream pCO2 values with increasing distance downstream (Duvert et al., 2018).
Additionally, our fieldwork results indicated the evasion of CO2 along the stream via outgassing was responsible for the gradual equilibration of CO2 in the stream with the atmosphere (Duvert et al., 2018; Schneider et al., 2020). The simultaneous increase in the DO concentrations downstream further corroborated the strong and rapid gas exchange in this headwater stream since its turbulent nature allowed oxygen to seep and dissolve into the stream water (Dick et al., 2016), and also permitted CO2 to be released into the atmosphere, yielding the aforementioned pCO2 and DO trend. In addition, DO and pCO2 measurements at site G further confirm that water-air gas exchange to reach equilibrium, and complete evasion of groundwater CO2, was likely to be achieved within 520 m of the groundwater source since the DO consistently reached saturation regardless of the sampling month, while pCO2 fell to the level similar to the ambient level. This distance was even shorter than that of a previous study which suggested that a majority of the CO2 in headwater streams sourced from groundwater might be degassed within a few kilometers (Davidson et al., 2010). We argue that this exceptionally large decline in the pCO2 level might be attributed to the presence of a waterfall between sites F and G. Several previous studies have elaborated on the importance of waterfalls in the emission of CO2 from freshwater ecosystems due to their abilities in triggering turbulence and favoring gaseous exchange through bubble-mediated transfer, which in turn produced a significant decrease (>50%) in pCO2 after the stream water passing through waterfalls (Teodoru et al., 2015; Leibowitz et al., 2017; Looman et al., 2021). Because the CO2 dynamics at downstream locations could be driven by interactions of multiple biogeochemical processes, such as community respiration, primary production, precipitation of minerals, and input of nutrients and CO2 from immediate surroundings (Davidson et al., 2010; Atkins et al., 2013; Peter et al., 2014; Duvert et al., 2018), the precise quantification of the fraction of groundwater-derived CO2 remained at site G remains an arduous task. Yet, the enormous decrease in radon-222 concentration by > 99% from site A to G as well as the observed DO and pCO2 longitudinal trends at least demonstrated that the stream water has lost most of its groundwater signature over this distance.
Our longitudinal pCO2 results were consistent with the findings observed by Schneider et al. (2020) study, who showed a higher pCO2 in the upper portion of the stream and declined in the lower reaches, although our study reach was even shorter than the study and the pCO2 at the source of groundwater was an order of magnitude higher. Another similar study also reported that the significant drop of the differences between the concentration of CO2 in the stream water and the ambient air in first order streams could be ascribed to highly efficient gas exchange resulting in significant CO2 loss along the reach (Schelker et al., 2016). Based on the above comparisons, our research data revealed that the evasion of CO2 along the headwater stream is governed by groundwater input and extremely likely to play a pivotal role in the C dynamics (Deirmendjian et al., 2018). Assuming the decline in pCO2 derived from groundwater along the reach was solely attributable to the evasion of CO2 with the contribution of in-stream metabolism being negligible, our measurements on pCO2 and discharge data estimated that the average areal evasion flux of CO2 from site A to F and from site F to G amounted to 19,000 and 140 mmol m−2 d−1, respectively. The former flux was two orders of magnitude higher than previous CO2 emission fluxes in stream networks that range from 180 to 920 mmol m−2 d−1 (Butman and Raymond, 2011; Crawford et al., 2013; Crawford et al., 2014; Wang et al., 2017). Therefore, our measurement indicated the water surface of the upstream region of a catchment is capable of emitting significantly more CO2 per unit area, in agreement with a groundwater study conducted in an alpine stream network which highlighted the CO2 emissions from mountainous streams in zones of local groundwater upwelling can outweigh those located in lowland areas (Horgby et al., 2019a). Despite the small water surface area, the CO2 emission from the first few tens of meters of a groundwater-fed headwater stream is potentially huge and must be considered for obtaining an accurate estimation of the reach-scale CO2 flux. Waterfalls and cascades along stream reach, on the other hand, may also facilitate the outgassing of CO2 and their contributions to reach- and catchment-scale CO2 emissions merit further investigation.
Environmental Factors Affecting Carbon Dioxide Dynamics
The CO2 dynamics within the headwater stream were found to be statistically correlated with a few water quality and environmental parameters. Firstly, the significant negative correlation between the DO and pCO2 level in most sampling months (Table 1) at sites A—F was consistent with the Li et al. (2021) study covering global rivers, and we anticipated this negative correlation was likely a consequence of rapid gas exchange since the turbulent nature of this headwater stream permits outgassing of a significant amount of CO2, causing a large decrease in the pCO2 within a short distance. At the same time, the intense turbulence triggered by steep slopes also resulted in a high rate of reaeration along the stream which led to the rapid replenishment of oxygen from the ambient air and thus a significant increase in DO levels (Huang et al., 2017). At site G, the DO levels were approximately similar regardless of the sampling month, so this could be a potential explanation for the lack of association between dissolved oxygen and pCO2 levels (Table 2). As anticipated, the significant negative pH-pCO2 relationship (Table 1) is likely to be attributed to the dissolution of CO2 in water lowering the pH.
The lack of strong and consistent association between pCO2 with the DOC concentration in the headwater stream (Tables 1 and 2) has also been documented in previous studies conducted in different parts of the world with varying climate conditions (Nydahl et al., 2017; Horgby et al., 2019a; Luo et al., 2019), and this reflected the mineralization of organic C is unlikely to be the primary source of pCO2 in this headwater stream (Winterdahl et al., 2016). Although DOC concentration has been hypothesized to be positively associated with pCO2 level in riverine systems owing to the in-situ oxidation of organic matter (Nydahl et al., 2017), the rapid outgassing of CO2 in this system may attenuate the strength of the pCO2—DOC relationship along the reach, reflecting the fact that the level of DOC concentration might play an auxiliary role in modulating pCO2 while the intense gas exchange was still the fundamental factor in controlling the C dynamics. In addition, the increase in DOC is occasionally associated with an increase in precipitation, but the dilution of stream water CO2 is possible when precipitation increases, thus obscuring the relationship between the two variables (Luo et al., 2019). Our results support the existence of this precipitation-induced dilution effect since significant positive pCO2—DOC relationships were only recorded during dry season when the precipitation was low (Table 1). Apart from the quantity of DOC, it has also been suggested the chemical properties and degradability of DOC had a role to play in determining the pCO2 level, while these properties could be impacted by changes in vegetation type and water residence time (Wickland et al., 2007; Dinsmore et al., 2013; Luo and Li, 2021). Thus, a more specific chemical analysis on the properties of DOC in streams is likely to better constrain the processes leading to pCO2 variabilities in headwater streams. Nonetheless, the results of our current study do not unambiguously preclude the possibility of organic C mineralization in influencing pCO2 level within the headwater stream, though its effect should be appreciably less influential than that of longitudinal evasive CO2 loss.
Furthermore, we also found that the CO2 dynamics of the headwater stream might be influenced by the ambient air temperature since there was a positive relationship between the monthly pCO2 at site A and air temperature (r = 0.44, Figure 5). In addition, January and September were the 2 months with the lowest and highest average pCO2 values in the stream, while the air temperature was the lowest and second highest (Figure 5). Thus, we suggested that the observed seasonal variabilities in pCO2 could be ascribable to the temperature-dependent metabolic activities because the higher temperature in summer months might have facilitated heterotrophic and root respiration in soil, and the downward diffusion of soil CO2 will lead to a surge in pCO2 values within the studied headwater stream (Jones and Mulholland, 1998; Deirmendjian and Abril, 2018). This has also been observed in a regional stream study that reported significantly higher pCO2 levels in summer than spring in headwaters (Jones and Mulholland, 1998). Although the effect of stormwater dilution on groundwater CO2 has been reported previously (Deirmendjian and Abril, 2018), it might not be a significant factor in regulating the pCO2 levels in the section of the headwater stream close to the groundwater source because of an absence of the relationships between the discharge and pCO2 at site A. In contrast, there was a significant positive correlation of pCO2 with discharge at site G (Table 2), and the cause of this relationship might be independent of DOC input since there was no significant relationship between discharge and DOC. Thus, it is hypothesized that such a relationship in the lower reach could be attributable to the increased runoff from the upper catchment area that was enriched in CO2 (Dinsmore et al., 2013).
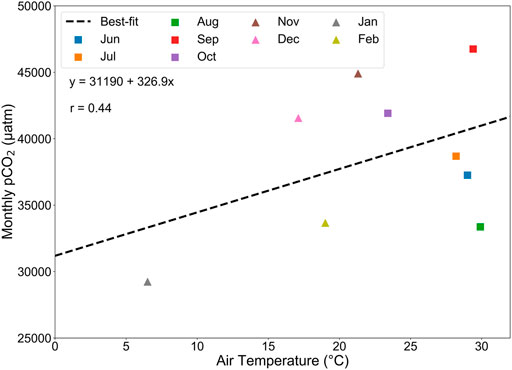
FIGURE 5. The relationship between monthly pCO2 at site A and ambient temperature. The dashed line represents the best-fit line of the regression.
Implications for Quantifying Carbon Dioxide Emissions From Stream Networks
Based on our sampling efforts, a close linkage was found between the groundwater and stream networks (Duvert et al., 2018), and the contribution of CO2 from groundwater and outgassing of CO2 along the reach was considered to be the primary determinants of C dynamics in this headwater stream. Although other environmental variables including DOC concentration and ambient air temperature might also be capable of regulating pCO2 levels in the studied headwater stream, the changes were substantially more modest as compared to the longitudinal changes in pCO2, even at distances of less than 10 m. Additionally, the influence of groundwater in pCO2 within the catchment was almost varnished at a distance of up to 520 m. This finding is prominent and highlights the necessity of future works focusing on exploring the possibility of other high-gradient headwater streams fed by groundwater having similarly high outgassing rates. On top of that, our study demonstrated that the upstream section of headwaters fed by groundwater are conceivable hotspots of CO2 emission since the flux can be several orders of magnitude higher than the previously reported stream networks with varying topography and climatic conditions. Because of the rapid gas exchange in high-gradient headwater streams, strong spatial heterogeneities in C dynamics are also occasionally detectable within a catchment. Therefore, the resulting estimates are likely to underestimate the true C flux if these headwater systems are not duly and adequately accounted for when quantifying the landscape-scale C budget (Deirmendjian and Abril, 2018; Aho and Raymond, 2019). The geomorphological characteristics of headwater streams, such as stream bed roughness and slope, are also subject to spatial heterogeneities that result in localized zones with strong turbulence and hence evasion (Duvert et al., 2018), and our results emphatically showed that the physical and biochemical processes controlling pCO2 may differ in headwater streams fed by groundwater and its downstream section. Therefore, a more intensive study of the variations of these processes and mechanisms with respect to changes in location within a headwater stream is a promising direction for future research on the C dynamics.
Conclusion
Our sampling on a high-gradient headwater stream fed by groundwater in Hong Kong indicated that headwater streams are prominent emitters of CO2. The pCO2 levels of the upper reaches of the headwater stream were 19–114 times higher than the atmosphere and dropped by approximately 72% over a distance shorter than 10 m from the groundwater resurgence, indicating that a substantial share of the CO2 derived from groundwater has been outgassed into the atmosphere within such a short distance. We also found that the groundwater signature and the effect of elevated CO2 from groundwater could be completely dissipated at a distance of up to 520 m based on the pCO2, DO, and radon-222 measurements at the lower reach of the headwater stream. Meanwhile, other water quality parameters, such as DO, DOC, EC, and pH, also exhibited longitudinal changes within the headwater stream, and pCO2 was found to be significantly associated with several water quality parameters including pH and DO. Although other environmental drivers, for example, the ambient temperature and the availability of DOC for metabolic activities may also modulate the pCO2 pattern, the intense gas exchange triggered by turbulence remains the overriding factor controlling the C dynamics in this headwater stream. The existence of waterfalls and cascades within a stream network may also influence the C dynamics since they could exert an accelerating effect in CO2 outgassing. Despite the generally small water surface area covered by a single headwater stream, this study suggests that high-gradient headwater streams are hotspots of CO2 emissions. The omission of these systems may introduce significant biases in estimating catchment-scale and regional CO2 flux from aquatic ecosystems, thus illustrating the importance of sampling in the immediate vicinity of groundwater emergence. We hope this work offers new knowledge that will lead to more accurate and comprehensive assessments of CO2 emissions from headwater streams in the humid subtropical region and render the potential of upscaling the estimates to broader regions with similar climatic and land cover features.
Data Availability Statement
The original contributions presented in the study are included in the article/Supplementary Material, further inquiries can be directed to the corresponding author.
Author Contributions
CC, FL, and LR contributed to conceptualization and design of the study. CC, CT, and BL performed the fieldworks and experiments. CC performed the statistical analysis and wrote the manuscript with inputs from all authors. LR supervised the project. All authors contributed to manuscript revision.
Funding
This study was financially supported by the Research Grants Council of Hong Kong (Grants: 17300619 and 27300118), the National Natural Science Foundation of China (Grant: 41807318), and the Hui Oi-Chow Trust Fund (Grant: 263690564).
Conflict of Interest
The authors declare that the research was conducted in the absence of any commercial or financial relationships that could be construed as a potential conflict of interest.
The handling Editor declared a past co-authorship with one of the authors (LR).
Publisher’s Note
All claims expressed in this article are solely those of the authors and do not necessarily represent those of their affiliated organizations, or those of the publisher, the editors, and the reviewers. Any product that may be evaluated in this article, or claim that may be made by its manufacturer, is not guaranteed or endorsed by the publisher.
Acknowledgments
Special thanks are also given to Jeannette Liu, Yu Ching Ip, and Fong Ching Ma for their contributions to the fieldwork.
References
Abril, G., Bouillon, S., Darchambeau, F., Teodoru, C. R., Marwick, T. R., Tamooh, F., et al. (2015). Technical Note: Large Overestimation of pCO2 Calculated from pH and Alkalinity in Acidic, Organic-Rich Freshwaters. Biogeosciences 12 (1), 67–78. doi:10.5194/bg-12-67-2015
Aho, K. S., and Raymond, P. A. (2019). Differential Response of Greenhouse Gas Evasion to Storms in Forested and Wetland Streams. J. Geophys. Res. Biogeosci. 124 (3), 649–662. doi:10.1029/2018JG004750
Argerich, A., Haggerty, R., Johnson, S. L., Wondzell, S. M., Dosch, N., Corson‐Rikert, H., et al. (2016). Comprehensive Multiyear Carbon Budget of a Temperate Headwater Stream. J. Geophys. Res. Biogeosci. 121 (5), 1306–1315. doi:10.1002/2015JG003050
Atkins, M. L., Santos, I. R., Ruiz-Halpern, S., and Maher, D. T. (2013). Carbon Dioxide Dynamics Driven by Groundwater Discharge in a Coastal Floodplain Creek. J. Hydrol. 493, 30–42. doi:10.1016/j.jhydrol.2013.04.008
Benstead, J. P., and Leigh, D. S. (2012). An Expanded Role for River Networks. Nat. Geosci 5 (10), 678–679. doi:10.1038/ngeo1593
Bernal, S., von Schiller, D., Sabater, F., and Martí, E. (2013). Hydrological Extremes Modulate Nutrient Dynamics in Mediterranean Climate Streams across Different Spatial Scales. Hydrobiologia 719 (1), 31–42. doi:10.1007/s10750-012-1246-2
Butman, D., and Raymond, P. A. (2011). Significant Efflux of Carbon Dioxide from Streams and Rivers in the United States. Nat. Geosci 4 (12), 839–842. doi:10.1038/ngeo1294
Campeau, A., Lapierre, J.-F., Vachon, D., and del Giorgio, P. A. (2014). Regional Contribution of CO2 and CH4 fluxes from the Fluvial Network in a lowland Boreal Landscape of Québec. Glob. Biogeochem. Cycles 28 (1), 57–69. doi:10.1002/2013GB004685
Cheung, P. K., Hung, P. L., and Fok, L. (2019). River Microplastic Contamination and Dynamics upon a Rainfall Event in Hong Kong, China. Environ. Process. 6 (1), 253–264. doi:10.1007/s40710-018-0345-0
Crawford, J. T., Lottig, N. R., Stanley, E. H., Walker, J. F., Hanson, P. C., Finlay, J. C., et al. (2014). CO2 and CH4 emissions from Streams in a lake-rich Landscape: Patterns, Controls, and Regional Significance. Glob. Biogeochem. Cycles 28 (3), 197–210. doi:10.1002/2013gb004661
Crawford, J. T., Stanley, E. H., Dornblaser, M. M., and Striegl, R. G. (2017). CO2 Time Series Patterns in Contrasting Headwater Streams of North America. Aquat. Sci. 79 (3), 473–486. doi:10.1007/s00027-016-0511-2
Crawford, J. T., Striegl, R. G., Wickland, K. P., Dornblaser, M. M., and Stanley, E. H. (2013). Emissions of Carbon Dioxide and Methane from a Headwater Stream Network of interior Alaska. J. Geophys. Res. Biogeosci. 118 (2), 482–494. doi:10.1002/jgrg.20034
Davidson, E. A., Figueiredo, R. O., Markewitz, D., and Aufdenkampe, A. K. (2010). Dissolved CO2 in Small Catchment Streams of Eastern Amazonia: A Minor Pathway of Terrestrial Carbon Loss. J. Geophys. Res. 115 (G4). doi:10.1029/2009JG001202
Dawson, J. J. C., Billett, M. F., Neal, C., and Hill, S. (2002). A Comparison of Particulate, Dissolved and Gaseous Carbon in Two Contrasting upland Streams in the UK. J. Hydrol. 257 (1), 226–246. doi:10.1016/S0022-1694(01)00545-5
Deirmendjian, L., and Abril, G. (2018). Carbon Dioxide Degassing at the Groundwater-Stream-Atmosphere Interface: Isotopic Equilibration and Hydrological Mass Balance in a sandy Watershed. J. Hydrol. 558, 129–143. doi:10.1016/j.jhydrol.2018.01.003
Deirmendjian, L., Loustau, D., Augusto, L., Lafont, S., Chipeaux, C., Poirier, D., et al. (2018). Hydro-ecological Controls on Dissolved Carbon Dynamics in Groundwater and export to Streams in a Temperate pine forest. Biogeosciences 15 (2), 669–691. doi:10.5194/bg-15-669-2018
Dick, J. J., Soulsby, C., Birkel, C., Malcolm, I., and Tetzlaff, D. (2016). Continuous Dissolved Oxygen Measurements and Modelling Metabolism in Peatland Streams. PLOS ONE 11 (8), e0161363. doi:10.1371/journal.pone.0161363
Dimova, N. T., Burnett, W. C., Chanton, J. P., and Corbett, J. E. (2013). Application of Radon-222 to Investigate Groundwater Discharge into Small Shallow Lakes. J. Hydrol. 486, 112–122. doi:10.1016/j.jhydrol.2013.01.043
Dinsmore, K. J., Wallin, M. B., Johnson, M. S., Billett, M. F., Bishop, K., Pumpanen, J., et al. (2013). Contrasting CO2 concentration Discharge Dynamics in Headwater Streams: A Multi-Catchment Comparison. J. Geophys. Res. Biogeosci. 118 (2), 445–461. doi:10.1002/jgrg.20047
Downing, J., Cole, J. J., Duarte, C. M., Middelburg, J. J., Melack, J. M., Prairie, Y. T., et al. (2012). Global Abundance and Size Distribution of Streams and Rivers. Iw 2 (4), 229–236. doi:10.5268/IW-2.4.502
Duan, S., He, Y., Kaushal, S. S., Bianchi, T. S., Ward, N. D., and Guo, L. (2017). Impact of Wetland Decline on Decreasing Dissolved Organic Carbon Concentrations along the Mississippi River Continuum. Front. Mar. Sci. 3 (280). doi:10.3389/fmars.2016.00280
Duvert, C., Butman, D. E., Marx, A., Ribolzi, O., and Hutley, L. B. (2018). CO2 Evasion along Streams Driven by Groundwater Inputs and Geomorphic Controls. Nat. Geosci 11, 813–818. doi:10.1038/s41561-018-0245-y
Fletcher, C. J. N. (1997). The Geology of Hong Kong. J. Geol. Soc. 154 (6), 999–1000. doi:10.1144/gsjgs.154.6.0999
Gómez-Gener, L., von Schiller, D., Marcé, R., Arroita, M., Casas-Ruiz, J. P., Staehr, P. A., et al. (2016). Low Contribution of Internal Metabolism to Carbon Dioxide Emissions along Lotic and Lentic Environments of a Mediterranean Fluvial Network. J. Geophys. Res. Biogeosci. 121 (12), 3030–3044. doi:10.1002/2016jg003549
Hong Kong Observatory (2021). Daily Data for Single Element. [Online]. Available: http://www.hko.gov.hk/en/cis/dailyElement.htm [Accessed] .
Horgby, Å., Boix Canadell, M., Ulseth, A. J., Vennemann, T. W., and Battin, T. J. (2019a). High‐Resolution Spatial Sampling Identifies Groundwater as Driver of CO2 Dynamics in an Alpine Stream Network. J. Geophys. Res. Biogeosci. 124 (7), 1961–1976. doi:10.1029/2019JG005047
Horgby, Å., Segatto, P. L., Bertuzzo, E., Lauerwald, R., Lehner, B., Ulseth, A. J., et al. (2019b). Unexpected Large Evasion Fluxes of Carbon Dioxide from Turbulent Streams Draining the World's Mountains. Nat. Commun. 10 (1), 4888. doi:10.1038/s41467-019-12905-z
Hotchkiss, E. R., Hall Jr, R. O., Sponseller, R. A., Butman, D., Klaminder, J., Laudon, H., et al. (2015). Sources of and Processes Controlling CO2 Emissions Change with the Size of Streams and Rivers. Nat. Geosci 8 (9), 696–699. doi:10.1038/ngeo2507
Huang, J., Yin, H., Chapra, S., and Zhou, Q. (2017). Modelling Dissolved Oxygen Depression in an Urban River in China. Water 9 (7), 520. doi:10.3390/w9070520
Johnson, M. S., Lehmann, J., Riha, S. J., Krusche, A. V., Richey, J. E., Ometto, J. P. H. B., et al. (2008). CO2 efflux from Amazonian Headwater Streams Represents a Significant Fate for Deep Soil Respiration. Geophys. Res. Lett. 35 (17). doi:10.1029/2008GL034619
Jones, J. B., and Mulholland, P. J. (1998). Influence of Drainage basin Topography and Elevation on Carbon Dioxide and Methane Supersaturation of Stream Water. Biogeochemistry 40 (1), 57–72. doi:10.1023/A:1005914121280
Koschorreck, M., Prairie, Y. T., Kim, J., and Marcé, R. (2021). Technical Note: CO2 Is Not like CH4 - Limits of and Corrections to the Headspace Method to Analyse pCO2 in Fresh Water. Biogeosciences 18 (5), 1619–1627. doi:10.5194/bg-18-1619-2021
Leibowitz, Z. W., Brito, L. A. F., De Lima, P. V., Eskinazi-Sant’Anna, E. M., and Barros, N. O. (2017). Significant Changes in Water pCO2 Caused by Turbulence from Waterfalls. Limnologica 62, 1–4. doi:10.1016/j.limno.2016.09.008
Li, M., Peng, C., Zhang, K., Xu, L., Wang, J., Yang, Y., et al. (2021). Headwater Stream Ecosystem: an Important Source of Greenhouse Gases to the Atmosphere. Water Res. 190, 116738. doi:10.1016/j.watres.2020.116738
Looman, A., Maher, D. T., and Santos, I. R. (2021). Carbon Dioxide Hydrodynamics along a wetland-lake-stream-waterfall Continuum (Blue Mountains, Australia). Sci. Total Environ. 777, 146124. doi:10.1016/j.scitotenv.2021.146124
Luo, J., Li, S., Ni, M., and Zhang, J. (2019). Large Spatiotemporal Shifts of CO2 Partial Pressure and CO2 Degassing in a Monsoonal Headwater Stream. J. Hydrol. 579, 124135. doi:10.1016/j.jhydrol.2019.124135
Luo, J., and Li, S. (2021). Optical Properties of Dissolved Organic Matter in a Monsoonal Headwater Stream, China: Insights for Structure, Source and Riverine pCO2. J. Clean. Prod. 282, 124545. doi:10.1016/j.jclepro.2020.124545
Manaka, T., H. M. Zakir Hossain, H. M. Z., Yoshimura, T., Suzuki, A., and Kawahata, H. (2019). Monthly Changes in pCO2 in the Ganges River: Implications for Carbon Release from Soil to the Atmosphere via Inland Waters. J. Agric. Meteorol. 75 (1), 47–55. doi:10.2480/agrmet.D-18-00007
Marx, A., Dusek, J., Jankovec, J., Sanda, M., Vogel, T., van Geldern, R., et al. (2017). A Review of CO2 and Associated Carbon Dynamics in Headwater Streams: A Global Perspective. Rev. Geophys. 55 (2), 560–585. doi:10.1002/2016rg000547
Maurice, L., Rawlins, B. G., Farr, G., Bell, R., and Gooddy, D. C. (2017). The Influence of Flow and Bed Slope on Gas Transfer in Steep Streams and Their Implications for Evasion of CO2. J. Geophys. Res. Biogeosci. 122 (11), 2862–2875. doi:10.1002/2017jg004045
Moore, R. D. (2004). Introduction to Salt Dilution Gauging for Streamflow Measurement Part 2: Constant-Rate Injection. Streamline Watershed Manage. Bull. 8 (1), 11–15.
Mullinger, N. J., Binley, A. M., Pates, J. M., and Crook, N. P. (2007). Radon in Chalk Streams: Spatial and Temporal Variation of Groundwater Sources in the Pang and Lambourn Catchments, UK. J. Hydrol. 339 (3), 172–182. doi:10.1016/j.jhydrol.2007.03.010
Nydahl, A. C., Wallin, M. B., and Weyhenmeyer, G. A. (2017). No Long-Term Trends in pCO2 Despite Increasing Organic Carbon Concentrations in Boreal Lakes, Streams, and Rivers. Glob. Biogeochem. Cycles 31 (6), 985–995. doi:10.1002/2016GB005539
Panton, A., Couceiro, F., Fones, G. R., and Purdie, D. A. (2020). The Impact of Rainfall Events, Catchment Characteristics and Estuarine Processes on the export of Dissolved Organic Matter from Two lowland Rivers and Their Shared Estuary. Sci. Total Environ. 735, 139481. doi:10.1016/j.scitotenv.2020.139481
Peter, H., Singer, G. A., Preiler, C., Chifflard, P., Steniczka, G., and Battin, T. J. (2014). Scales and Drivers of temporal pCO2 dynamics in an Alpine Stream. J. Geophys. Res. Biogeosci. 119 (6), 1078–1091. doi:10.1002/2013JG002552
Piñol, J., and Avila, A. (1992). Streamwater pH, Alkalinity, pCO2 and Discharge Relationships in Some Forested Mediterranean Catchments. J. Hydrol. 131 (1), 205–225. doi:10.1016/0022-1694(92)90218-K
Pu, J., Li, J., Zhang, T., Xiong, X., and Yuan, D. (2019). High Spatial and Seasonal Heterogeneity of pCO2 and CO2 Emissions in a Karst Groundwater-Stream Continuum, Southern China. Environ. Sci. Pollut. Res. 26 (25), 25733–25748. doi:10.1007/s11356-019-05820-9
Pumpanen, J., Lindén, A., Miettinen, H., Kolari, P., Ilvesniemi, H., Mammarella, I., et al. (2014). Precipitation and Net Ecosystem Exchange Are the Most Important Drivers of DOC Flux in upland Boreal Catchments. J. Geophys. Res. Biogeosci. 119 (9), 1861–1878. doi:10.1002/2014JG002705
Ran, L., Butman, D. E., Battin, T. J., Yang, X., Tian, M., Duvert, C., et al. (2021a). Substantial Decrease in CO2 Emissions from Chinese Inland Waters Due to Global Change. Nat. Commun. 12 (1), 1730. doi:10.1038/s41467-021-21926-6
Ran, L., Shi, H., and Yang, X. (2021b). Magnitude and Drivers of CO2 and CH4 Emissions from an Arid/semiarid River Catchment on the Chinese Loess Plateau. J. Hydrol. 598, 126260. doi:10.1016/j.jhydrol.2021.126260
Schelker, J., Singer, G. A., Ulseth, A. J., Hengsberger, S., and Battin, T. J. (2016). CO2 Evasion from a Steep, High Gradient Stream Network: Importance of Seasonal and Diurnal Variation in Aquatic pCO2 and Gas Transfer. Limnol. Oceanogr. 61 (5), 1826–1838. doi:10.1002/lno.10339
Schneider, C. L., Herrera, M., Raisle, M. L., Murray, A. R., Whitmore, K. M., Encalada, A. C., et al. (2020). Carbon Dioxide (CO2) Fluxes from Terrestrial and Aquatic Environments in a High‐Altitude Tropical Catchment. J. Geophys. Res. Biogeosci. 125 (8), e2020JG005844. doi:10.1029/2020JG005844
Teodoru, C. R., Nyoni, F. C., Borges, A. V., Darchambeau, F., Nyambe, I., and Bouillon, S. (2015). Dynamics of Greenhouse Gases (CO2, CH2, N2O) along the Zambezi River and Major Tributaries, and Their Importance in the Riverine Carbon Budget. Biogeosciences 12 (8), 2431–2453. doi:10.5194/bg-12-2431-2015
Ulseth, A. J., Hall, R. O., Boix Canadell, M., Madinger, H. L., Niayifar, A., and Battin, T. J. (2019). Distinct Air-Water Gas Exchange Regimes in Low- and High-Energy Streams. Nat. Geosci. 12, 259–263. doi:10.1038/s41561-019-0324-8
Wallin, M. B., Grabs, T., Buffam, I., Laudon, H., Ågren, A., Öquist, M. G., et al. (2013). Evasion of CO2 from Streams - the Dominant Component of the Carbon export through the Aquatic Conduit in a Boreal Landscape. Glob. Change Biol. 19 (3), 785–797. doi:10.1111/gcb.12083
Wang, C., Xie, Y., Liu, S., McCallum, J. L., Li, Q., and Wu, J. (2021). Effects of Diffuse Groundwater Discharge, Internal Metabolism and Carbonate Buffering on Headwater Stream CO2 Evasion. Sci. Total Environ. 777, 146230. doi:10.1016/j.scitotenv.2021.146230
Wang, X., He, Y., Yuan, X., Chen, H., Peng, C., Zhu, Q., et al. (2017). pCO2 and CO2 fluxes of the Metropolitan River Network in Relation to the Urbanization of Chongqing, China. J. Geophys. Res. Biogeosci. 122 (3), 470–486. doi:10.1002/2016JG003494
Wickland, K. P., Neff, J. C., and Aiken, G. R. (2007). Dissolved Organic Carbon in Alaskan Boreal Forest: Sources, Chemical Characteristics, and Biodegradability. Ecosystems 10 (8), 1323–1340. doi:10.1007/s10021-007-9101-4
Winterdahl, M., Wallin, M. B., Karlsen, R. H., Laudon, H., Öquist, M., and Lyon, S. W. (2016). Decoupling of Carbon Dioxide and Dissolved Organic Carbon in Boreal Headwater Streams. J. Geophys. Res. Biogeosci. 121 (10), 2630–2651. doi:10.1002/2016JG003420
Keywords: carbon dioxide, carbon dioxide emissions, groundwater, carbon cycle, headwater streams
Citation: Chan CN, Tsang CL, Lee F, Liu B and Ran L (2021) Rapid Loss of Dissolved CO2 From a Subtropical Steep Headwater Stream. Front. Earth Sci. 9:741678. doi: 10.3389/feart.2021.741678
Received: 15 July 2021; Accepted: 11 October 2021;
Published: 01 November 2021.
Edited by:
Shafi Mohammad Tareq, Jahangirnagar University, BangladeshReviewed by:
Youhei Yamashita, Hokkaido University, JapanSiyue Li, Southern Cross University, Australia
Copyright © 2021 Chan, Tsang, Lee, Liu and Ran. This is an open-access article distributed under the terms of the Creative Commons Attribution License (CC BY). The use, distribution or reproduction in other forums is permitted, provided the original author(s) and the copyright owner(s) are credited and that the original publication in this journal is cited, in accordance with accepted academic practice. No use, distribution or reproduction is permitted which does not comply with these terms.
*Correspondence: Lishan Ran, lsran@hku.hk