- 1Institute of Marine Sciences, Guangdong Provincial Key Laboratory of Marine Biotechnology, Shantou University, Shantou, China
- 2School of Geography and the Environment, University of Oxford, Oxford, United Kingdom
- 3Southern Marine Science and Engineering Guangdong Laboratory (Zhuhai), Zhuhai, China
The Earth has gone through multiple ice ages in the past million years. Understanding the ice age dynamics is crucial to paleoclimatic study, and is helpful for addressing future climate challenges. Though ice ages are paced by variations in Earth’s orbit geometry, how various climatic system components on the Earth respond to insolation forcing and interact with each other remains unclear. A prevailing view argues that the initial responses occur in the northern high latitudes (i.e. the northern high-latitude hypothesis, NHH). This opinion is challenged by recent reports, such as the lead of climate change in the Southern Hemisphere (SH) relative to that in the Northern Hemisphere (NH), the southern control on Atlantic meridional overturning circulations (AMOC), and the potential significance of Southern Hemisphere (SH). Alternatively, the tropical hypothesis (TH) argues for a leading role of the tropics. Both the NHH and the TH belong to a single-forcing mechanism, and have difficulty in interpreting phenomena, such as the saw-tooth pattern of the ice ages. Here we present a new proposal concerning the Earth’s ice age dynamics: the bimodal forcing hypothesis (BFH). The essential assumption of this hypothesis is that for glacial-interglacial cycles, the cooling (glaciation) starts from the northern high latitudes, whereas the warming (deglaciations) starts from the SH. Particularly, the BFH emphasizes the significance of SH oceans in accumulating and transferring heat for deglaciations. Thus, it is capable to reasonably explain the saw-tooth pattern. We compiled 100 paleotemperature records globally for validation. The BFH is consistent with most of these records, and provides a straightforward and comprehensible way to interpret ice age on Earth.
Introduction
How the Earth’s climate would respond to increasing anthropogenic atmospheric carbon dioxide concentration concerns the human society (IPCC and Stocker, 2013; Steffen et al., 2018). Resolving this problem requires insights from both the current and paleoclimatic studies (e.g. Zheng et al., 2021). Climate of the past ∼2.6 million years (Myr) is characterized by repeated growth and decay of large continental ice sheets, with astronomical cycles at ∼23 thousand years (kyr), ∼41 kyr, and ∼100 kyr (Milankovitch, 1941; Hays et al., 1976). Although variations in Earth orbital geometry have been widely accepted as the ultimate cause of glacial-interglacial cycles, the process that translates the insolation oscillations into periodic climate change is still in debate (Imbrie et al., 1992, 1993; Denton et al., 2010). A long-lasting enigma is the saw-tooth pattern of ice ages, i.e., relatively long periods of ice-sheet growth followed by relatively short periods of ice-sheet decay (Broecker and Donk, 1970; Lisiecki and Raymo, 2005). How do symmetric insolation fluctuations result in asymmetric ice age cycles? Internal feedbacks in Earth’s climate system are believed to be the answer. Different understandings of internal feedbacks with emphasis on different climate components lead to multiple climate hypotheses, such as the northern high-latitude hypothesis (NHH; e.g. Denton et al., 2010) and the tropical hypothesis (TH; e.g. Cane, 1998; Clement et al., 2001; Chiang, 2009; Beck et al., 2018; Wang, 2021). The NHH and the TH, both belong to a single-forcing mechanism (Ding, 2006), argue for a leading role of the northern high latitudes and the tropics, respectively. However, a single-forcing mechanism has difficulty in interpreting phenomena like the saw-tooth pattern.
The bimodal forcing hypothesis (BFH) we proposed here assumes cooling/glaciation starts from the northern high-latitudes while warming/deglaciation from the SH. We tested this hypothesis with a compilation of 100 global paleotemperature records. The BFH is compatible with most of them, and can better explain puzzles like the saw-tooth problem. In the following, we first outline the NHH and its drawbacks, then illustrate the logic of the BFH, and finally discuss future directions of this new hypothesis.
The Northern High-Latitude Hypothesis and Its Drawbacks
The Key Points of Northern High-Latitude Hypothesis
So far, the NHH has been the most prevailing ice age hypothesis. Essentially, it argues for a uniquely triggering role of the summer insolation at 65°N in both hemispheres (e.g. Kawamura et al., 2007). A concomitant assumption is that initial responses to insolation forcing happen in northern high latitudes, no matter in ice age onsets (e.g. Imbrie et al., 1992; 1993) or terminations (e.g. Denton et al., 2010). Besides, the ‘switch’ of Atlantic meridional overturning circulation (AMOC), which exerts an important effect on interhemispheric heat redistribution, is assumed to lie in the northern North Atlantic (e.g. Broecker and Denton, 1989; Clark et al., 2002). After the pioneering interpretation of glacial-interglacial cycles from a northern perspective (Imbrie et al., 1992; 1993), a comprehensive NHH-based picture of working processes during the last deglaciation was presented (Denton et al., 2010).
In summary, the rising NH summer insolation at the end of Last Glacial Maximum (LGM) (Figure 1A, black curve) initiated ice-sheet melting and delivered freshwater into the North Atlantic. This freshwater forcing shut down the AMOC during the Heinrich stadial 1 (HS1) interval (Figure 1B; Ganopolski and Rahmstorf, 2001; McManus et al., 2004; Liu et al., 2009), resulting in the deglacial warming in the SH through the bipolar seesaw mechanism (Crowley, 1992; Broecker, 1998; Stocker and Johnsen, 2003). Meanwhile, reduced AMOC and expanded sea ice in North Atlantic caused the southward shift of SH westerlies and the release of CO2 from the deep ocean to the atmosphere (Figure 1C; Toggweiler et al., 2006; Anderson et al., 2009). The last deglaciation was accomplished when atmospheric CO2 was raised above threshold.
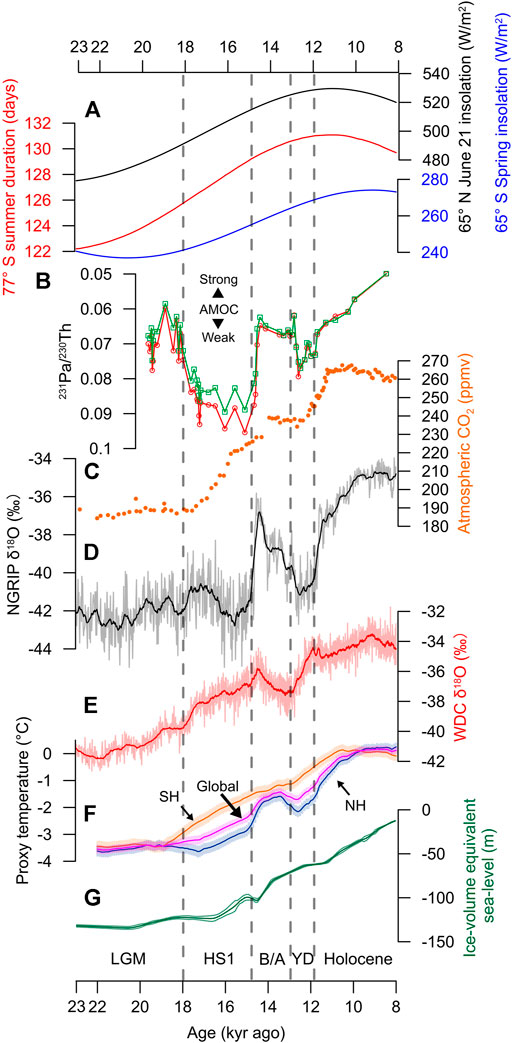
FIGURE 1. Climatic records during the last deglaciation. (A) 65°N June 21 insolation (black) (Laskar et al., 2004). 65°S averaged mean longitude spring insolation (August 21 to November 20, blue) (Laskar et al., 2004; Stott et al., 2007). 77°S summer duration (red), which is measured as the number of days whose diurnal average insolation exceeds 250 W/m2 (Huybers and Denton, 2008). (B) North Atlantic core OCE326-GGC5 231Pa/230Th ratios for the overall strength of AMOC. Red and green are 238U-based value and 232U-based results, respectively. Increasing values (plotted downward) indicate reduced overturning strength (McManus et al., 2004). (C) Atmospheric CO2 from EPICA Dome C (EDC) ice core (Monnin et al., 2001). (D) δ18O from North Greenland Ice Core Project (NGRIP) ice core (grey. Black for 20 points smoothed data). (Rasmussen et al., 2006). (E) δ18O from West Antarctic Ice Sheet Divide (WDC) ice core, (grey. Black for 30 points smoothed data) (WAIS Divide Project Members, 2015). (F) Northern Hemisphere (NH; blue), Southern Hemisphere (SH; orange), and global (pink) surface temperature stacks with 1σ error range (Shakun et al., 2012). (G) Ice-volume equivalent sea level plotted with 2σ uncertainty (Lambeck et al., 2014). All data are on their published timescale. Periods of Last Glacial Maximum (LGM), Henrich stadial 1 (HS1), Bølling-Allerød (B/A), Younger Dryas (YD) and Holocene are separate by grey dashed lines.
The seemingly self-consistent NHH actually has unsolvable problems, and there is an increasing number of studies challenging the NHH. We summarize three aspects of these challenges, i.e., the lead of climate change in the SH with respect to that in the NH, the southern control on AMOC variations, and the potential significance of SH insolation.
The Lead of Climate Change in the Southern Hemisphere with Respect to That in the Northern Hemisphere
Orbital-scale climate changes in the NH do not always lead those in the SH or the tropics (Shackleton and Pisias, 1985; Sowers et al., 1991, 1993; Pichon et al., 1992; Broecker and Henderson, 1998; Paillard and Parrenin, 2004; Bouttes et al., 2011, 2012; Paillard, 2015; Mariotti et al., 2016). Changes in sea surface temperature (SST) from the SH Indian Ocean (Hays et al., 1976) and the tropical Pacific (Lea et al., 2000; Visser et al., 2003) led variations in NH ice sheets by up to a few thousand years. Particularly, an early deglacial warming prior to the LGM has been observed from the SH mid/low-latitude SST records (Pahnke and Sachs, 2006; Santos et al., 2017).
Polar ice cores offer another opportunity to compare temporal relationship between hemispheres. A pioneering attempt to synchronize ice core records from the Antarctica and the Greenland showed that the onset of warming in Antarctica occurred ∼3 kyr prior to that in Greenland during the last deglaciation (14.7 kyr ago; Figure 1D; Sowers and Bender, 1995). A surface air temperature record from the West Antarctic Ice Sheet Divide ice core (WDC; Figure 1E) revealed that significant warming in West Antarctica began by 20 kyr ago during the last deglaciation, confirming an earlier deglacial warming in Antarctica (WAIS Divide Project Members, 2013). Additionally, Antarctic Vostok temperature rising occurred ∼4–9 kyr prior to the ice-sheet melting during the last four ice age terminations (Petit et al., 1999).
Compilations of surface temperature reconstructions during the last termination further confirm early warming in the SH and the tropics. Assessment of deglacial SST development in the Pacific Ocean revealed that the timing of initial deglacial warming was generally early (before 18 kyr ago) in the south, late (later than 15.5 kyr ago) in the north, and mixed with both early and intermediate (between 18 and 15.5 kyr ago) signals in the tropics (Kiefer and Kienast, 2005; Tachikawa et al., 2009). A lead of deglacial warming in the SH relative to that in the NH was confirmed at a global scale as well (Figure 1F; Shakun et al., 2012).
We also compile 100 paleotemperature records during the last deglaciation (see Material and Methods), including land/sea surface and deep ocean temperatures, and compare their initial timings of the last deglacial warming (Figure 2). Two points stand out from this temporal-spatial warming pattern of the last deglaciation. First, initial warming in the SH (mostly centered between 20 and 18 kyr ago) is earlier than in the NH (mostly centered after 18 kyr ago). Second, warming started prior to 20 kyr ago in some SH surface records, and prior to 18 kyr ago in two deep ocean records. This early and strong warming is inconsistent with the NHH view that bipolar seesaw initiates deglacial warming in the SH.
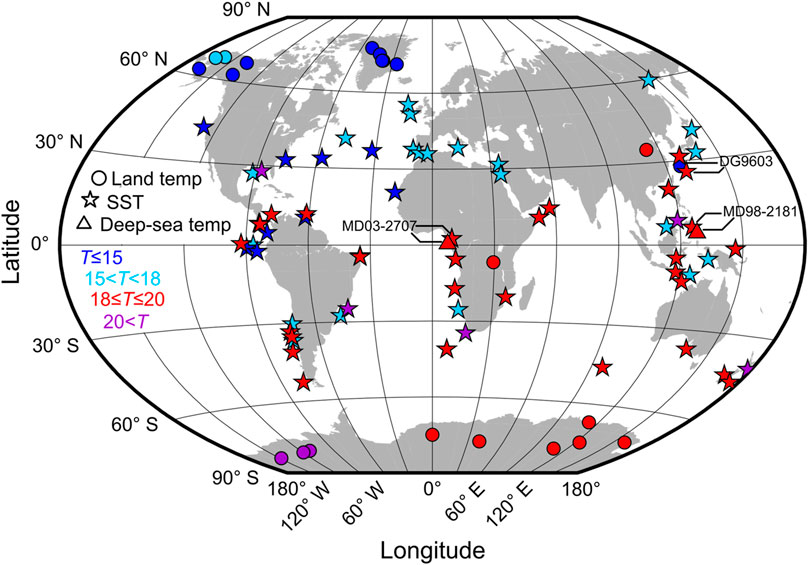
FIGURE 2. Timings of initial warming from 100 climatic records worldwide. Different data shapes represent temperature records of land (circle), sea surface (pentacle), and deep-sea (triangle, only two), respectively. Different timings of initial warming (T) are differentiated by colors. Oceanic temperature records which are too close to or seriously overlapped with the land data points have been shifted slightly from their original positions. Note that core MD03-2707 and MD98-2181 contain both SST and deep-sea temperature records, and core DG9603 in Okinawa trough contains SST and pollen records which reflect adjacent land climate change. Most records in the SH show early initial warming (before 18 kyr), whereas records in the middle and high NH show either moderate (between 18 and 15 kyr) or late (after 15 kyr) initial warming. The early deglacial warming observed in some tropical and NH mid-latitude records (e.g. West Pacific) may partly arise from the influence of low-latitude insolation (Xu et al., 2013; Wang, 2021). Details about the database for this map can be found in supplementary materials.
The Southern Control on Atlantic Meridional Overturning Circulations Variations
An 213Pa/230Th record recovered from the subtropical North Atlantic has demonstrated that a nearly total shutdown of AMOC occurred between 17.5 and 15 kyr ago, followed by an abrupt resumption at ∼14.7 kyr ago (McManus et al., 2004; Böhm et al., 2015). The near-collapse of AMOC during the HS1 interval was regarded as the cause of SH warming in NHH, and was usually attributed to catastrophic iceberg discharge into the North Atlantic (e.g. Ganopolski and Rahmstorf, 2001; Liu et al., 2009). However, recent studies favor that episodic Heinrich events with huge iceberg discharge were the results rather than the causes of AMOC reduction. According to a sedimentary record from the northeast Atlantic, for the majority of abrupt climate changes over the past four glacial cycles, iceberg arrived too late to trigger cooling (Barker et al., 2015). And episodic ice-discharge events were probably a consequence of increased basal melting rate due to increased subsurface temperature caused by an inactive AMOC (Shaffer et al., 2004; Liu et al., 2009). A northwest Atlantic record showed that, for four Heinrich events (H1, H3, H5a and H6), the subsurface water started to warm approximately 1–2 kyr before each Heinrich event (Marcott et al., 2011). New evidence also suggested that the small amount of meltwater from ice-sheet margins during early deglaciation was unable to induce near-complete collapse of AMOC (e.g. Colin de Verdière et al., 2006; Sévellec et al., 2010).
In addition, the coupling of AMOC variations and sea level changes during the last deglaciation was at odds with a northern freshwater-forcing scenario, which predicts association of more freshwater input (i.e. larger sea level rise) with a less active AMOC. Within the duration when the AMOC was significantly reduced (17.5–15 kyr ago), a rise of ∼25 m at a rate of ∼12 m kyr−1 only occurred during the latter half of the interval (∼16.5–15 kyr ago) after a period of near-constant sea level between ∼18 and 16.5 kyr ago (Figure 1G; Lambeck et al., 2014). In contrast, the most prominent meltwater pulse (MWP-1A; ∼20 m in less than 500 years) coincided with a sharp resumption of AMOC at ∼14.6 kyr ago (Deschamps et al., 2012).
To overcome deficiencies in modulating AMOC with a northern origin, some researchers argue for a southern control on the AMOC variability. A numerical simulation revealed that a gradual warming and sea-ice retreat in the Southern Ocean during deglaciations would induce an abrupt resumption of AMOC (Knorr and Lohmann, 2003). Another simulation also showed that freshwater perturbations to the regions of Antarctic Intermediate Water (AAIW) formation would induce a shift of North Atlantic Deep Water (NADW) formation from the “off” to the “on” mode (Weaver et al., 2003). This driving mechanism well explains the synchronicity of MWP-1A with AMOC resumption during the last deglaciation.
The Potential Significance of Southern Hemisphere Insolation
The NHH view that NH summer insolation drives terminations is questioned by recent proposals that local insolation is at least equally important in driving terminations in the SH. For example, due to the non-linear relationship between radiation and temperature, the seasonal distribution of the solar energy could control the annual temperature of the Antarctic (Huybers and Denton, 2008). This radiation-driven temperature (proportional to the local summer duration) was rising from about 25 kyr ago (Huybers and Denton, 2008). Alternatively, increased springtime insolation over the Southern Ocean (Stott et al., 2007) along with retreating sea ice due to the positive feedback between temperature and sea ice area (Levermann et al., 2007), could account for early onset of deglacial warming in the SH. Particularly, the retreat of sea ice would lead to decreased stratification in the Southern Ocean by inducing enhanced Ekman transport, both contributing to the subsequent rise in atmospheric CO2 (Stephens and Keeling, 2000; Stott et al., 2007; Basak et al., 2018). Moreover, there are doubts that Antarctic temperature records derived from ice cores were biased, and the orbital component of the bias-corrected temperature record can be explained by local insolation without involving the NH (Laepple et al., 2011).
In summary, the astronomical-forcing theory nicely explains the dominant periods within glacial-interglacial cycles, but fails to illustrate explicitly the response of different climate system components to insolation forcing.
The Bimodal Forcing Hypothesis
A single-forcing mechanism such as the NHH seems unsatisfactory in explaining ice age dynamics, thus we put forth the BFH. In this conceptual model, glaciations (cooling) start from the NH while deglaciations (warming) begin from the SH (Figure 3). Here we present an interpretation of the onset and termination of the last glacial period (∼120–10 kyr ago) under the framework of BFH, which fits better with available paleoclimatic records and model simulations.
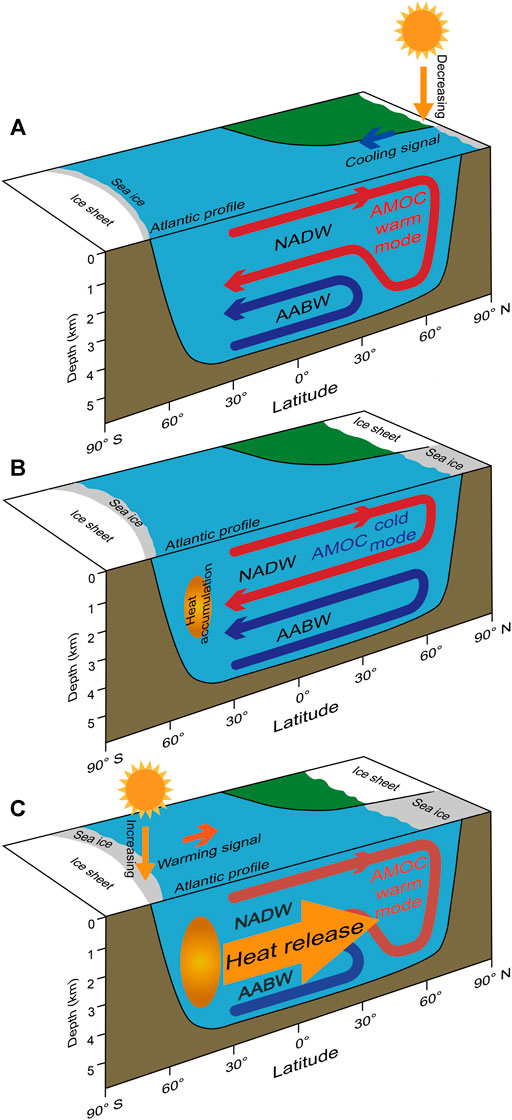
FIGURE 3. Schematic of the bimodal forcing hypothesis. (A) During glacial inception, high northern latitudes first respond to decreasing insolation, and the coeval vigorous AMOC helps to deliver cooling signal to the rest of the Earth. (B) AMOC switches from a warm to cold mode when ice age is near its maximum, and this mode change results in heat accumulation in the SH. (C) The accumulated heat together with SH insolation cause the deglacial warming in the Southern Ocean and Antarctica. This warming and associated sea-ice retreat and CO2 release terminate glacial conditions. Particularly, warming in the SH leads to AMOC resumption during deglaciations, this resumption is accompanied by AMOC overshoot (Skinner et al., 2013) and transported a huge amount of heat from the SH to the NH.
Cooling Down: Mechanism of the Onset of the Last Ice Age
The BFH assumes that northern high latitudes firstly responded to the decline of NH summer insolation beginning at ∼128 kyr ago (Figure 4A), same as the NHH. Diminishing insolation triggered the Earth moving forward into the last ice age, assisted by a series of positive feedbacks such as vegetation and sea ice (Khodri et al., 2005). An early oceanic response to insolation to the south of Greenland indicated that SST started to decrease at ∼119 kyr ago and a strong ice-rafted-deposit (IRD) input occurred at ∼117 kyr ago, signaling the first regrowth of the Greenland Ice Sheet during the end of last interglacial period (Irvalı et al., 2016).
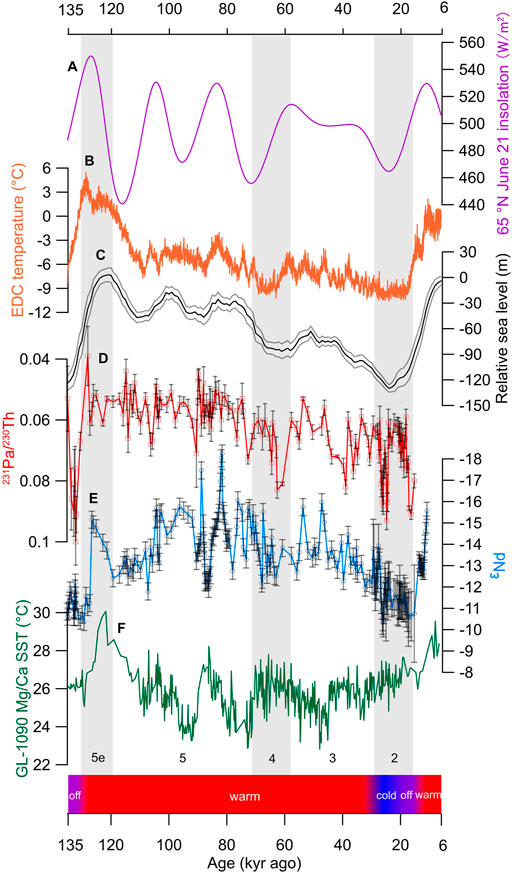
FIGURE 4. Climatic records since the last interglacial period. (A) 65°N June 21 insolation (Laskar et al., 2004). (B) EDC temperature reconstruction based on ice δD (Jouzel et al., 2007). (C). Composite sea level in one σ error (Spratt and Lisiecki, 2016). North Atlantic core ODP 1063 231Pa/230Th (D) and 143Nd/144Nd (εNd) (E; Böhm et al., 2015) records. εNd is a proxy for changes in sources and mixing of water masses. Increasced values (plotted downward) indicate shoaling of the AMOC. (F) Western South Atlantic core GL-1090 SST data (Santos et al., 2017). All data are on their published timescale. The mode shifts of AMOC derived from the combined 231Pa/230Th and εNd records is showed in the bottom (Böhm et al., 2015). Grey and orange shadings mark cold periods (MIS 2, 4) and the last interglacial (MIS 5e), respectively.
This initial cooling in the northern high latitudes was propagated through oceanic and atmospheric circulations towards the rest of the world. According to studies on oceanic circulations (e.g. Broecker, 1991; Marshall and Speer, 2012; Talley, 2013; Ferrari et al., 2014), once a cooling signal was imprinted on the northern North Atlantic, the formation regions of NADW, during the onset of last ice age, temperature of the whole ocean would begin to decrease within centuries. Reconstructions of deep ocean temperatures at various sites and with different methods all reveal a significant cooling during the MIS 5e-5 days transition (∼115 kyr ago; e.g. Labeyrie et al., 1987; Shackleton, 2000; Cutler et al., 2003; Adkins, 2013). For instance, a deep ocean cooling of ∼2°C was estimated between the MIS 5e and MIS 5c for two drilling cores in the Atlantic and Pacific (Cutler et al., 2003). Also, considerable temperature reductions around the MIS 5e-5 days transition were observed worldwide at sea (e.g. Labeyrie et al., 1996; Lea et al., 2000; Tachikawa et al., 2009; Koutavas, 2018), and land surface (e.g. Jouzel et al., 2007; Figure 4B). Compared to oceanic processes, atmospheric circulations played a relatively subordinate role during the onset of last ice age, because the contribution of atmosphere to rapid deep ocean cooling is limited, and the tropics is a barrier for trans-hemispheric atmospheric processes (Manabe and Broccoli, 1985; Chiang and Bitz, 2005). The aforementioned cooling trends are apparently synchronous at a global scale, which seems in contradiction with the BFH. However, this synchronicity could be misled by dating uncertainties and low sampling resolution.
Warming up: Mechanism of the Termination of the Last Ice Age
From its beginning at ∼120 kyr ago, the last ice age lasted until the deglaciation at ∼18 kyr ago (Figure 4C). Within the glacial period, the Earth experienced three NH summer insolation peaks but none triggered deglaciations (Figure 4A; Cheng et al., 2016), implying that apart from insolation, internal feedbacks are also needed to determine terminations. Simultaneous measurements of 231Pa/230Th and 143Nd/144Nd from North Atlantic evidenced a warm-to-cold mode shift of AMOC during the MIS three to two transition at ∼27 kyr ago (Figures 4D,E; Böhm et al., 2015). As reduction in AMOC would lead to heat accumulation in SH through the bipolar seesaw, the mode shift of AMOC during the later part of last ice age was probably responsible for the early deglaciation warming registered in SH low/mid-latitudes (e.g. Pahnke and Sachs, 2006; Santos et al., 2017; Figure 4F). The rapid ice-sheet melting indicated by asymmetric saw-tooth pattern required huge amount of heat, which might partly source from the accumulated heat in the SH linked to reduced AMOC.
The deglacial warming in the Southern Ocean and Antarctica at ∼20 kyr ago lagged that in the SH mid/low latitudes occurring at ∼27 kyr ago, indicating a southward transmission of heat in the SH. The summer sea-ice edge around the Antarctica started to retreat at ∼23 kyr ago (Collins et al., 2012), the surface air in the West Antarctica had felt the warming by ∼20 kyr ago (WAIS Divide Project Members, 2013), and the Southern Ocean began to warm at ∼19 kyr ago (Stott et al., 2007). This lag of deglacial warming possibly result from the existence of a thermal threshold between the Southern Ocean and the SH low/mid-latitudes, which was finally reached when the SH summer duration (Huybers and Denton, 2008; Figure 1A, red curve), and/or spring insolation (Stott et al., 2007; Figure 1A, blue curve), began to increase at ∼23 kyr ago. The accumulated heat associated with reduced AMOC (Santos et al., 2017), or the heat accumulation in deep Southern Ocean during the long Greenland stadial starting from 27.6 kyr ago (Skinner et al., 2020) could contribute to this initial warming. Once Antarctic sea ice had retreated and the Southern Ocean had warmed, positive feedbacks worked to release CO2 from the deep ocean to the atmosphere (e.g. Stephens and Keeling, 2000; Toggweiler et al., 2006; Watson et al., 2015), and CO2 helped to drive the warming in the NH (Shakun et al., 2012). Moreover, heat carried by a Southern Ocean-origin water mass led to a 3°C warming in the deep North Atlantic during the HS1, possibly resulting in the reinvigoration of AMOC at ∼14.7 kyr ago (Thiagarajan et al., 2014). Simulations also support a controlling role of the Southern Ocean warming in AMOC resumption (Knorr and Lohmann, 2003; Weaver et al., 2003; Buizert and Schmittner, 2015). The resumpted AMOC transported a considerable amount of heat from the SH to the NH at an unprecedented rate in the next few hundred years and caused a rapid melting of ice sheets, the MWP-1A (Deschamps et al., 2012). By this time, the transition from the LGM to Holocene on both hemispheres seemed irreversible.
In summary, the BFH is distinct from previous single-forcing hypotheses, in that it assumes the initial response of glacial onsets is different from that of glacial terminations. When the NH summer insolation starts to decline, it is the northern high latitudes that first react. The NH ice sheets begin to grow, and the high-latitude surface temperature begin to decrease. Subsequently, through a series of atmospheric and oceanic processes, especially the global oceanic overturning circulation, the cooling signal is transmitted towards the rest of the world. As the ice age proceeds near its maximum, the AMOC switches from a warm to a cold mode due to a few possible reasons, such as a threshold value has been reached by the volume of NH ice sheets, the temperature of NADW (Adkins, 2013), or the area of Antarctic sea ice (Ferrari et al., 2014). Then heat begins to accumulate in the SH as a result of reduced AMOC. Once the accumulated heat plus the SH local insolation attains a certain value, the Antarctic surface temperature starts to increase, the sea ice around the Antarctica starts to retreat, and the CO2 preserved in deep ocean is added back to the atmosphere. Finally, the heat stored in the SH becomes so large that the AMOC switches back to the warm mode and both hemispheres move toward another interglacial period.
FUTURE DIRECTIONS
In general, the BFH differs from previous ice-age hypotheses in two aspects. First, it assumes that different climatic components drive glaciation and deglaciation, contrasting with single-forcing hypotheses which argue for a dominant role of a single climatic component during both ice-age onset and termination. This might benefit the understanding for the saw-tooth pattern, which indicates different processes of cooling and warming. Second, the BFH recognizes the significance of SH oceans in accumulating and transferring heat for terminations.
The BFH awaits further improvement, as many issues need to be resolved. For example, a crucial idea within the BFH, that reduced AMOC at the MIS three to two transition contributed to heat accumulation in the SH, is not yet consistent with traditional bipolar seesaw which is used to explain millennial-scale oscillations. Specific mechanisms transmitting heat from the SH low/mid latitudes to the Southern Ocean and Antarctica during ∼27-∼20 kyr ago remains unclear. And to which extent SH local insolation contributes to deglaciation is unsettled.
Materials and Methods
In order to investigate the temporal-spatial distribution of the last glacial-interglacial transition (the time when temperature or proxy records have obviously shifted), we compiled 100 proxy records around the world (Supplementary Table S1, Supplementary Figure S1). Only proxy records with firm chronological control and high resolution have been collected. For records with the 14C-based age model, we recalibrated all available 14C dates using Intcal 13 and SHcal 13. Then the age model was built up by linear interpolation between 14C-dated control points. We determine the start of deglaciation by visually identifying the first obvious shift in temperature or proxy curves since 34 kyr ago. Then this age is compared to the timing given by the original author(s) if available. In case that the result has apparent discrepancies (the difference between two ages is over 1,000 yr), we adopt the opinion of original author(s).
Data Availability Statement
The original contributions presented in the study are included in the article/Supplementary Material, further inquiries can be directed to the corresponding author.
Author Contributions
ZL proposed the hypothesis. ZL, YX, and PZ wrote the paper. PZ made figures. ZL, YX, and PZ have equal contributions.
Funding
This work was funded by National Natural Science Foundation of China (Grant No. 41877438, 41290252), and the STU Scientific Research Foundation for Talents (Grant No. NTF19003), both granted to ZL.
Conflict of Interest
The authors declare that the research was conducted in the absence of any commercial or financial relationships that could be construed as a potential conflict of interest.
Publisher’s Note
All claims expressed in this article are solely those of the authors and do not necessarily represent those of their affiliated organizations, or those of the publisher, the editors and the reviewers. Any product that may be evaluated in this article, or claim that may be made by its manufacturer, is not guaranteed or endorsed by the publisher.
Acknowledgments
Peter Clark and Joerg Lippold shared their published data. ZL thanks late Prof. Nicholas Shackleton for inspiring discussions many years ago when ZL was a DPhil student in Oxford, which triggered the thinking of this issue.
Supplementary Material
The Supplementary Material for this article can be found online at: https://www.frontiersin.org/articles/10.3389/feart.2021.736895/full#supplementary-material
References
Adkins, J. F. (2013). The Role of Deep Ocean Circulation in Setting Glacial Climates. Paleoceanography 28 (3), 539–561. doi:10.1002/palo.20046
Anderson, R. F., Ali, S., Bradtmiller, L. I., Nielsen, S. H. H., Fleisher, M. Q., Anderson, B. E., et al. (2009). Wind-Driven Upwelling in the Southern Ocean and the Deglacial Rise in Atmospheric CO2. Science 323 (5920), 1443–1448. doi:10.1126/science.1167441
Barker, S., Chen, J., Gong, X., Jonkers, L., Knorr, G., and Thornalley, D. (2015). Icebergs Not the Trigger for North Atlantic Cold Events. Nature 520 (7547), 333–336. doi:10.1038/nature14330
Basak, C., Fröllje, H., Lamy, F., Gersonde, R., Benz, V., Anderson, R. F., et al. (2018). Breakup of Last Glacial Deep Stratification in the South Pacific. Science 359 (6378), 900–904. doi:10.1126/science.aao2473
Beck, J. W., Zhou, W., Li, C., Wu, Z., White, L., Xian, F., et al. (2018). A 550,000-year Record of East Asian Monsoon Rainfall from10Be in Loess. Science 360 (6391), 877–881. doi:10.1126/science.aam5825
Böhm, E., Lippold, J., Gutjahr, M., Frank, M., Blaser, P., Antz, B., et al. (2015). Strong and Deep Atlantic Meridional Overturning Circulation during the Last Glacial Cycle. Nature 517 (7532), 73–76. doi:10.1038/nature14059
Bouttes, N., Paillard, D., Roche, D. M., Brovkin, V., and Bopp, L. (2011). Last Glacial Maximum CO2 and δ13C Successfully Reconciled. Geophys. Res. Lett. 38, L02705. doi:10.1029/2010gl044499
Bouttes, N., Paillard, D., Roche, D. M., Waelbroeck, C., Kageyama, M., Lourantou, A., et al. (2012). Impact of Oceanic Processes on the Carbon Cycle during the Last Termination. Clim. Past 8, 149–170. doi:10.5194/cp-8-149-2012
Broecker, W. S., and Denton, G. H. (1989). The Role of Ocean-Atmosphere Reorganizations in Glacial Cycles. Geochimica et Cosmochimica Acta 53 (10), 2465–2501. doi:10.1016/0016-7037(89)90123-3
Broecker, W. S., and Henderson, G. M. (1998). The Sequence of Events Surrounding Termination II and Their Implications for the Cause of Glacial-Interglacial CO2 changes. Paleoceanography 13 (4), 352–364. doi:10.1029/98pa00920
Broecker, W. S. (1998). Paleocean Circulation during the Last Deglaciation: a Bipolar Seesaw? Paleoceanography 13 (2), 119–121. doi:10.1029/97pa03707
Broecker, W. S., and van Donk, J. (1970). Insolation Changes, Ice Volumes, and the O18 record in Deep-Sea Cores. Rev. Geophys. 8 (1), 169–198. doi:10.1029/rg008i001p00169
Buizert, C., and Schmittner, A. (2015). Southern Ocean Control of Glacial AMOC Stability and Dansgaard-Oeschger Interstadial Duration. Paleoceanography 30, 1595–1612. doi:10.1002/2015pa002795
Cane, M. A. (1998). CLIMATE CHANGE: A Role for the Tropical Pacific. Science 282, 59–61. doi:10.1126/science.282.5386.59
Cheng, H., Edwards, R. L., Sinha, A., Spötl, C., Yi, L., Chen, S., et al. (2016). The Asian Monsoon over the Past 640,000 Years and Ice Age Terminations. Nature 534 (7609), 640–646. doi:10.1038/nature18591
Chiang, J. C. H., and Bitz, C. M. (2005). Influence of High Latitude Ice Cover on the marine Intertropical Convergence Zone. Clim. Dyn. 25 (5), 477–496. doi:10.1007/s00382-005-0040-5
Chiang, J. C. H. (2009). The Tropics in Paleoclimate. Annu. Rev. Earth Planet. Sci. 37, 263–297. doi:10.1146/annurev.earth.031208.100217
Clark, P. U., Pisias, N. G., Stocker, T. F., and Weaver, A. J. (2002). The Role of the Thermohaline Circulation in Abrupt Climate Change. Nature 415, 863–869. doi:10.1038/415863a
Clement, A. C., Cane, M. A., and Seager, R. (2001). An Orbitally Driven Tropical Source for Abrupt Climate Change. J. Clim. 14 (11), 2369–2375. doi:10.1175/1520-0442(2001)014<2369:aodtsf>2.0.co;2
Colin de Verdière, A., Ben Jelloul, M., and Sévellec, F. (2006). Bifurcation Structure of Thermohaline Millennial Oscillations. J. Clim. 19, 5777–5795. doi:10.1175/jcli3950.1
Collins, L. G., Pike, J., Allen, C. S., and Hodgson, D. A. (2012). High-resolution Reconstruction of Southwest Atlantic Sea-Ice and its Role in the Carbon Cycle during marine Isotope Stages 3 and 2. Paleoceanography 27, PA3217. doi:10.1029/2011pa002264
Crowley, T. J. (1992). North Atlantic Deep Water Cools the Southern Hemisphere. Paleoceanography 7 (4), 489–497. doi:10.1029/92pa01058
Cutler, S., Cutler, H., Matesic, D., Cheng, H., Adkins, J., Gallup, C. D., et al. (2003). Mode of Action of Phytotoxic Fungal Metabolites. Earth Planet. Sci. Lett. 206 (3–4), 253–270. doi:10.1201/9780203492789.ch13
Denton, G. H., Anderson, R. F., Toggweiler, J. R., Edwards, R. L., Schaefer, J. M., and Putnam, A. E. (2010). The Last Glacial Termination. Science 328, 1652–1656. doi:10.1126/science.1184119
Deschamps, P., Durand, N., Bard, E., Hamelin, B., Camoin, G., Thomas, A. L., et al. (2012). Ice-sheet Collapse and Sea-Level Rise at the Bølling Warming 14,600 Years Ago. Nature 483 (7391), 559–564. doi:10.1038/nature10902
Ding, Z. (2006). The Milankovitch Theory of Pleistocene Glacial Cycles: Challenges and Chances. Quat. Sci. 26 (5), 710–717. (In Chinese with English abstract). doi:10.3321/j.issn:1001-7410.2006.05.005
Ferrari, R., Jansen, M. F., Adkins, J. F., Burke, A., Stewart, A. L., and Thompson, A. F. (2014). Antarctic Sea Ice Control on Ocean Circulation in Present and Glacial Climates. Proc. Natl. Acad. Sci. 111 (24), 8753–8758. doi:10.1073/pnas.1323922111
Ganopolski, A., and Rahmstorf, S. (2001). Rapid Changes of Glacial Climate Simulated in a Coupled Climate Model. Nature 409, 153–158. doi:10.1038/35051500
Hays, J. D., Imbrie, J., and Shackleton, N. J. (1976). Variations in the Earth's Orbit: Pacemaker of the Ice Ages. Science 194, 1121–1132. doi:10.1126/science.194.4270.1121
Huybers, P., and Denton, G. (2008). Antarctic Temperature at Orbital Timescales Controlled by Local Summer Duration. Nat. Geosci 1, 787–792. doi:10.1038/ngeo311
Imbrie, J., Berger, A., Boyle, E. A., Clemens, S. C., Duffy, A., Howard, W. R., et al. (1993). On the Structure and Origin of Major Glaciation Cycles 2. The 100,000-year Cycle. Paleoceanography 8, 699–735. doi:10.1029/93pa02751
Imbrie, J., Boyle, E. A., Clemens, S. C., Duffy, A., Howard, W. R., Kukla, G., et al. (1992). On the Structure and Origin of Major Glaciation Cycles 1. Linear Responses to Milankovitch Forcing. Paleoceanography 7, 701–738. doi:10.1029/92pa02253
Irvalı, N., Ninnemann, U. S., Kleiven, H. F., Galaasen, E. V., Morley, A., and Rosenthal, Y. (2016). Evidence for Regional Cooling, Frontal Advances, and East Greenland Ice Sheet Changes during the Demise of the Last Interglacial. Quat. Sci. Rev. 150, 184–199. doi:10.1016/j.quascirev.2016.08.029
IPCC (2013). Climate Change 2013: The Physical Science Basis. Contribution of Working Group I to the Fifth Assessment Report of the Intergovernmental Panel on Climate Change. Editors T. F. Stocker (Cambridge: Cambridge Univ. Press), 11535. doi:10.1017/CBO9781107415324
Jouzel, J., Masson-Delmotte, V., Cattani, O., Dreyfus, G., Falourd, S., Hoffmann, G., et al. (2007). Orbital and Millennial Antarctic Climate Variability over the Past 800,000 Years. Science 317 (5839), 793–796. doi:10.1126/science.1141038
Kawamura, K., Parrenin, F., Lisiecki, L., Uemura, R., Vimeux, F., Severinghaus, J. P., et al. (2007). Northern Hemisphere Forcing of Climatic Cycles in Antarctica over the Past 360,000 Years. Nature 448 (7156), 912–916. doi:10.1038/nature06015
Khodri, M., Cane, M. A., Kukla, G., Gavin, J., and Braconnot, P. (2005). The Impact of Precession Changes on the Arctic Climate during the Last Interglacial-Glacial Transition. Earth Planet. Sci. Lett. 236 (1), 285–304. doi:10.1016/j.epsl.2005.05.011
Kiefer, T., and Kienast, M. (2005). Patterns of Deglacial Warming in the Pacific Ocean: a Review with Emphasis on the Time Interval of Heinrich Event 1. Quat. Sci. Rev. 24, 1063–1081. doi:10.1016/j.quascirev.2004.02.021
Knorr, G., and Lohmann, G. (2003). Southern Ocean Origin for the Resumption of Atlantic Thermohaline Circulation during Deglaciation. Nature 424, 532–536. doi:10.1038/nature01855
Koutavas, A. (2018). Temperature Correlations between the Eastern Equatorial Pacific and Antarctica over the Past 230,000 Years. Earth Planet. Sci. Lett. 485, 43–54. doi:10.1016/j.epsl.2017.12.041
Labeyrie, L. D., Duplessy, J. C., and Blanc, P. L. (1987). Variations in Mode of Formation and Temperature of Oceanic Deep Waters over the Past 125,000 Years. Nature 327 (6122), 477–482. doi:10.1038/327477a0
Labeyrie, L., Labracherie, M., Gorfti, N., Pichon, J. J., Vautravers, M., Arnold, M., et al. (1996). Hydrographic Changes of the Southern Ocean (Southeast Indian Sector) over the Last 230 Kyr. Paleoceanography 11 (1), 57–76. doi:10.1029/95pa02255
Laepple, T., Werner, M., and Lohmann, G. (2011). Synchronicity of Antarctic Temperatures and Local Solar Insolation on Orbital Timescales. Nature 471 (7336), 91–94. doi:10.1038/nature09825
Lambeck, K., Rouby, H., Purcell, A., Sun, Y., and Sambridge, M. (2014). Sea Level and Global Ice Volumes from the Last Glacial Maximum to the Holocene. Proc. Natl. Acad. Sci. 111, 15296–15303. doi:10.1073/pnas.1411762111
Laskar, J., Robutel, P., Joutel, F., Gastineau, M., Correia, A. C. M., and Levrard, B. (2004). A Long-Term Numerical Solution for the Insolation Quantities of the Earth. Astron. Astrophys. 428, 261–285. doi:10.1051/0004-6361:20041335
Lea, D. W., Pak, D. K., and Spero, H. J. (2000). Climate Impact of Late Quaternary Equatorial Pacific Sea Surface Temperature Variations. Science 289, 1719–1724. doi:10.1126/science.289.5485.1719
Levermann, A., Schewe, J., and Montoya, M. (2007). Lack of Bipolar See-Saw in Response to Southern Ocean Wind Reduction. Geophys. Res. Lett. 34, L12711. doi:10.1029/2007gl030255
Lisiecki, L. E., and Raymo, M. E. (2005). A Pliocene-Pleistocene Stack of 57 Globally Distributed Benthic δ18O Records. Paleoceanography 20, PA1003. doi:10.1029/2004pa001071
Liu, Z., Otto-Bliesner, B. L., He, F., Brady, E. C., Tomas, R., Clark, P. U., et al. (2009). Transient Simulation of Last Deglaciation with a New Mechanism for Bolling-Allerod Warming. Science 325 (5938), 310–314. doi:10.1126/science.1171041
Manabe, S., and Broccoli, A. J. (1985). The Influence of continental Ice Sheets on the Climate of an Ice Age. J. Geophys. Res. 90, 2167–2190. doi:10.1029/jd090id01p02167
Marcott, S. A., Clark, P. U., Padman, L., Klinkhammer, G. P., Springer, S. R., Liu, Z., et al. (2011). Ice-shelf Collapse from Subsurface Warming as a Trigger for Heinrich Events. Proc. Natl. Acad. Sci. 108, 13415–13419. doi:10.1073/pnas.1104772108
Mariotti, V., Paillard, D., Bopp, L., Roche, D. M., and Bouttes, N. (2016). A Coupled Model for Carbon and Radiocarbon Evolution during the Last Deglaciation. Geophys. Res. Lett. 43, 1306–1313. doi:10.1002/2015gl067489
Marshall, J., and Speer, K. (2012). Closure of the Meridional Overturning Circulation through Southern Ocean Upwelling. Nat. Geosci 5 (5), 171–180. doi:10.1038/ngeo1391
McManus, J. F., Francois, R., Gherardi, J.-M., Keigwin, L. D., and Brown-Leger, S. (2004). Collapse and Rapid Resumption of Atlantic Meridional Circulation Linked to Deglacial Climate Changes. Nature 428, 834–837. doi:10.1038/nature02494
Milankovitch, M. (1941). Kanon der Erdbestrahlung und seine Andwendung auf das Eiszeitenproblem. Belgrade: Royal Serbian Academy Special Publication. (English translation published in 1969 by Israel Program for Scientific Translations, US Dept. Comm.).
Monnin, E., Indermühle, A., Dällenbach, A., Flückiger, J., Stauffer, B., Stocker, T. F., et al. (2001). Atmospheric CO2 Concentrations over the Last Glacial Termination. Science 291, 112–114. doi:10.1126/science.291.5501.112
Pahnke, K., and Sachs, J. P. (2006). Sea Surface Temperatures of Southern Midlatitudes 0-160 Kyr B. P. Paleoceanography 21, PA2003. doi:10.1029/2005pa001191
Paillard, D., and Parrenin, F. (2004). The Antarctic Ice Sheet and the Triggering of Deglaciations. Earth Planet. Sci. Lett. 227, 263–271. doi:10.1016/j.epsl.2004.08.023
Paillard, D. (2015). Quaternary Glaciations: from Observations to Theories. Quat. Sci. Rev. 107, 11–24. doi:10.1016/j.quascirev.2014.10.002
Petit, J. R., Jouzel, J., Raynaud, D., Barkov, N. I., Barnola, J.-M., Basile, I., et al. (1999). Climate and Atmospheric History of the Past 420,000 Years from the Vostok Ice Core, Antarctica. Nature 399, 429–436. doi:10.1038/20859
Pichon, J.-J., Labeyrie, L. D., Bareille, G., Labracherie, M., Duprat, J., and Jouzel, J. (1992). Surface Water Temperature Changes in the High Latitudes of the Southern Hemisphere over the Last Glacial-Interglacial Cycle. Paleoceanography 7 (3), 289–318. doi:10.1029/92pa00709
Rasmussen, S. O., Andersen, K. K., Svensson, A. M., Steffensen, J. P., Vinther, B. M., Clausen, H. B., et al. (2006). A New Greenland Ice Core Chronology for the Last Glacial Termination. J. Geophys. Res.-Atmos. 111, 907–923. doi:10.1029/2005jd006079
Santos, T. P., Lessa, D. O., Venancio, I. M., Chiessi, C. M., Mulitza, S., Kuhnert, H., et al. (2017). Prolonged Warming of the Brazil Current Precedes Deglaciations. Earth Planet. Sci. Lett. 463, 1–12. doi:10.1016/j.epsl.2017.01.014
Sévellec, F., Huck, T., and Colin de Verdière, A. (2010). From Centennial to Millennial Oscillation of the Thermohaline Circulation. J. Mar. Res. 68, 597–624. doi:10.1357/002224011795977635
Shackleton, N. J., and Pisias, N. G. (1985). “Atmospheric Carbon Dioxide, Orbital Forcing, and Climate,” in The Carbon Cycle and Atmospheric CO2: Natural Variations Archean to Present. Editors E. Sundquist, and W. Broecker (Washington DC: American Geophysical Union).
Shackleton, N. J. (2000). The 100,000-year Ice-Age Cycle Identified and Found to Lag Temperature, Carbon Dioxide, and Orbital Eccentricity. Science 289 (5486), 1897–1902. doi:10.1126/science.289.5486.1897
Shaffer, G., Olsen, S. M., and Bjerrum, C. J. (2004). Ocean Subsurface Warming as a Mechanism for Coupling Dansgaard-Oeschger Climate Cycles and Ice-Rafting Events. Geophys. Res. Lett. 31, L24202. doi:10.1029/2004gl020968
Shakun, J. D., Clark, P. U., He, F., Marcott, S. A., Mix, A. C., Liu, Z., et al. (2012). Global Warming Preceded by Increasing Carbon Dioxide Concentrations during the Last Deglaciation. Nature 484, 49–54. doi:10.1038/nature10915
Skinner, L. C., Scrivner, A. E., Vance, D., Barker, S., Fallon, S., and Waelbroeck, C. (2013). North Atlantic versus Southern Ocean Contributions to a Deglacial Surge in Deep Ocean Ventilation. Geology 41, 667–670. doi:10.1130/g34133.1
Skinner, L., Menviel, L., Broadfield, L., Gottschalk, J., and Greaves, M. (2020). Southern Ocean Convection Amplified Past Antarctic Warming and Atmospheric CO2 Rise during Heinrich Stadial 4. Commun. Earth Environ. 1, 23. doi:10.1038/s43247-020-00024-3
Sowers, T., and Bender, M. (1995). Climate Records Covering the Last Deglaciation. Science 269, 210–214. doi:10.1126/science.269.5221.210
Sowers, T., Bender, M., Labeyrie, L., Martinson, D., Jouzel, J., Raynaud, D., et al. (1993). A 135,000-year Vostok-Specmap Common Temporal Framework. Paleoceanography 8 (6), 737–766. doi:10.1029/93pa02328
Sowers, T., Bender, M., Raynaud, D., Korotkevich, Y. S., and Orchardo, J. (1991). The δ18O of Atmospheric O2 from Air Inclusions in the Vostok Ice Core: Timing of CO2 and Ice Volume Changes during the Penultimate Deglaciation. Paleoceanography 6 (6), 679–696. doi:10.1029/91pa02023
Spratt, R. M., and Lisiecki, L. E. (2016). A Late Pleistocene Sea Level Stack. Clim. Past 12, 1079–1092. doi:10.5194/cp-12-1079-2016
Steffen, W., Rockström, J., Richardson, K., Lenton, T. M., and Folke, D., (2018). Trajectories of the Earth System in the Anthropocene. Proc. Natl. Acad. Sci. USA 115 (33), 8252–8259. doi:10.1073/pnas.1810141115
Stephens, B. B., and Keeling, R. F. (2000). The Influence of Antarctic Sea Ice on Glacial-Interglacial CO2 Variations. Nature 404 (6774), 171–174. doi:10.1038/35004556
Stocker, T. F., and Johnsen, S. J. (2003). A Minimum Thermodynamic Model for the Bipolar Seesaw. Paleoceanography 18 (4), 1087. doi:10.1029/2003pa000920
Stott, L., Timmermann, A., and Thunell, R. (2007). Southern Hemisphere and Deep-Sea Warming Led Deglacial Atmospheric CO2 Rise and Tropical Warming. Science 318, 435–438. doi:10.1126/science.1143791
Tachikawa, K., Vidal, L., Sonzogni, C., and Bard, E. (2009). Glacial/interglacial Sea Surface Temperature Changes in the Southwest Pacific Ocean over the Past 360ka. Quat. Sci. Rev. 28, 1160–1170. doi:10.1016/j.quascirev.2008.12.013
Talley, L. (2013). Closure of the Global Overturning Circulation through the Indian, Pacific, and Southern Oceans: Schematics and Transports. oceanog 26 (1), 80–97. doi:10.5670/oceanog.2013.07
Thiagarajan, N., Subhas, A. V., Southon, J. R., Eiler, J. M., and Adkins, J. F. (2014). Abrupt Pre-bølling-allerød Warming and Circulation Changes in the Deep Ocean. Nature 511, 75–78. doi:10.1038/nature13472
Toggweiler, J. R., Russell, J. L., and Carson, S. R. (2006). Midlatitude Westerlies, Atmospheric CO2, and Climate Change during the Ice Ages. Paleoceanography 21, PA2005. doi:10.1029/2005pa001154
Visser, K., Thunell, R., and Stott, L. (2003). Magnitude and Timing of Temperature Change in the Indo-Pacific Warm Pool during Deglaciation. Nature 421, 152–155. doi:10.1038/nature01297
WAIS Divide Project Members (2013). Onset of Deglacial Warming in West Antarctica Driven by Local Orbital Forcing. Nature 500, 440–444. doi:10.1038/nature12376
WAIS Divide Project Members (2015). Precise Interpolar Phasing of Abrupt Climate Change during the Last Ice Age. Nature 520, 661–665. doi:10.1038/nature14401
Wang, P. (2021). Low-latitude Forcing: A New Insight into Paleo-Climate Changes. The Innovation 2 (3), 100145. doi:10.1016/j.xinn.2021.100145
Watson, A. J., Vallis, G. K., and Nikurashin, M. (2015). Southern Ocean Buoyancy Forcing of Ocean Ventilation and Glacial Atmospheric CO2. Nat. Geosci 8, 861–864. doi:10.1038/ngeo2538
Weaver, A. J., Saenko, O. A., Clark, P. U., and Mitrovica, J. X. (2003). Meltwater Pulse 1A from Antarctica as a Trigger of the Bolling-Allerod Warm Interval. Science 299, 1709–1713. doi:10.1126/science.1081002
Keywords: milankovitch theory, ice age cycles, forcing mechanisms, bimodal hypothesis, termination from the south, cooling from the north
Citation: Lai Z, Xu Y and Zheng P (2021) Earth Ice Age Dynamics: A Bimodal Forcing Hypothesis. Front. Earth Sci. 9:736895. doi: 10.3389/feart.2021.736895
Received: 06 July 2021; Accepted: 24 August 2021;
Published: 08 September 2021.
Edited by:
Shiyong Yu, Jiangsu Normal University, ChinaCopyright © 2021 Lai, Xu and Zheng. This is an open-access article distributed under the terms of the Creative Commons Attribution License (CC BY). The use, distribution or reproduction in other forums is permitted, provided the original author(s) and the copyright owner(s) are credited and that the original publication in this journal is cited, in accordance with accepted academic practice. No use, distribution or reproduction is permitted which does not comply with these terms.
*Correspondence: Zhongping Lai, emhvbmdwaW5nLmxhaUB5YWhvby5jb20=